ABSTRACT
Growing global demand for deep-sea resources may lead to increased pressure on benthic ecosystems. Here we examined changes in meiofaunal communities following an in situ physical disturbance experiment. A significant change in meiofaunal community structure in surface (0-1 cm) and subsurface (1-5 cm) sediments was observed immediately following the disturbance at both the directly and indirectly disturbed sites and adjacent potentially undisturbed/indirectly disturbed sites, reflecting a decrease in the abundance of several meiofaunal taxa. Abundance, taxon richness and diversity also decreased immediately following disturbance across all sites. Surface community parameters returned to pre-disturbance values one year post-disturbance, but only a partial recovery was observed for the subsurface community over the same period. The accumulation of recently resuspended and deposited sediment could have led to lower meiofaunal density and shifts in community structure. Passive dispersal and recolonisation from outside the study area may explain why the surface community recovered more fully than the subsurface community. We show that meiofauna were negatively affected by a relatively minor disturbance compared to proposed commercial seabed mining operations, but the finding that communities had largely recovered one year after disturbance has implications for resilience of fauna to much more pronounced impacts of potential large-scale mining.
Introduction
Deep-sea (depths > 200 m) soft sediment environments constitute the largest habitat on Earth characterised by highly diverse communities that play an important role in providing valuable ecosystem services (Smith et al. Citation2009; Thurber et al. Citation2014; Snelgrove et al. Citation2018). However, these communities are particularly vulnerable to anthropogenic disturbance due to the generally low productivity of deep-sea environments and the naturally low levels of disturbance they have experienced during their evolution (Ramirez-Llodra et al. Citation2010). Increasing human pressures to expand the exploitation of deep-sea resources has led to a fishing footprint extending to deeper areas further offshore (Bensch et al. Citation2008). Global demand for raw elemental and mineral materials to support emerging ‘green technologies’ (Hein et al. Citation2013) will likely see seabed mining operations developing in the near future, although there are concerns about the impact of such human activities (Gollner et al. Citation2017; Niner et al. Citation2018; Clark et al. Citation2020; Orcutt et al. Citation2020).
A number of simulated mining experiments were conducted between 1970–1995 on the abyssal plains of the eastern Pacific Ocean in an effort to understand the impacts of polymetallic nodule mining on soft-sediment benthic communities (reviewed by Jones et al. Citation2017). These experiments revealed the likely impacts of mining gear on seabed habitats and their communities, including direct faunal mortality, habitat removal and habitat modification (Ingole et al. Citation2000; Ahnert and Schriever Citation2001; Radziejewska Citation2002; Miljutin et al. Citation2011). Mining impacts all benthic organisms, including microbes, meio-, macro- and megafauna, and the ecosystem functions they support, which may not completely recover even decades following the initial seabed disturbance (Miljutin et al. Citation2011; Jones et al. Citation2017; Simon-Lledó et al. Citation2019; Vonnahme et al. Citation2020). Similarly, research on the impacts of bottom trawling disturbance on deep-sea soft sediment-ecosystems provides evidence for pronounced and persistent changes to the physical and biogeochemical characteristics of the sediments and faunal communities, including loss of labile organic matter and fine sediment particles due to erosion and sediment mixing with concomitant reduction in infaunal abundance and diversity (Pusceddu et al. Citation2014; Thrush et al. Citation2016; Paradis et al. Citation2021). In shelf environments, the effects of trawling on seafloor biogeochemistry and faunal communities have been shown to differ between fine, cohesive sediments compared to coarser and non-cohesive sediments, although patterns of impact vary (Sciberras et al. Citation2016; Hale et al. Citation2017). Studies conducted in shallow environments show potentially weaker impacts of trawling on infauna relative to epifaunal organisms (e.g. Collie et al. Citation2000, Tiano et al. Citation2020), which may lead to disturbed seafloor communities becoming more strongly dominated by smaller-bodied infauna relative to undisturbed communities (Kaiser et al. Citation2001).
While the direct impacts for benthic communities from the physical impact of the seabed by potential seabed mining and from bottom trawling are relatively well understood, the impact from the resuspension and deposition of sediment (hereafter referred to collectively as sedimentation) resulting from the operation of mining equipment and trawls is less well studied (Sharma et al. Citation2001; Clark et al. Citation2016; Christiansen et al. Citation2019). The effects of sedimentation on individual organisms are wide-ranging and occur along a continuum from potentially beneficial (Rosenfeld et al. Citation1999; Anthony Citation2000) to causing mortality (Flores et al. Citation2012; Hendrick et al. Citation2016). Sedimentation can reduce the function of individual organisms (Pinheiro et al. Citation2021), and at the wider community level, ecosystem functioning can be impaired (Mevenkamp et al. Citation2017) and biodiversity reduced (Thrush et al. Citation2016). However, these sedimentation effects are known largely from studies of shallow-water benthic communities and laboratory experiments on shallow-water taxa. It is only comparatively recently that a greater focus has been given to assessing the responses of deep-sea communities and taxa to the effects of sedimentation (e.g. Mobilia et al. Citation2021). These studies are necessary to define what constitutes ‘significant adverse impacts’ in the context of fishing (FAO Citation2009) or ‘serious harm’ to an ecosystem or community in the context of deep-sea mining, which is a critical step in determining conditions under which mining companies may or may not operate in the future (Levin et al. Citation2016).
In Aotearoa/New Zealand, there has been interest in mining phosphorite nodules on the Chatham Rise since their economic value and their potential use as a locally-sourced agricultural fertiliser was first recognised (Cullen Citation1975; Mackay et al. Citation1980). However, little was known about benthic communities in this area at the time that the prospect of mining was originally proposed (Dawson Citation1984; Kudrass and Von Rad Citation1984a) and subsequent mining-related studies have also been limited to assessing baseline conditions (Rowden et al. Citation2014; Leduc et al. Citation2015). Phosphorite deposits vary from grains of 1 mm to nodules from 2 to 150 mm (Cullen Citation1980), and on the Chatham Rise, a range of benthic taxa have been found in association with these deposits. These communities include a potentially specialised infauna dominated by amphipods (Leduc et al. Citation2015), while larger phosphorite nodules can sit exposed on the seabed surface and be colonised by animals such as corals and sponges (Dawson Citation1984; Kudrass and Von Rad Citation1984a). Mining of phosphorite nodules is expected to remove large amounts of seabed and generate associated sediment plumes (Lescinski et al. Citation2014). Therefore, the benthic communities and taxa of phosphorite deposit habitats are expected to be particularly vulnerable to the impacts of sedimentation from mining activities.
In 2013, a Mineral Prospecting Licence was issued to Chatham Rock Phosphate (CRP) by the New Zealand government which allowed CRP to conduct sampling to evaluate the economic value of phosphorite deposits within their licence area on the rise. In order to begin mining, companies must first obtain a ‘marine consent’ from the Environmental Protection Authority (EPA) in accordance with the Exclusive Economic Zone and Continental Shelf (Environmental Effects) Act 2012. An environmental impact assessment (EIA) is required to gain this consent (among other requirements) and was provided by CRP based on numerous field and desktop studies (Chatham-Rock-Phosphate Citation2014). The consent to mine sought by CRP was refused in 2015 in part because the EIA did not present sufficient quantitative information on the expected impact of the proposed mining on benthic communities in the area, including the likely effects of sedimentation that would result from CRP’s proposed mining plan (EPA Citation2015).
In 2016, the National Institute of Water and Atmospheric Research (NIWA) initiated a project (‘Resilience of deep-sea benthic communities to the effects of sedimentation’; ROBES) to assess the response of benthic communities on the Chatham Rise to sedimentation from a physical seabed disturbance designed to stimulate a sediment plume that could mimic the features arising from types of sea-floor mining and bottom trawling, which may negatively impact benthic communities elsewhere (Hinchen et al. Citation2021).
The present study uses samples from ROBES to assess the response of meiofauna to physical disturbance and sedimentation. Meiofauna are an important component of benthic ecosystems, with studies in coastal and shelf environments showing that meiofauna grazing and micro-bioturbation have a positive effect on sediment processes including carbon mineralisation and nutrient cycling (Alkemade et al. Citation1992; Rysgaard et al. Citation2000; Nascimento et al. Citation2012; Bonaglia et al. Citation2014; Schratzberger and Ingels Citation2018). In the deep sea, the diversity of nematodes (the most abundant meiofaunal taxon) is positively correlated with ecosystem function, suggesting that they may influence ecosystem processes beyond shallow water ecosystems (Danovaro et al. Citation2008; but see also Leduc et al. Citation2013). However relatively little is known about the impact of seabed disturbance on meiofauna communities, with the majority of studies so far focusing on mega- and macrofauna. The evidence available to date suggests that disturbance from trawling may have a positive (Pranovi et al. Citation2000), negative (Schratzberger and Jennings Citation2002; Hinz et al. Citation2008; Pusceddu et al. Citation2014), or only minor impact on meiofauna community attributes (Schratzberger et al. Citation2002; Lampadariou et al. Citation2005; Hasemann et al. Citation2020). In the deep sea, experiments conducted on the impacts of nodule mining on abyssal nematode communities in the Clarion-Clipperton Fracture Zone (Tropical and Equatorial Pacific) showed a negative impact on nematode abundance and diversity with impacts still detectable 26 years following the initial disturbance (Miljutin et al. Citation2011). This lack of recovery may be related to the slow currents characteristic of abyssal ecosystems, low productivity resulting in slow reproductive rates and population growth, and/or the lack of opportunistic species able to quickly recolonise disturbed areas.
The aims of the present study are to assess how meiofaunal community structure is impacted by physical disturbance and sedimentation, whether impacted communities show signs of recovery within short to medium timeframes (less than one week to one year), and to investigate how the observed meiofaunal patterns may be explained by a suite of sediment variables. We hypothesised that: (1) seabed disturbance and sedimentation will lead to a significant short-term shift in meiofaunal community parameters at directly and indirectly disturbed sites (i.e. subject to both direct physical disturbance effects and indirect sedimentation effects) relative to adjacent undisturbed or indirectly disturbed sites (i.e. potentially subject to indirect sedimentation effects), followed by partial to full recovery (i.e. a statistically significant treatment x time interaction in analyses of multivariate community data), and (2) disturbance effects on the meiofauna community will be most pronounced in surface, rather than in subsurface sediments because surface communities are directly exposed to both the mechanical nature of the physical disturbance and the subsequent sedimentation.
Methods
Survey area and design
The Chatham Rise is a submarine topographic feature that extends roughly 1000 km east of Aotearoa/New Zealand. The crest of the rise is 300–400 m deep and slopes away steeply northwards to the Hikurangi Plateau and more gently southwards to the Bounty Trough to depths of more than 3000 m (Mackay et al. Citation2005) (). The topography of the crest, where phosphorite deposits are mainly concentrated, is flat-lying though highly irregular (Nodder et al. Citation2012) and contains sediment-filled hummocks and swales (Kudrass and Von Rad Citation1984b), pockmarks, and iceberg scour marks (Stewart et al. Citation2016). Sediments covering the crest region are predominantly organic-rich, glauconitic muddy sands (Nelson et al. Citation2022), with terrigenous sediments more common in western areas (McDougall Citation1982). Surficial or near-surface nodules occur in a layer of sediment that ranges in thickness from a few centimetres to 70 cm deep (20-30 cm average) (Cullen Citation1987).
Figure 1. Map of (A) Aotearoa/New Zealand (inset) showing location of Chatham Rise, study area (black filled rectangle), Mid Chatham Rise Benthic Protection Area (BPA) and Chatham Rock Phosphate’s Mineral License and Mineral Permit areas (numbered), and (B) study area showing location of reference site (black X) and Butterknife feature (black rectangle), with multibeam echosounder backscatter background showing areas of high and low reflectivity (white and dark grey shading, respectively).
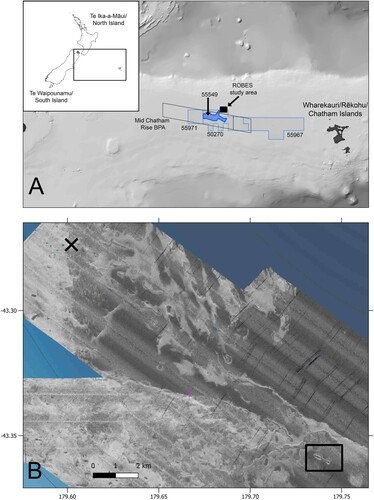
Most of the crest region contains a structurally and functionally diverse infaunal community dominated by meiofaunal nematodes (Grove et al. Citation2006). Meiofaunal density and biomass are highest on the crest and the upper southern slope and decrease with increasing water depth either side of the rise; however, this decrease is more pronounced on the northern slope (Nodder et al. Citation2003; Grove et al. Citation2006). This relationship is likely explained by strong correlations between infaunal biomass and sediment chlorophyll a concentration (often decreasing with increasing water depth), revealing a close link between benthic biomass and food supply on the Chatham Rise (Berkenbusch et al. Citation2011; Pilditch et al. Citation2015). This pattern reflects the different levels of primary productivity and flux of organic matter from the euphotic zone to the seabed across the Rise (Nodder and Northcote Citation2001; Nodder et al. Citation2007).
The ROBES survey area was located on the northern edge of the crest of the Chatham Rise at depths of ca. 450 m ( & 2). The area consists of a relatively uniform, flat-lying sediment slope with patches of exposed bedrock and cobbles where previous seabed imagery and benthic samples indicated that coral communities could be present (Bowden et al. Citation2017; Clark et al. Citation2018). The area was selected based on the following criteria: Substrate – an area with nodule/sediment combination realistic as a substrate for potential mining (and trawling); Biology – an area where the faunal composition is representative of CRP’s mining license area (and trawling areas) (e.g. presence of the coral Goniocorella dumosa), with densities high enough to measure meaningful changes following sedimentation, but not too high as to be considered a sensitive or vulnerable habitat; Trawling distribution – an area with low trawling effort to date (Baird and Mules Citation2021) and likely to be untrawled in the future; and, Away from protected areas – an area several kilometres outside of the Mid Chatham Rise Benthic Protection Area ((A)).
A localised seabed disturbance was conducted in June 2019 adjacent to an elongated seafloor depression called the ‘Butterknife’ (). Backscatter data from an acoustic survey using a shipboard multibeam echosounder (MBES) and towed camera footage identified that the butterknife-shaped feature consisted of a hard substrate rim of exposed bedrock and cobbles with dense coral communities and soft substrates inside and on either side of the rim (Clark et al. Citation2019).
Figure 2. Butterknife feature showing location of directly disturbed sites (DI; grey filled circles), undisturbed or indirectly disturbed sites (UI; black diamonds), trajectory of Sediment Cloud Inducing Plow (SCIP) when in contact on the seabed (light blue) and location of the three landers (white crosses; L1-L3). Multibeam echosounder backscatter background shows areas of high and low reflectivity (white and dark grey shading, respectively).
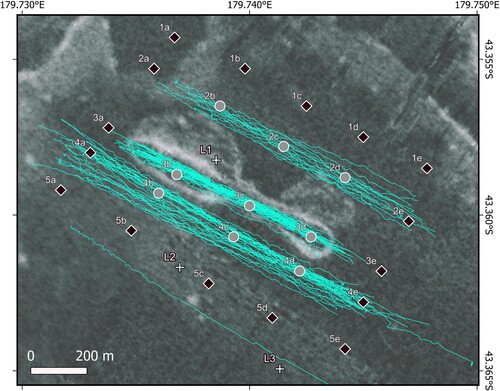
Pre- and immediate post-disturbance sampling events were conducted in 2019 followed by a post-disturbance monitoring survey one year later in 2020, allowing the resilience and potential recovery of benthic communities to be assessed in the short to medium term (i.e. week to year). An agricultural plough (termed ‘Sediment Cloud Induction Plough’ (SCIP)) (Clark et al. Citation2019), similar to that used for the DISCOL disturbance (Thiel and Schriever Citation1990), was modified to control the depth of seabed penetration and to direct resuspended sediment vertically during the disturbance (). The weight of SCIP, including the attached harrow mat, was 800 kg and the frame measured 2.5 × 3.4 × 0.8 m (length x width x height). A tickler chain was attached to the front of SCIP, which initiated the sediment disturbance. Behind the chain, twenty-eight tynes, or ‘teeth’ (total length 67 cm), were attached to the SCIP frame in three rows. These tynes extended 15 cm below the frame to stir up deeper sediments. A harrow mat (weighing 180 kg) was attached at the rear of the frame with the purpose of scraping the top of the sediment and increase sedimentation with smaller ‘teeth’ at a higher density than the more deeply penetrating tynes. SCIP was towed at 1.5-2.0 knots, and its position was monitored by fitting an ultra-short baseline transducer.
Figure 3. The Sediment Cloud Induction Plough (SCIP) used during the Butterknife disturbance experiment, showing (a) tickler chain, (b) tynes, and (c) harrow mat.
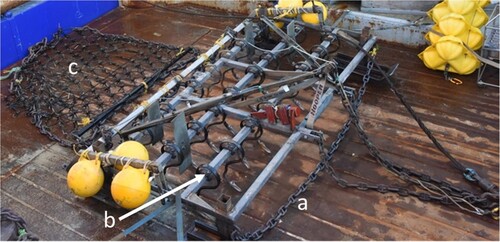
There were three disturbance transects around the Butterknife: (1) to the south of the southern rim, (2) ‘inside’ the rim with shorter tows to land and haul clear of the east and west rims, and (3) to the north of the northern rim. Multiple SCIP tows were conducted along each transect to more accurately simulate seafloor mining by attempting to maximise sediment disturbance ().
A 5 × 5 multicorer sampling grid was designed to cover the Butterknife area to span the range of disturbed and potentially undisturbed conditions (), with core sampling taking place pre-disturbance on 16–17 June 2019 (voyage TAN1903), immediately post-disturbance on 24–25 June 2019 (TAN1903) (Clark et al. Citation2019) and one year post-disturbance on 14–19 June 2020 (TAN2005) (Clark et al. Citation2021) (). Based on the location of the SCIP disturbance transects, multicore sampling points were allocated to one of two treatments: directly and indirectly disturbed (DI; subject to both direct effect of physical disturbance and indirect effect of sedimentation) or undisturbed/indirectly disturbed (UI; undisturbed or subject to indirect effect of sedimentation). Nine sites located in the centre of the grid were allocated to the DI treatment while the surrounding 16 sites were allocated to the UI treatment. Three of these UI sites (2e, 4a and 4e) were not strictly unimpacted by the SCIP but were included in the UI treatment because the number of tow lines was much less than the central DI sites.
Table 1. Details of study sites on the Chatham Rise Butterknife feature during the ROBES voyages TAN1903 (Pre- and Post-disturbance) and TAN2005 (One year post-disturbance). DI = directly and indirectly disturbed; UI = undisturbed or indirectly disturbed; x = collected sample; ns = no sample.
Due to time and technical constraints, not all of the sites were sampled at each time period, resulting in varying numbers of samples within the DI and UI areas at different sampling times. A reference site located ∼15 km to the north-west of the Butterknife disturbance area was also sampled for comparative purposes in 2019 and 2020.
An Oceans Instruments MC-800 multicorer was used to collect seabed core samples. Six core tubes (9.52 cm internal diameter) were loaded onto the multicorer frame, and one deployment was conducted at each target site during each sampling event. One core was used for meiofaunal analyses, and another from the same deployment was used for sediment analyses. The reference site was sampled with three deployments of the multicorer conducted pre-disturbance and one year after disturbance, with one core from each deployment used for meiofaunal analyses, and another for sediment analyses.
Sample treatment and analysis
Meiofauna samples were obtained using a 29 mm diameter subcore pushed to a depth of 5 cm into the sediments. One subcore was obtained from each of the cores obtained for meiofaunal analyses. Each subcore was split into 0–1 and 1–5 cm sediment layers, and each layer was fixed in 10% buffered formalin. In the laboratory, sediments were sieved using a 45 μm mesh, and the sieve contents were transferred to freshwater with Rose Bengal stain and left overnight. The meiofauna were then extracted using the Ludox method (Somerfield and Warwick Citation1996). Meiofauna were counted under a stereomicroscope (6-60× magnification) and major taxa (e.g. nematodes, harpacticoid copepods) identified.
A range of physical and biogeochemical parameters were measured for each sediment depth layer. The following physical parameters were determined: mean grain size, proportions of sand and silt/clay (mud) particles, particle skewness, kurtosis, sorting, and water content. Biogeochemical parameters were also determined, including proportions of particulate organic carbon (%POC) and particulate nitrogen (%PN), as measures of food availability, as well as their molar ratio (C:N) as a measure of food quality. Total organic matter (%TOM) was also determined as an additional measure of food availability, chlorophyll a (Chl a; μg/g of dry weight sediment) as a measure of ‘fresh’ phytodetrital organic matter, phaeopigments (Phaeo; μg/g of dry weight sediment) as a measure of degraded phytodetrital organic matter, and their ratio (Chl a:Phaeo) as a measure of the relative ‘freshness’ of the phytodetrital organic matter, with higher values being more labile. Physical sediment parameters (except %H2O and porosity) were determined by laser diffraction using a Beckman Coulter particle sizer, while %H2O and %TOM were determined by drying and loss-on-ignition (4 hours at 500°C) (Eleftheriou and Moore Citation2005). Porosity, a measure of the void spaces in the sediment, was calculated by dividing the volume of fluid (cm3) (Vw) by the total volume of wet sediment (cm3) (Vt) in each core sample. Chlorophyll a and phaeopigments were determined by standard spectrophotometric techniques subsequent to freeze-drying and extraction in 90% acetone (Sartory Citation1982), and %POC and %PN were measured using a CHN analyser (CE Instruments NC2500) with an estimated machine precision of 2%.
Bacterial sampling was also conducted with surface scrape samples obtained from the same cores as the meiofauna subcores. Sediment for bacterial analyses was obtained using a sterilised stainless-steel spoon, placed in 50 ml Falcon tubes and frozen at – 80°C before being transported to the laboratory for analysis. Flow cytometry was used to measure bacterial cell abundance (cells/cm3) following the methods of Morono et al. (Citation2009, Citation2013).
Three benthic landers were deployed for the duration of the Butterknife disturbance and carried a range of instrumentation to describe the environmental and sedimentary conditions during the experiment (i.e. currents, turbidity, temperature, salinity, dissolved oxygen, sediment fluxes) (see Clark et al. Citation2019). The landers were deployed on 15 June 2019. Lander 1 was positioned to the north of the northern Butterknife rim within 180 m of the disturbed area; Landers 2 and 3 were positioned to the south and southeast of the southern Butterknife rim at distances of 180 and 370 m to the nearest disturbed area, respectively (). After the disturbance, the landers were retrieved on 25 June 2019 for analysis of the samples and data they collected, such as total mass and POC fluxes and grain-size analyses.
Data analyses
Experimental designs in which samples are taken at two or more time periods at the same point are considered to be ‘repeated measure’ designs. These designs account for samples that are correlated through time and thus lack independence. The multicore sampling grid at the Butterknife feature was sampled on three separate occasions; however, based on the coordinate positions from the HIPAP acoustic positioning system attached to the multicorer, we calculate that the multicorer was typically several 10s of metres away (range: 0–80 m) from where the initial sample was taken at any particular site. The position of the multicorer was also likely affected by ocean currents which influence the lateral movement of the instrument as it is lowered about 450 m to the seabed. Given that the meiofauna subcores were 29 mm in diameter, and that the composition of deep-sea meiofaunal communities can vary at the centimetre scale (Gallucci et al. Citation2009), the core samples can be treated as independent and we therefore used a more conservative two-way design with crossed factors.
Data analyses were conducted using statistical routines in PRIMER v.7 (Clarke and Gorley Citation2015) with the PERMANOVA add-on (Anderson et al. Citation2008). PERMANOVA was used for analyses of univariate (abundance, taxon richness, Shannon diversity H’10 and Pielou’s evenness J’) and multivariate data as it does not rely on normally distributed data or balanced designs (Anderson et al. Citation2008). A similar suite of analyses was conducted on both sediment and meiofauna data to investigate potential disturbance effects in space and time.
Multivariate sediment data from the 0–1 and 1–5 cm layers were analysed separately to compare variability in the surface versus subsurface sediment layers. Prior to analyses, sediment data were normalised and resemblance matrices were built using the Euclidean similarity measure (Anderson et al. Citation2008). P-values were obtained by 9999 permutations of the residuals under a reduced model. We used the fixed factors Treatment (two levels; DI and UI) and Sampling period (three levels; pre-disturbance, post-disturbance, and one-year post-disturbance) to analyse patterns in sediment parameters at the Butterknife sites. We also compared sediment characteristics at the Reference site to investigate any differences between: (1) pre-disturbance and one-year post-disturbance, and (2) the Reference and Butterknife sites prior to disturbance. These analyses allowed us to determine any background temporal variability in sediment characteristics. Where PERMANOVA tests yielded significant results, pairwise PERMANOVA comparisons were conducted to identify which pair(s) of treatment levels were responsible for the significant factor effect. The SIMPER routine was used to identify which sediment parameters were responsible for any significant differences.
Analyses of meiofauna data were conducted using the same PERMANOVA design as for the sediment data, followed by pairwise comparisons and SIMPER. Multivariate meiofauna data were square root transformed to reduce the influence of the numerically abundant nematodes, and similarity matrices were built using the Bray–Curtis similarity measure. Preliminary analyses showed similar results using fourth-root transformation of multivariate meiofauna data. Bray–Curtis resemblance matrices were also used to construct non-metric multi-dimensional scaling plots (nMDS plots) using centroids to visualise the relative dissimilarities between samples and treatment groups. The SIMPER routine was used to identify the contribution of each taxon to the dissimilarities between groups. Characterising taxa are those that contribute the most to the dissimilarity between groups (>10% contribution), while discriminating taxa are those that contribute relatively consistently to those differences (dissimilarity/SD ratio >1.3) (Clarke and Warwick Citation2001).
Relationships between predictor variables from the sediment environment and meiofaunal community structure at the Butterknife sites were investigated using distance-based linear models (DistLMs) in PRIMER (Anderson et al. Citation2008). Separate analyses were conducted for the 0–1 and 1–5 cm layers. Predictor variables were checked for multicollinearity, which led to the removal of skewness and kurtosis from the list of predictor variables as they were highly correlated with each other and both were also highly correlated to silt/clay content (r ≥ 0.9; Anderson et al. Citation2008).
DistLMs were conducted where the PERMANOVA results detected significant changes in community structure, which resulted in four DistLM analyses being conducted: two analyses for pre – and post-disturbance data focusing on potential immediate disturbance impacts (0-1 and 1–5 cm were considered separately), and two analyses for the post-disturbance and one year post-disturbance data focusing on potential recovery (0-1 and 1–5 cm separately). The DI and UI sites were pooled together for these analyses as PERMANOVA results indicated no significant difference between treatments for either sediment layer (P > 0.05). As for the PERMANOVA analyses, multivariate abundance data were square root-transformed to reduce the influence of dominant taxa, and the resemblance matrices were built using Bray–Curtis similarity. Relationships between environmental predictor variables and meiofaunal community structure were first investigated by marginal tests, and subsequently by sequential tests, using the stepwise selection procedure with 9999 permutations, to determine which combination of factors best explained variation in community structure. For both marginal and sequential tests, only variables significantly correlated (P < 0.05) with meiofaunal community structure are presented.
Results
Sediments
Detailed results from the deployment of the benthic landers will be reported elsewhere (Nodder et al. Citationin prep.), so only a summary is presented here to provide sufficient environmental context for this meiofauna study. The turbidity sensors mounted at <1 m above the seabed on the benthic landers deployed around the Butterknife recorded increased turbidity in the water column during the time of coring operations and the main SCIP disturbance and confirmed that the main disturbance did create a sediment plume resulting from the resuspension of seafloor sediment ().
Figure 4. Turbidity measurements from three benthic landers positioned around the Butterknife. Benthic landers 1, 2 and 3 are labelled L1, L2 and L3, respectively. Lander 1 was positioned closest to the Butterknife and Lander 3 was furthest (see ). Red labels indicate the time at which sampling and disturbance events took place. FTU = Formazin Turbidity Unit (Nodder et al. Citationin prep; see also Clark et al. Citation2019).
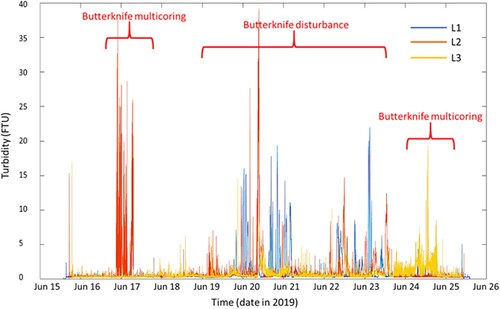
Detailed results from the sediment sample analyses will be reported in Nodder et al. (Citationin prep) but are repeated in part because these sediment variables were included in the analysis of the meiofauna data. Surficial sediment at the Butterknife sites consisted of poorly sorted sandy mud or muddy sand, mostly comprised of very fine sand and silt particles (<125 µm-diameter) (Supplement 1). On average, the 0–1 cm layer was characterised by greater water content than the 1–5 cm layer (48% versus 42%, respectively), as well as higher porosity (70% versus 65%), phaeopigment concentrations (5.0 versus 4.0 μg g-1 dry weight), organic carbon content (0.62% versus 0.55%) and mean particle diameter (70 versus 67 μm).
Sediment characteristics at the Reference site did not differ significantly between pre-disturbance and one year post-disturbance in either sediment layer (P > 0.05). Sediment characteristics were broadly similar between the Reference and Butterknife sites prior to disturbance, although there were differences (PERMANOVA, P < 0.05) in sediment granulometry characteristics, i.e. coarser mean grain size at the Reference site relative to Butterknife sites (91-104 versus 67-70 μm, respectively), higher particle sorting (75-87 versus 59-62), and lower silt/clay content (41-45% versus 56-57%) and porosity (66-72% versus 55-61%).
Analyses of multivariate sediment data at the Butterknife sites show a significant effect of sampling time for both 0–1 cm and 1–5 cm layers (PERMANOVA, P < 0.05), but no significant disturbance effect for either sediment layer (). There was a significant interaction effect for the 0–1 cm layer but not the 1–5 cm layer. Pairwise comparisons showed that sediment characteristics in the 0–1 cm layer differed significantly between all sampling times at the DI sites, whilst at the UI sites significant differences were only observed between pre-disturbance and one year post-disturbance and between immediately post-disturbance and one year post-disturbance. SIMPER analyses indicate that the dissimilarity in sediment characteristics between pre – and post-disturbance were mainly due to an increase in C:N ratio, indicating lower food quality as a result of lower nitrogen content and higher carbon content post-disturbance (, ). Variability in C:N ratios also increased markedly between pre – to post-disturbance. There was a decrease in food availability as indicated by lower total organic matter content post-disturbance, a pattern which tended to be more pronounced at the DI sites than at the UI sites. One year later, C:N ratio and total organic matter content had returned to pre-disturbance conditions. Differences in sediment physical characteristics between pre-disturbance and one year post-disturbance were related to decreased silt/clay content and increased mean grain size one year post-disturbance, as well as slightly greater total organic matter content. Bacteria cell concentrations from surface scrape samples increased gradually between pre-, post – and one year post disturbance at both disturbed and undisturbed sites (Supplement 1).
Figure 5. Sediment characteristics (C:N ratio, chlorophyll a (Chl a), total organic matter (TOM), mud content (%silt/clay) and water content (%H2O)) at directly and indirectly disturbed (DI; grey bars), undisturbed or indirectly disturbed (UI; black bars) and reference sites (white bars) prior to disturbance, immediately post-disturbance, and one year post-disturbance (mean and standard deviation). Data for the 0–1 cm layer are shown on the left-hand side graphs and corresponding data for the 1–5 cm layer on the right-hand side graphs. White bars with dotted outline show values that were measured pre-disturbance at the reference site but shown next to post-disturbance bars for ease of comparison.
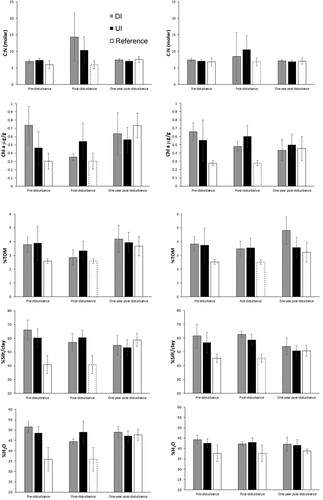
Table 2. PERMANOVA results showing significance testing for the effect of factors Time (Pre-disturbance, post-disturbance, and one year post-disturbance) and Disturbance (directly and indirectly disturbed (DI) versus undisturbed or indirectly disturbed (UI)) and their interaction on multivariate sediment parameters. Separate analyses were conducted for the 0–1 and 1–5 cm sediment layers as well as the two layers combined (0-5 cm).
Table 3. Results of SIMPER analyses showing the environmental factors responsible for differences among sampling times, i.e. pre- versus post-disturbance, pre – versus one year post-disturbance and post – versus one year post-disturbance, for the 0–1 and 1–5 cm sediment layers. % Cum. Contrib. = cumulative percentage contribution to dissimilarity in sediment characteristics. C:N = molar carbon to nitrogen ratio in sediment organic matter (i.e. molar POC:PN); TOM = %total organic matter; PN = %sediment particulate nitrogen; POC = %sediment particulate organic carbon; Silt/clay = %silt/clay; Chl a = sediment chlorophyll a in µg/g dry sediment; Phaeo = sediment phaeopigments in µg/g dry sediment.
For the 1–5 cm layer, sediment characteristics only differed significantly between pre-disturbance and one year post-disturbance, and between post-disturbance and one year post-disturbance. SIMPER analyses indicate that the dissimilarity in sediment characteristics between immediately post-disturbance and one year post-disturbance was driven by an increase in C:N ratio (as seen in the 0–1 cm layer, but less pronounced) and phaeopigment concentrations, as well as a decrease in POC and silt/clay contents, a pattern broadly similar to that observed for the 0–1 cm layer (, ). As seen in the 0–1 cm layer, differences between pre-disturbance and one year post-disturbance were mainly due to decreases in silt/clay content (and a concomitant decrease in mean grain size) and increases in total organic matter content, as well as decreased chlorophyll a content.
Overall, food availability and quality decreased immediately post-disturbance, more markedly at the DI than UI sites, and more noticeably in the surface than subsurface layer, and returned to pre-disturbance levels (or greater e.g. TOM) one year later. Sediment granulometry showed limited change immediately post-disturbance, providing little evidence of sediment deposition or resuspension, but was slightly coarser one year later. No significant temporal change was found at the Reference site (PERMANOVA, P > 0.05), although all food availability variables tended to be higher in 2020 relative to 2019, and sediment tended to be slightly finer.
Meiofauna – general description of community and variability at reference site
A total of 79 621 meiofauna specimens belonging to 22 taxa were counted and identified down to 5 cm sediment depth (Supplement 2). Meiofauna abundance in the depth-integrated 0–5 cm sediment layer ranged from 314 to 3947 ind. 10 cm−2 (mean = 1698 ind. 10 cm−2), and taxon richness ranged from 5 to 11 taxa core−1 (mean = 8.1 taxa core−1; ). Overall, about two thirds of the meiofauna (1100 ind. 10 cm−2) was found in the 1–5 cm layer, and one third (598 ind. 10 cm−2) in the 0–1 cm layer (). Taxon richness, diversity and evenness tended to be greater in the 0–1 cm layer than in the 1–5 cm layer. Nematodes were the most dominant taxon, accounting for 92% of all specimens overall (range: 38-98%), followed by copepods (mean: 3%, range: 0.8-16%), nauplii (mean: 2%, range: 0.2-10%) and polychaetes (mean: 2%, range: 0-58%) (). All other taxa represented less than 0.5% of the total. Nematodes were less dominant in the 0–1 cm layer (mean: 81% of total meiofauna, range: 3-96%) than in the 1–5 cm layer (mean: 98%, range: 77-100%). One 0–1 cm layer sample from a DI site (5d, post-disturbance) was characterised by unusually high abundance of polychaetes (1303 ind. 10 cm−2) as well as low nematode abundance (44 ind. 10 cm−2).
Figure 6. Meiofaunal community parameters (abundance, richness, Shannon diversity H’10 and Pielou’s eveness J’) at directly and indirectly disturbed (DI; grey bars), undisturbed or indirectly disturbed (UI; black bars) and reference sites (white bars) prior to disturbance, immediately post-disturbance, and one year post-disturbance (mean and standard deviation). Data for the 0–1 cm layer are shown on the left-hand side graphs and corresponding data for the 1–5 cm layer on the right-hand side graphs. White bars with dotted outline show values that were measured pre-disturbance at the reference site but shown next to post-disturbance bars for ease of comparison.
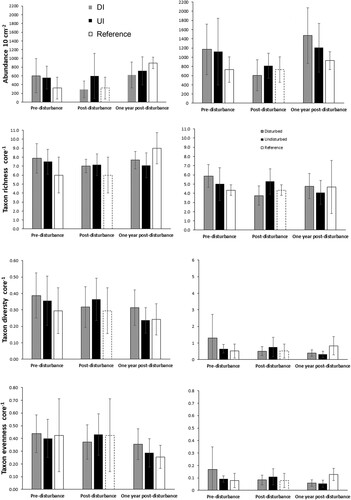
Table 4. Mean abundance (ind. 10 cm-2) of meiofauna taxa at the Reference and Butterknife sites in surface (0-1 cm) and subsurface sediment layers (1-5 cm).
Table 5. Summary of meiofaunal univariate community parameters at the disturbed, undisturbed and reference sites sampled pre-, post-, and one year post-disturbance (means, and standard deviations in brackets). Data are shown for the 0–1 cm, 1–5 cm and combined 0–5 cm sediment layers. N = abundance 10 cm-2; S = taxon richness; H′10 = Shannon diversity; J′ = taxon evenness.
There was a significant increase in mean meiofauna abundance in the 0–1 cm layer at the Reference site one year after disturbance (894 ind. 10 cm−2) relative to pre-disturbance (320 ind. 10 cm−2) (PERMANOVA, P < 0.05). Other univariate and multivariate parameters in the 0–1 cm layer did not vary significantly between sampling times. There were no significant differences for any of the univariate or multivariate community parameters in the 1–5 cm layer between pre-disturbance and one year post-disturbance at the Reference site. There was no significant pre-disturbance difference in meiofaunal community structure of either sediment layers between the Reference and Butterknife sites (P > 0.05).
Meiofauna – disturbance effects at the Butterknife sites
PERMANOVA results show a significant treatment effect on meiofauna abundance in the 0–1 cm layer, with lower meiofaunal abundance in the Butterknife DI sites compared to the UI sites (P = 0.02), but no significant time or interaction effect (P > 0.05; , ). No significant treatment, time or interaction effects were found for taxon richness, diversity or evenness in the 0–1 cm layer. There was a significant effect of sampling time on community structure in the 0–1 cm layer (P = 0.003), but no significant treatment or interaction effect (P > 0.05, ). Pairwise comparisons show that meiofaunal community structure in the 0–1 cm layer at the Butterknife sites differed significantly between pre-disturbance and one year post-disturbance, and between post-disturbance and one year post-disturbance (P < 0.05), but not between pre- and post-disturbance (P = 0.057), although the P-value was close to the threshold value. If the outlier sample with high polychaete abundance is removed, however, all pairwise comparisons become significant (P < 0.05).
Table 6. PERMANOVA results showing significance testing for the effect of factors Time (Pre-disturbance, post-disturbance, and one year post-disturbance) and Disturbance (directly and indirectly disturbed (DI) versus undisturbed or indirectly disturbed (UI)) and their interaction on meiofaunal community parameters. Separate analyses were conducted for the 0–1 and 1–5 cm sediment layers as well as the two layers combined (0-5 cm).
SIMPER analyses show that the bulk (>70%) of the variability in community structure among sampling times (combined DI and UI data) was due to changes in the abundance of nematodes, copepods, nauplii and polychaetes (). Nematodes and copepods decreased post-disturbance followed by an increase one year later, whereas nauplii decreased post-disturbance followed by a slight decrease one year after. Polychaetes showed the opposite pattern to nematodes and copepods. The dissimilarity to standard deviation ratio for polychaetes, however, was low (0.5-1.3), indicating that polychaetes do not vary in a consistent way among sampling times. When the single outlier sample at site 5d (post-disturbance) with high polychaete abundance was removed, the temporal pattern in polychaete abundance was reversed and the dissimilarity to standard deviation ratio increased to 1.3-1.4. Removal of the outlying sample also leads to the inclusion of kinorhynchs as a taxon responsible for community differences among sampling times; like the other taxa kinorhynchs show an immediate post-disturbance decrease followed by a recovery one year later.
Table 7. Results of SIMPER analyses showing the meiofaunal taxa responsible for differences in multivariate community structure at directly and indirectly disturbed (DI) and undisturbed or indirectly disturbed sites (UI) combined among sampling times, i.e. pre – versus post-disturbance, pre – versus one year post-disturbance and post – versus one year post-disturbance, for the 0–1 and 1–5 cm sediment layers. Diss/SD = dissimilarity to standard deviation ratio; Contribution % = percentage contribution to dissimilarity in community structure.
There was a significant time effect on all meiofaunal univariate parameters in the 1–5 cm layer at the Butterknife sites, but no significant treatment or interaction effect. Pairwise comparisons (combined DI and UI data) show that abundance in the 1–5 cm layer decreased significantly post-disturbance, followed by a significant increase one year later to pre-disturbance abundance levels. Taxon richness decreased significantly immediately following disturbance but showed no change one year later. Taxon diversity and evenness did not change immediately following disturbance but decreased significantly one year later. As observed in the 0–1 cm layer, there was a significant effect of sampling time on community structure in the 1–5 cm layer, but no significant treatment or interaction effect (). Community structure differed significantly between all sampling times. Most (≥75%) of the variability was due to changes in the abundance of nematodes, copepods and nauplii (SIMPER; ). All three decreased in abundance post-disturbance in the 1–5 cm layer followed by an increase one year later for nematodes, whereas copepods and nauplii showed no change or a further slight decrease one year later.
The nMDS plots of multivariate sample data and centroids summarise the patterns in meiofauna community structure (). The plots show a clear segregation at all sampling times of meiofaunal communities in the 0–1 and 1–5 cm layers, which reflects the greater overall abundance of meiofauna in the latter as well as greater taxon richness, diversity and evenness in the former. For both sediment layers, community structure shifts post-disturbance, reflecting a decrease in the abundance of the main taxa, followed by a return towards pre-disturbance community structure one year after disturbance. This shift back towards the initial community structure is most obvious for the 0–1 cm layer, where the majority of the discriminating taxa return to pre-disturbance abundance levels, and less so for the 1–5 cm layer where the densities of copepods and nauplii remain depressed relative to pre-disturbance levels.
Figure 7. Two-dimensional multidimensional scaling (nMDS) plot of square root-transformed meiofaunal taxon abundance data at combined directly and indirectly disturbed (DI) and undisturbed or indirectly disturbed (UI) sites (top) and their centroids (bottom) sampled pre-disturbance (circles), immediately post-disturbance (diamonds) and one year post-disturbance (crosses). Data are shown for the meiofaunal communities in the 0–1 cm sediment layer (grey symbols) and 1–5 cm sediment layer (black symbols) separately. See for results of PERMANOVA analysis.
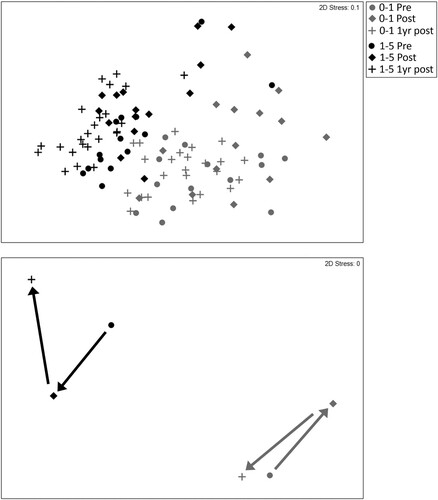
There were no statistically significant relationships between any of the environmental parameters and meiofaunal community structure in the analysis focusing on the effects of disturbance (i.e. combining pre – and post-disturbance data), in either the 0–1 or 1–5 cm sediment layers (P > 0.1). In the analyses focusing on potential recovery from disturbance (i.e. combining post – and one year post-disturbance data), community structure in the 0–1 cm layer was significantly correlated with sediment total organic matter content, sediment C:N ratio and porosity in marginal tests (). Sediment total organic matter content was retained as the only significant predictor variable in the sequential tests. Community structure in the 1–5 cm layer was significantly correlated with sediment chlorophyll a concentrations as well as phaeopigment concentrations, C:N ratio and silt/clay content in marginal tests. Chlorophyll a content was retained as the only significantly variable in sequential tests.
Table 8. Results of DistLM analyses showing correlations between environmental parameters and meiofauna community structure post-disturbance and 1 year post disturbance (combined directly and indirectly disturbed (DI) and undisturbed or indirectly disturbed sites (UI)). TOM = %total organic matter; C:N = molar carbon to nitrogen ratio in sediment organic matter (i.e. molar POC:PN); PN = %sediment particulate nitrogen; POC = %sediment particulate organic carbon; Silt/clay = %silt/clay; Chl a = sediment chlorophyll a in µg/g dry sediment; Phaeo = sediment phaeopigments in µg/g dry sediment.
Discussion
The meiofauna community at both the directly disturbed and indirectly disturbed sites (DI) and adjacent potentially undisturbed or indirectly disturbed sites (UI) were impacted by the experimental SCIP disturbance. We expected the surface meiofauna community to be more severely impacted by disturbance, however, disturbance impacts were similar for both surface and subsurface meiofauna. We observed a near complete recovery of the meiofauna community one year post-disturbance as expected, which was linked to increased food availability in the sediments over that period. However, our results suggest that meiofauna living in surface sediments may recover more quickly than meiofauna living in subsurface sediments. We discuss these results in more detail below with regards to the impact of disturbance on sediment characteristics and the Chatham Rise meiofauna community.
Effect of disturbance on sediment characteristics
The physical seabed disturbance and sedimentation induced by SCIP led to a relatively minor decrease in food quality (C:N ratio) and quantity (TOM) in the sediment immediately after disturbance. This decrease was most pronounced in the surface sediment layer (0-1 cm) at sites directly and indirectly impacted by the disturbance (DI sites), whereas limited change was observed in the 1–5 cm subsurface sediment layer at sites not directly impacted by the disturbance (UI sites). Sediment parameters in both layers returned back to pre-disturbance values one year after disturbance.
Physical disturbance of the sediments can lead to loss of organic matter via the resuspension and removal of organic-rich surface sediments and sediment mixing that increases oxygen penetration and hence the remineralisation of buried organic matter (e.g. Tiano et al. Citation2019). Fine organic material can also be winnowed leaving the remaining coarser sediment poorer in high quality, labile organic matter (Sané et al. Citation2013). In a meta-analysis of fishing disturbance impacts on sediment carbon storage, Epstein et al. (Citation2021) showed that only 29% of studies found a negative effect whilst most (61%) found no significant effect. The magnitude of any disturbance effects on sediment food quantity and quality can vary based on a variety of factors including history of disturbance from natural and anthropogenic sources, sediment granulometry, and functional characteristics of the benthic community (Sané et al. Citation2013; Hale et al. Citation2017). Fine cohesive sediments which are only infrequently disturbed (such as deep-sea sediments) are more likely to exhibit a loss of organic matter following a physical disturbance event than non-cohesive sandy sediments in areas subject to strong currents or regular anthropogenic disturbance (Epstein et al. Citation2021). Despite the relatively deep location of our Chatham Rise study sites (∼450 m-deep), sediments were moderately coarse-grained, comprising predominantly sandy muds with moderate sand content (mean = 43%) and a relatively deep oxic layer (2.5-3.0 cm; Hale et al. Citationin prep.). It seems likely that resuspension is relatively frequent in our study area (Cullen and Singleton Citation1977; Nodder and Northcote Citation2001). Images of the seafloor taken at eight sites where there were live coral clumps recorded in 2018 (see Clark et al. Citation2018) show sediment covering on average 24% of the area (author’s unpublished data). Acoustic Doppler current profiler data obtained at the Chatham Rise Reference site in 2018 indicate relatively high near-bed current speeds of up to 0.4 m s−1 (Clark et al. Citation2018), as observed at other Chatham Rise crest locations (Heath Citation1983; Chiswell Citation1994). These characteristics may help explain the weak and short-term impact of the Butterknife disturbance on sediment characteristics at our study sites.
Effect of disturbance on meiofauna
We found limited evidence to support our first hypothesis of a disturbance effect on meiofauna communities restricted to the directly and indirectly disturbed (DI) sites (i.e. our analyses showed no significant disturbance × time interaction effect on meiofaunal abundance, richness, diversity or community structure in surface or subsurface sediment layers). Instead, we observed a shift in meiofaunal community structure in surface and subsurface sediments immediately following seabed disturbance at both DI and UI sites, reflecting a decrease in the abundance of the main meiofaunal taxa (i.e. nematodes, copepods, nauplii, polychaetes and kinorhynchs). Likewise, we found no evidence to support our second hypothesis predicting that disturbance effects on meiofaunal communities would be more pronounced in surface than subsurface sediments. Instead, we found a similar shift in meiofaunal community attributes immediately after the disturbance in both sediment layers, with a more complete recovery one year after disturbance in surface sediments relative to subsurface sediments.
Our observations of a shift in meiofaunal community structure immediately after disturbance, followed by a partial recovery one year later, at both DI and UI sites suggests that the impacts of disturbance on meiofauna were not due to direct mortality, mechanical sediment mixing, or winnowing of meiofauna due to direct physical disturbance from the SCIP. Given the small physical dimensions of the cores used to sample meiofauna, it is possible that some of the samples obtained at the DI sites post-disturbance were taken outside of the immediate SCIP footprint, which could account for some of the similarity in the post-disturbance response observed at DI and UI sites (i.e. some DI cores may only have experience indirect sedimentation effect similar to the UI cores). The settlement of fine particles resuspended by the passage of the disturber could have affected meiofaunal communities at both DI and UI sites in a similar manner, and on a timeframe of several days. No direct measurements of sedimentation on the seafloor were made. However, a small decrease in sediment organic matter quantity and quality was detected in analyses of sediment characteristics, which likely resulted from the suspension and redeposition of sediment and organic matter. The SCIP video camera also showed clear suspension of sediment from the gear as confirmed by turbidity and camera data from the landers (). The effect on near-bed turbidity was typically greatest at the most proximal lander site (L1), deployed within the set of disturbed sites, and this effect diminished systematically at the less proximal sites (i.e. from L2 to L3), which were to the south of the line of undisturbed sites (5a-e) (, ). Total mass flux to the seabed, as measured by sediment traps deployed on benthic landers during the disturbance experiment, reached values almost five times greater (ca. 29 gm−2d−1) than mean background fluxes for that time of year on Chatham Rise (6 gm−2d−1), indicating significant settlement of resuspended particles at the seafloor (Nodder et al. Citationin prep.). The accumulation of recently resuspended and deposited, organic matter-rich sediment, with low food quality (heightened C:N ratios), as observed in the sediment traps deployed in the Butterknife area (Nodder et al. Citationin prep.), could have led to lower meiofaunal density in the surface 0–1 cm layer, if we assume that meiofauna did not migrate vertically in the sediment in the period immediately following disturbance. This ‘dilution’ effect could also potentially explain the decrease in meiofauna density in the 1–5 cm layer if no vertical migration took place.
Experimental studies conducted on shallow water meiofauna have shown that although community structure is impacted by the deposition of up to several cm of sediment (Schratzberger et al. Citation2000), at least part of the community (nematodes in particular) is capable of migrating up into the layer of newly deposited material (Wulff et al. Citation1997). One study conducted on a mud flat over a period of nine months even showed a positive effect of burial on nematode species richness and diversity, whilst macrofauna showed a negative response (Whomersley et al. Citation2009). The positive response of meiofauna to burial in this instance may have been related to lower competition from macrofauna and from the increased availability of food resources (Whomersley et al. Citation2009). On the other hand, in an experimental study conducted in a Norwegian fjord (200 m depth) the deposition of 3 cm of sediment led to significant nematode mortality after 16 days (Mevenkamp et al. Citation2017). In comparison, in a study conducted at 4200 m depth in the Peru Basin (Southeast Pacific), the deposition of 2 cm of crushed nodule particles led to upward migration by surface (0-2 cm) meiofauna into the newly deposited material 11 days later, whereas the subsurface meiofauna (2-5 cm) did not migrate (Mevenkamp et al. Citation2019). On the continental slope of Japan, the deposition of an estimated 1–7 cm of sediment following the 2011 Mw 9.0 Tōhoku mega-earthquake led to unusual subsurface peaks in nematode abundance one year after the event, whereas benthic copepods were always most abundant in surface sediments (Nomaki et al. Citation2015). Overall, these studies indicate that meiofauna in both shallow and deep-sea environments may, over timescales of days to weeks, respond to localised sediment deposition events by migrating up into the freshly deposited layer, but that large scale events such as earthquake-triggered turbidity flows may have longer lasting, negative impacts. However, so far, no experimental data are available about the meiofauna response immediately following burial (on timescales of hours to a few days).
Research on the effects of bottom trawling have shown a similar range of responses by meiofauna, from positive (Pranovi et al. Citation2000), negative (Schratzberger and Jennings Citation2002; Hinz et al. Citation2008; Pusceddu et al. Citation2014), to only minor (Schratzberger et al. Citation2002; Lampadariou et al. Citation2005). This variability in response may be related to disturbance site conditions such as hydrodynamic processes and food supply, with meiofaunal communities in areas that are frequently disturbed by currents and highly productive less likely to be negatively impacted by trawl disturbance than communities in areas that are infrequently disturbed and with low food supply (Hinz et al. Citation2008). Generally, the most negative and pervasive impacts on sediment meiofauna by trawling disturbance are linked to chronic disturbance leading to shifts in sediment characteristics such as grain size and food availability (Pusceddu et al. Citation2014), which are key factors influencing the structure of meiofauna communities (Giere Citation2009; Leduc et al. Citation2012). The absence of any major changes in the physical structure and biogeochemistry of disturbed and undisturbed surface sediments (0-1 cm), as observed during the ROBES experiment, and the location of the experiment in a region characterised by high productivity and export flux (Nodder and Northcote Citation2001; Nodder et al. Citation2016) and moderately strong near-bottom currents (Chiswell Citation1994), suggests that meiofaunal communities on the Chatham Rise crest are likely to have adaptations to seafloor disturbances on the scales of the ROBES experiment.
Research on seabed mining impacts on abyssal meiofaunal communities have shown a negative impact immediately following disturbance followed by partial recovery in the following years, which may be aided by increased organic matter availability stemming from the reworking of the sediment by the disturbance itself or from natural variability in surface primary production (Radziejewska et al. Citation2001; Radziejewska Citation2002; Ingole et al. Citation2005; Sharma et al. Citation2005). However, in some instances recovery may not be complete for as long as 26 years post-disturbance (Miljutin et al. Citation2011). The information available to date is limited to the direct impacts of simulated mining disturbance on meiofaunal communities (Jones et al. Citation2017), and to our knowledge, there are at present no published data on the potential indirect impact of mining on the benthos that may occur through the settling of seafloor mining-generated sediment plumes (Weaver et al. Citation2022).
On Chatham Rise, one year following disturbance, meiofaunal community parameters had largely recovered to values observed pre-disturbance. The main exception was the abundance of harpacticoid copepods and nauplii, which remained below pre-disturbance levels in the subsurface sediment layer, leading to altered community structure and lower taxon richness. This partial to near-complete recovery of surface and subsurface meiofauna communities was linked to increases in food quantity and quality over the same period (increased TOM, lower C:N ratios) as well as shifts in sediment physical characteristics (increase in silt/clay particles and porosity). Whilst an increase in food supply may have contributed to the recovery of meiofauna by promoting population growth locally (Grove et al. Citation2006; Smith et al. Citation2008), recolonisation via active and passive means may also have played a role. Passive dispersal may be particularly important in the highly dynamic environment that characterises the Chatham Rise study area, where water masses and the location of the Subtropical Front can vary annually (Collins et al. Citation2023) and current speeds can reach up to 0.4 m s−1, leading to the expectation of moderately frequent sediment resuspension and lateral transport over potentially large distances (Hadfield et al. Citation2007, Citation2011; Lescinski et al. Citation2014; Clark et al. Citation2018). Recolonisation from nearby areas would explain why the surface meiofauna community recovered more fully than the subsurface community, because taxa that are found primarily in subsurface sediment layers are less prone to resuspension and have lower potential for passive dispersal via bottom currents (Hauquier et al. Citation2017).
Implications
Meiofauna are generally considered more resilient to physical disturbance than larger infaunal and epifaunal taxa because they are less likely to be damaged due to their small size, have a faster turnover rate and reproduce throughout the year (Giere Citation2009; Moens et al. Citation2014). The results of our study on the Chatham Rise indicated that meiofauna communities are indeed generally resilient to disturbance and had largely recovered from the simulated disturbance after one year. Data obtained as part of the same disturbance experiment show a similar but slightly more pronounced effect of disturbance on macrofauna (including decreased macrofaunal abundance immediately following disturbance at the DI sites but not the UI sites), followed by an apparently complete recovery a year later (Murray et al. Citationsubmitted). Therefore, it appears that meio – and macrofauna on Chatham Rise are both relatively resilient to a short-term localised physical disturbance.
Although impacts of the disturbance by SCIP on meiofauna were relatively small and transitory, meiofauna did show a negative and short term (<1 year) response to a relatively minor seafloor disturbance, with SCIP acting more like a bottom trawl than a proposed mining operation. Passive dispersal by currents may be an important mechanism for recolonisation of disturbed patches of sediment on the Chatham Rise and in other upper bathyal environments, but we do not yet know at what spatial scales this process occurs. Our results indicate that recolonisation may take place at a scale of about one km2 in our study area, but this is a relatively small area compared to that of commercial phosphorite mining operations proposed in 2014 (100s of km2) (Chatham Rock Phosphate Citation2014) or current commercial bottom trawling on the Chatham Rise (10,000s of km2) (Baird and Mules Citation2021). Furthermore, our results indicate that some subsurface meiofaunal taxa take longer than one year to recover, which could potentially lead to long-term impacts on deep-sea ecosystem processes and services such as carbon and nutrient cycling that meiofauna are known to facilitate (Schratzberger and Ingels Citation2018).
Supplement 1 Sediment data
Download MS Excel (37.2 KB)Supplement 2 Meiofauna data
Download MS Excel (26 KB)Acknowledgments
We are grateful to the officers and crews of the RV Tangaroa ROBES voyages, as well as other members of the scientific parties, for their support and professionalism that made the sampling possible. We thank Megan Carter and Jane Halliday (both NIWA) for sorting the meiofauna samples. Karen Thompson and the team at the NIWA Hamilton Water Quality Laboratory are thanked for generating the bacterial and environmental data used in the analyses. We are grateful to Teresa Radziejewska and Vadim Mokievsky for providing constructive criticisms on the manuscript. This research was made possible by funding from the Tangaroa Reference Group (TRG) for ship time, and for the ROBES project as a whole by the Ministry for Business, Innovation and Employment (MBIE) (MBIE contract CO1X1614).
Disclosure statement
No potential conflict of interest was reported by the author(s).
Additional information
Funding
References
- Ahnert A, Schriever G. 2001. Response of abyssal Copepoda Harpacticoida (Crustacea) and other meiobenthos to an artificial disturbance and its bearing on future mining for polymetallic nodules. Deep-Sea Research II. 48:3779–3794.
- Alkemade R, Wielemaker A, Hemminga MA. 1992. Stimulation of decomposition of Spartina anglica leaves by the bacterivorous marine nematode Diplolaimelloides bruciei (Monhysteridae). Journal of Experimental Marine Biology and Ecology. 159:267–278. doi:10.1016/0022-0981(92)90041-8.
- Anderson M, Clarke K, Gorley R. 2008. Permanova+ for primer. Guide to software and statistical methods. Plymouth, UK: University of Auckland and PRIMER-E Ltd.
- Anthony KR. 2000. Enhanced particle-feeding capacity of corals on turbid reefs (Great Barrier Reef, Australia). Coral Reefs. 19:59–67. doi:10.1007/s003380050227.
- Baird SJ, Mules R. 2021. Extent of bottom contact by commercial trawling and dredging in New Zealand waters, 1989–90 to 2018–19. New Zealand Aquatic Environment and Biodiversity Report. 260:157.
- Bensch A, Gianni M, Greboval D, Sanders J, Hjort A. 2008. Worldwide review of bottom fisheries in the high seas. FAO Technical Paper. 522:1–145.
- Berkenbusch K, Probert PK, Nodder S. 2011. Comparative biomass of sediment benthos across a depth transect, Chatham Rise, Southwest Pacific Ocean. Marine Ecology Progress Series. 425:79–90. doi:10.3354/meps09014.
- Bonaglia S, Nascimento FJA, Bartoli M, Klawonn I, Brüchert V. 2014. Meiofauna increases bacterial denitrification in marine sediments. Nature Communications. 5:5133. doi:10.1038/ncomms6133.
- Bowden DA, Davey N, Fenwick M, George S, Macpherson D, Ray C, Stewart R, Christensen-Field C, Gibson K. 2017. Quantifying Benthic Biodiversity: factual voyage report from RV Tangaroa voyage TAN1701 to Chatham Rise, 4 January – 2 February 2017. New Zealand Aquatic Environment and Biodiversity Report. 185:98.
- Chatham Rock Phosphate. 2014. Marine Consent Application and Environmental Impact Assessment.
- Chiswell SM. 1994. Acoustic Doppler current profiler measurements over the chatham rise. New Zealand Journal of Marine and Freshwater Research. 28:167–178. doi:10.1080/00288330.1994.9516605.
- Christiansen B, Denda A, Christiansen S. 2019. Potential effects of deep seabed mining on pelagic and benthopelagic biota. Marine Policy. 114:103442. doi:10.1016/j.marpol.2019.02.014.
- Clark MR, Althaus F, Schlacher TA, Williams A, Bowden DA, Rowden AA. 2016. The impacts of deep-sea fisheries on benthic communities: a review. ICES Journal of Marine Science: Journal du Conseil. 73:51–69.
- Clark MR, Durden JM, Christiansen S. 2020. Environmental Impact Assessments for deep-sea mining: Can we improve their future effectiveness? Marine Policy. 114. doi:10.1016/j.marpol.2018.11.026.
- Clark MR, Nodder S, Leduc D, Eager C, George S, Hale R, Hart A, Murray C, Rauhina-August L, Ray C, et al. 2021. Resilience of deep-sea benthic communities to the effects of sedimentation (“ROBES”): Voyage Report of Survey 3 (TAN2005), June 2020. NIWA Client Report 2021054WN. https://niwa.co.nz/sites/niwa.co.nz/files/ROBES-Voyage-Report-3-March-2021-NIWA.pdf.
- Clark MR, Nodder S, O'Callaghan J, Browne R, Daymond A, de Joux P, George S, Hale R, Hart A, Jhugroo K, et al. 2019. Resilience of deep-sea benthic communities to the effects of sedimentation (“ROBES”): Voyage report of Survey 2: June 2019. NIWA Client Report 2019330WN.
- Clark MR, Nodder S, O'Callaghan J, Hickey C, Chin C, Eager C, Elliot F, Gamon M, George S, Gerring P, et al. 2018. Resilience of deep-sea benthic communities to the effects of sedimentation (“ROBES”): Voyage Report of Survey 1: May-June 2018. Unpublished NIWA voyage report. 136 p. https://niwa.co.nz/sites/niwa.co.nz/files/ROBES_VoyageReport_1Nov2018-web.pdf.
- Clarke KR, Gorley RN. 2015. PRIMER v7: UserManual/tutorial. PRIMER-E, Plymouth, UK. Collie JS, Escanero GA, Valentine PC. 2000. Photographic evaluation of the impacts of bottom fishing on benthic epifauna. ICES Journal of Marine Science. 57:987–1001.
- Clarke KR, Warwick R. 2001. Change in marine communities. An approach to statistical analysis and interpretation. Plymouth: PRIMER-E Ltd.
- Collie JS, Hall SJ, Kaiser MJ, Poiner IR. 2000. A quantitative analysis of fishing impacts on shelf-sea benthos. Journal of Animal Ecology. 69:785–798.
- Collins C, O’Callaghan J, Clark MR, Nodder SD. 2023. The role of wind and buoyancy forcing on mixed layer depths and productivity on the Chatham Rise from ocean glider and ship-based observations. New Zealand Journal of Marine and Freshwater Research. 1–21. doi:10.1080/00288330.2023.2215533.
- Cullen D. 1975. Petrology, distribution and economic potential of phosphorite deposits on Chatham Rise, east of New Zealand. New Zealand Oceanographic Institute.
- Cullen D. 1980. Distribution, composition and age of submarine phosphorites on Chatham Rise, east of New Zealand. SEPM Special Publication. 28:139–148.
- Cullen DJ. 1987. The submarine phosphate resource on central Chatham Rise. DMFS reports.
- Cullen DJ, Singleton RJ. 1977. The distribution of submarine phosphorite deposits on central Chatham Rise, east of New Zealand. 1. Surface distribution from underwater photographs. NZOI Oceanographic Field Report 10:24p.
- Danovaro D, Gambi C, Dell’Anno A, Corinaldesi C, Fraschetti S, Vanreusel A, Vincx M, Gooday AJ. 2008. Exponential decline of deep-sea ecosystem functioning linked to benthic biodiversity loss. Current Biology. 18:1–8. doi:10.1016/j.cub.2007.11.056.
- Dawson E. 1984. The benthic fauna of the Chatham Rise: an assessment relative to possible effects of phosphorite mining. Geologisches Jahrbuch D. 65:213–235.
- Eleftheriou A, Moore DC. 2005. Macrofauna techniques. In: Methods for the study of marine benthos (Editor: Eleftheriou A). 3rd edition. Oxford: Blackwell Science; p. 160–228.
- EPA. 2015. Decision on Marine Consent Application Chatham Rock Phosphate Limited to Mine Phosphorite Nodules on the Chatham Rise.
- Epstein G, Middelburg JJ, Hawkins JP, Norris CR, Roberts CM. 2021. The impact of mobile demersal fishing on carbon storage in seabed sediments. Global Change Biology. 28:2875–2894. doi:10.1111/gcb.16105.
- FAO. 2009. Management of deep-sea fisheries in the high seas. Rome, Italy: FAO. 73 p.
- Flores F, Hoogenboom MO, Smith LD, Cooper TF, Abrego D, Negri AP. 2012. Chronic exposure of corals to fine sediments: lethal and sub-lethal impacts. PloS ONE 7:e37795.
- Gallucci F, Moens T, Fonseca G. 2009. Small-scale spatial patterns of meiobenthos in the Arctic deep sea. Marine Biodiversity. 39:9–25. doi:10.1007/s12526-009-0003-x.
- Giere O. 2009. Meiobenthology: the microscopic motile fauna of aquatic sediments. Berlin: Springer-Verlag.
- Gollner S, Kaiser S, Menzel L, Jones DOB, et al. 2017. Resilience of benthic deep-sea fauna to mining activities. Marine Environmental Research. 129:76–101. doi:10.1016/j.marenvres.2017.04.010.
- Grove S, Probert PK, Berkenbusch K, Nodder S. 2006. Distribution of bathyal meiofauna in the region of the Subtropical Front, Chatham Rise, south-west Pacific. Journal of Experimental Marine Biology and Ecology. 330:342–355. doi:10.1016/j.jembe.2005.12.038.
- Hadfield M, Rickard G, Nodder SD. 2011. Oceanographic models of Chatham Rise for sediment dispersal estimates. Prepared for Chatham Rock Phosphate Limited. NIWA Client report WSE11301, 27 pp.
- Hadfield M, Rickard GJ, Uddstrom MJ. 2007. A hydrodynamic model of Chatham Rise, New Zealand. New Zealand Journal of Marine and Freshwater Research. 41:239–264. doi:10.1080/00288330709509912.
- Hale R, Godbold JA, Sciberras M, Dwight J, Wood J, Hiddink JG, Solan M. 2017. Mediation of macronutrients and carbon by post-disturbance shelf sea sediment communities. Biogeochemistry. 135:121–133. doi:10.1007/s10533-017-0350-9.
- Hale R, Murray C, Stewart R, Eager C, Hickey C, Leduc D, Rowden AA, Nodder SD, Pilditch C, Clark MR. in prep. Simulated deep sea mining disturbance alters the linkage between infaunal biomass and benthic sediment community oxygen consumption on the Chatham Rise.
- Hasemann C, Mokievsky V, Sablotny B, Tekman MB, Soltwedel T. 2020. Effects of sediment disturbance on deep-sea nematode communities: Results from an in-situ experiment at the Arctic LTER observatory HAUSGARTEN. Journal of Experimental Marine Biology and Ecology. 533:151471. doi:10.1016/j.jembe.2020.151471.
- Hauquier F, Leliaert F, Rigaux A, Derycke S, Vanreusel A. 2017. Distinct genetic differentiation and species diversification within two marine nematodes with different habitat preference in Antarctic sediments. BMC Evolutionary Ecology. 17:120.
- Heath RA. 1983. Observations on Chatham Rise currents. New Zealand Journal of Marine and Freshwater Research. 17:321–330. doi:10.1080/00288330.1983.9516006.
- Hein Jr, Mizell K, Koschinsky A, Conrad TA. 2013. Deep-ocean mineral deposits as a source of critical metals for high- and green-technology applications: Comparison with land-based resources. Ore Geology Reviews. 51:1–14. doi:10.1016/j.oregeorev.2012.12.001.
- Hendrick VJ, Hutchison ZL, Last KS. 2016. Sediment burial intolerance of marine macroinvertebrates. PLoS One. 11:e0149114. doi:10.1371/journal.pone.0149114.
- Hinchen H, Gallyot J, Carter A, Ferguson M, Webb K, Nelson M, Jenkins C. 2021. Detecting the impacts on UK sublittoral rock communities of resuspended sediments from fishing activity. Ecological Indicators. 125:107545. doi:10.1016/j.ecolind.2021.107545.
- Hinz H, Hiddink JG, Forde J, Kaiser MJ. 2008. Large-scale responses of nematode communities to chronic otter-trawl disturbance. Canadian Journal of Fisheries and Aquatic Sciences. 65:723–732. doi:10.1139/f08-002.
- Ingole BS, Ansari ZA, Rathod V, Rodrigues N. 2000. Response of meiofauna to immediate benthic disturbance in the central Indian Ocean basin. Marine Georesources & Geotechnology. 18:263–272. doi:10.1080/10641190009353794.
- Ingole BS, Goltekar R, Gonsalves S, Ansari ZA. 2005. Recovery of deep-sea meiofauna after artificial disturbance in the Central Indian Basin. Marine Georesources & Geotechnology. 23:253–266. doi:10.1080/10641190500446540.
- Jones DOB, Kaiser S, Sweetman AK, Smith CR, Menot L, Vink A, et al. 2017. Biological responses to disturbance from simulated deep-sea polymetallic nodule mining. PLoS One. 12:e0171750.
- Kaiser MJ, Ramsay K, Richardson CA, Spence FE, Brand AR. 2001. Chronic fishing disturbance has changed shelf sea benthic community structure. Journal of Animal Ecology. 69:494–503. doi:10.1046/j.1365-2656.2000.00412.x.
- Kudrass HR, Von Rad U. 1984a. Underwater television and photography observations, side-scan sonar and acoustic reflectivity measurements of phosphorite-rich areas on the Chatham Rise (New Zealand). Geologisches Jahrbuch. Reihe D. Mineralogie, Petrographie, Geochemie, Lagerstättenkunde. 69–89.
- Kudrass HR, Von Rad U. 1984b. Geology and some mining aspects of the Chatham Rise phosphorite: a synthesis of Sonne-17 results. Geologisches Jahrbuch. Reihe D. Mineralogie, Petrographie, Geochemie, Lagerstättenkunde. 233–252.
- Lampadariou N, Hatziyanni E, Tselepides A. 2005. Meiofaunal community structure in Thermaikos Gulf: Response to intense trawling pressure. Continental Shelf Research. 25:2554–2569. doi:10.1016/j.csr.2005.08.016.
- Leduc D, Rowden AA, Pilditch CA, Maas EW, Probert PK. 2013. Is there a link between deep-sea biodiversity and ecosystem function? Marine Ecology. 34:334–344. doi:10.1111/maec.12019.
- Leduc D, Rowden AA, Probert PK, Pilditch CA, Nodder SD, Vanreusel A, Duineveld GCA, Witbaard R. 2012. Further evidence for the effect of particle-size diversity on deep-sea benthic biodiversity. Deep Sea Research Part I: Oceanographic Research Papers. 63:164–169. doi:10.1016/j.dsr.2011.10.009.
- Leduc D, Rowden AA, Torres LG, Nodder SD, Pallentin A. 2015. Distribution of macro-infaunal communities in phosphorite nodule deposits on Chatham Rise, Southwest Pacific: implications for management of seabed mining. Deep Sea Research Part I: Oceanographic Research Papers. 99:105–118. doi:10.1016/j.dsr.2015.01.006.
- Lescinski J, Jeuken C, Cronin K, Vroom J, Elias E. 2014. Modelling investigations on mine tailing plume dispersion on the Chatham Rise. Report prepared by Deltares for Chatham Rock Phosphate Ltd. Deltares Final Report 1209110-000-ZKS-0007. 131p. plus Appendices.
- Levin LA, Mengerink K, Gjerde KM, Rowden AA, Dover V, Clark CL, Ramirez-Llodra MR, Currie E, Smith B, Sato CR, N K. 2016. Defining “serious harm” to the marine environment in the context of deep-seabed mining. Marine Policy. 74:245–259. doi:10.1016/j.marpol.2016.09.032.
- Mackay A, Gregg P, Syers J. 1980. A preliminary evaluation of Chatham Rise phosphorite as a direct-use phosphatic fertiliser. New Zealand Journal of Agricultural Research. 23:441–449. doi:10.1080/00288233.1980.10417867.
- Mackay K, Wood B, Clark MR. 2005. Chatham Rise bathymetry. Wellington, New Zealand: National Institute of Water & Atmospheric Research.
- McDougall JC. 1982. Bounty sediments. New Zealand Oceanographic Institute (now NIWA) chart. Oceanic Series. 1:1:000 000.
- Mevenkamp L, Guilini K, Boetius A, de Grave J, Laforce B, Vandenberghe D, Vincze L, Vanreusel A. 2019. Responses of an abyssal meiobenthic community to short-term burial with crushed nodule particles in the south-east Pacific. Biogeosciences. 16:2329–2341. doi:10.5194/bg-16-2329-2019.
- Mevenkamp L, Stratmann T, Guilini K, Moodley L, van Oevelen D, Vanreusel A, Westerlund S, Sweetman A. 2017. Impaired short-term functioning of a benthic community from a deep Norwegian fjord following deposition of mine tailings and sediments. Frontiers in Marine Science. 4:169. doi:10.3389/fmars.2017.00169.
- Miljutin DM, Miljutina MA, Arbizu PM, Galeron J. 2011. Deep-sea nematode assemblage has not recovered 26 years after experimental mining of polymetallic nodules (Clarion-Clipperton Fracture Zone, Tropical Eastern Pacific). Deep Sea Research Part I: Oceanographic Research Papers. 58:885–897. doi:10.1016/j.dsr.2011.06.003.
- Mobilia V, Cummings VJ, Clark MR, Tracey D, Bell JJ. 2021. Short-term physiological responses of the New Zealand deep-sea sponge Ecionemia novaezealandiae to elevated concentrations of suspended sediments. Journal of Experimental Marine Biology and Ecology. 541:151579. doi:10.1016/j.jembe.2021.151579.
- Moens T, Braeckman U, Derycke S, Fonseca G, Gallucci F, Gingold R, Guilini K, Ingels J, Leduc D, Vanaverbeke J, et al. 2014. Ecology of free-living marine nematodes. In: Schmidt-Rhaesa A, editor. Handbook of zoology: gastrotricha, cycloneuralia and gnathifera volume 2: nematoda. Berlin: De Gruyter; p. 109–152.
- Morono Y, Terada T, Kallmeyer J, Inagaki F. 2013. An improved cell separation technique for marine subsurface sediments: applications for high-throughput analysis using flow cytometry and cell sorting. Environmental Microbiology. 15:2841–2849. doi:10.1111/1462-2920.12153.
- Morono Y, Terada T, Masui N, Inagaki F. 2009. Discriminative detection and enumeration of microbial life in marine subsurface sediments. The ISME Journal. 3:503–511. doi:10.1038/ismej.2009.1.
- Murray C, Rowden AA, Leduc D, Nodder SD, Hale R, Halliday J, Clark MR. submitted. Effects of experimental in situ seabed disturbance on deep-sea macrofaunal communities of Chatham Rise. Southwest Pacific. New Zealand Journal of Marine and Freshwater Research.
- Nascimento FJ, Näslund J, Elmgren R. 2012. Meiofauna enhances organic matter mineralization in soft sediment ecosystems. Limnology and Oceanography. 57:338–346. doi:10.4319/lo.2012.57.1.0338.
- Nelson CS, Lawless AS, Nodder SD, Zwingmann H. 2022. Latest Miocene (Kapitean/Messinian) glauconite and the central Chatham Rise greensand: an enigmatic, highly condensed, relict/palimpsest deposit on the modern seafloor. New Zealand Journal of Geology and Geophysics. 65:529–554. doi:10.1080/00288306.2021.1977341.
- Niner HJ, Ardron JA, Escobar EG, Gianni M, Jaeckel A, Jones DOB, Levin LA, Smith CR, Thiele T, Turner PJ, et al. 2018. Deep-sea mining with no net loss of biodiversity—An impossible aim. Frontiers in Marine Science. 5:53. doi:10.3389/fmars.2018.00053.
- Nodder SD, Bowden DA, Pallentin A, Mackay K. 2012. Seafloor habitats and benthos of a continental ridge: Chatham Rise, New Zealand. In: Seafloor Geomorphology as Benthic Habitat. Elsevier; p. 763–776.
- Nodder SD, Chiswell SM, Northcote LC. 2016. Annual cycles of deep-ocean biogeochemical export fluxes in subtropical and subantarctic waters, southwest Pacific Ocean. Journal of Geophysical Research: Oceans. 121:2405–2424. doi:10.1002/2015JC011243.
- Nodder SD, Duineveld GC, Pilditch CA, Sutton PJ, Probert PK, Lavaleye MS, Witbaard R, Chang FH, Hall JA, Richardson KM. 2007. Focusing of phytodetritus deposition beneath a deep-ocean front, Chatham Rise, New Zealand. Limnology and Oceanography. 52:299–314. doi:10.4319/lo.2007.52.1.0299.
- Nodder SD, Eager C, O’Callaghan J, Leduc D, Deppeler S, Gerring P, Searson S, Quinn W, George S, Overden R, et al. in prep. Near-bed sediment dynamics and fluxes within the Subtropical Frontal Zone on Chatham Rise crest, and implications for deep-sea bottom trawling and seabed mining.
- Nodder SD, Northcote LC. 2001. Episodic particulate fluxes at southern temperate mid-latitudes (42–45°S) in the Subtropical Front region, east of New Zealand. Deep Sea Research Part I: Oceanographic Research Papers. 48:833–864. doi:10.1016/S0967-0637(00)00062-5.
- Nodder SD, Pilditch CA, Probert PK, Hall JA. 2003. Variability in benthic biomass and activity beneath the Subtropical Front, Chatham Rise, SW Pacific Ocean. Deep Sea Research Part I: Oceanographic Research Papers. 50:959–985. doi:10.1016/S0967-0637(03)00094-3.
- Nomaki H, Mochizuki T, Kitahashi T, Nunoura T, Arai K, Toyokufu T, Tanaka G, Shigeno S, Tasumi E, Fujikura K, Watanabe S. 2015. Effects of mass sedimentation events after the 2011 off the Pacific coast of Tohoku Earthquake on benthic prokaryotes and meiofauna inhabiting the upper bathyal sediments. Journal of Oceanography. 72:113–128. doi:10.1007/s10872-015-0293-5.
- Orcutt BN, Bradley JA, Brazelton WJ, Estes ER, Goordial JM, Huber JA, Jones RM, Mahmoudi N, Marlow JJ, Murdock S, Pachiadaki M. 2020. Impacts of deep-sea mining on microbial ecosystem services. Limnology and Oceanography. 65:1489–1510. doi:10.1002/lno.11403.
- Paradis S, Goni M, Masque P, Duran R, Arjona-Camas M, Palanques A, Puig P. 2021. Persistence of biogeochemical alterations of deep-sea sediments by bottom trawling. Geophysical Research Letters. 48:e2020GL091279. doi:10.1029/2020GL091279.
- Pilditch C, Leduc D, Nodder SD, Probert PK, Bowden D. 2015. Spatial patterns and environmental drivers of benthic infaunal community structure and ecosystem function on the New Zealand continental margin. New Zealand Journal of Marine and Freshwater Research. 49:224–246. doi:10.1080/00288330.2014.995678.
- Pinheiro M, Oliveira A, Barros S, Alves N, Raimundo J, Caetano M, Coimbra J, Neuparth T, Santos MM. 2021. Functional, biochemical and molecular impact of sediment plumes from deep-sea mining on Mytilus galloprovincialis under hyperbaric conditions. Environmental Research. 195:110753. doi:10.1016/j.envres.2021.110753.
- Pranovi F, Raicevich S, Franceschini G, Farrace MG, Giovanardi O. 2000. Rapido trawling in the northern Adriatic Sea: effects on benthic communities in an experimental area. ICES Journal of Marine Science. 57:517–524. doi:10.1006/jmsc.2000.0708.
- Pusceddu A, Bianchelli S, Martin J, Puig P, Palanques A, Masque P, Danovaro R. 2014. Chronic and intensive bottom trawling impairs deep-sea biodiversity and ecosystem functioning. Proceedings of the National Academy of Sciences. 111:8861–8866. doi:10.1073/pnas.1405454111.
- Radziejewska T. 2002. Responses of deep-sea meiobenthic communities to sediment disturbance simulating effects of polymetallic nodule mining. International Review of Hydrobiology. 87:457.
- Radziejewska T, Drzycimski I, Galtsova W, Kulangieva LV, Stoyanova V. 2001. Changes in genus-level diversity of meiobenthic free-living nematodes (Nematoda) and harpacticoids (Copepoda harpacticoida) at an abyssal site following experimental sediment disturbance. Proceedings of the 4th (2001) Isope Ocean Mining Symposium. 38–43.
- Ramirez-Llodra E, Brandt A, Danovaro R, De Mol B, Escobar E, German CR, Levin LA, Martinez Arbizu P, Menot L, Buhl-Mortensen P, et al. 2010. Deep, diverse and definitely different: unique attributes of the world’s largest ecosystem. Biogeosciences. 7:2851–2899. doi:10.5194/bg-7-2851-2010.
- Rosenfeld M, Bresler V, Abelson A. 1999. Sediment as a possible source of food for corals. Ecology Letters. 2:345–348. doi:10.1046/j.1461-0248.1999.00097.x.
- Rowden AA, Leduc D, Torres L, Bowden DA, Hart A, Chin C, Davey N, Wright J, Carter M, Crocker B, et al. 2014. Benthic communities of MPL area 50270 on the Chatham Rise. NIWA Client Report WLG2012-25 prepared for Chatham Rock Phosphate Ltd. 102pp. https://epa.govt.nz/assets/FileAPI/proposal/EEZ000006/Applicants-proposal-documents/e8784b016d/EEZ000006-Appendix15-Rowden-et-al-Benthic-Communities-Report.pdf.
- Rysgaard S, Christensen PB, Sørensen MV, Funch P, Berg P. 2000. Marine meiofauna, carbon and nitrogen mineralization in sandy and soft sediments of Disko Bay, West Greenland. Aquatic Microbial Ecology. 21:59–71. doi:10.3354/ame021059.
- Sané E, Martin J, Puig P, Palanques A. 2013. Organic biomarkers in deep-sea regions affected by bottom trawling: pigments, fatty acids, amino acids and carbohydrates in surface sediments from the La Fonera (Palamós) Canyon, NW Mediterranean Sea. Biogeosciences. 10:8093–8108. doi:10.5194/bg-10-8093-2013.
- Sartory D. 1982. Spectrophotometric analysis of chlorophyll a in freshwater plankton. Pretoria, South Africa: Department of Environmental Affairs Hydrological Research Institute. Technical Report TR 115.
- Schratzberger M, Dinmore TA, Jennings S. 2002. Impacts of trawling on the diversity, biomass and structure of meiofauna assemblages. Marine Biology. 140:83–93. doi:10.1007/s002270100688.
- Schratzberger M, Ingels J. 2018. Meiofauna matters: The roles of meiofauna in benthic ecosystems. Journal of Experimental Marine Biology and Ecology. 502:12–25. doi:10.1016/j.jembe.2017.01.007.
- Schratzberger M, Jennings S. 2002. Impacts of chronic trawling disturbance on meiofaunal communities. Marine Biology. 141:991–1000. doi:10.1007/s00227-002-0895-5.
- Schratzberger M, Rees HL, Boyd SE. 2000. Effects of simulated deposition of dredged material on structure of nematode assemblages - the role of burial. Marine Biology. 136:519–530. doi:10.1007/s002270050712.
- Sciberras M, Parker R, Powell C, Robertson C, Kroger S, Bolam S, Hiddink JG. 2016. Impacts of bottom fishing on the sediment infaunal community and biogeochemistry of cohesive and non-cohesive sediments. Limnology and Oceanography. 61:2076–2089. doi:10.1002/lno.10354.
- Sharma R, Bath BN, Sankar SJ. 2005. Monitoring the impact of simulated deep-sea mining in Central Indian Basin. Marine Georesources & Geotechnology. 23:339–356. doi:10.1080/10641190500446763.
- Sharma R, Nath B, Parthiban N, Sankar G, J S. 2001. Sediment redistribution during simulated benthic disturbance and its implications on deep seabed mining. Deep Sea Research Part II: Topical Studies in Oceanography. 48:3363–3380. doi:10.1016/S0967-0645(01)00046-7.
- Simon-Lledó E, Bett BJ, Huvenne VAI, Köser K, Schoening T, Greinert J, Jones DOB. 2019. Biological effects 26 years after simulated deep-sea mining. Scientific Reports. 9:8040. doi:10.1038/s41598-019-44492-w.
- Smith CR, De Leo FC, Bernardino AF, Sweetman AK, Martinez Arbizu P. 2008. Abyssal food limitation, ecosystem structure and climate change. Trends in Ecology & Evolution. 23:518–528. doi:10.1016/j.tree.2008.05.002.
- Smith KL, Ruhl HA, Bett BJ, Billett DSM, Lampitt RS, Kaufman RS. 2009. Climate, carbon cycling, and deep-ocean ecosystems. Proceedings of the National Academy of Sciences. 106:19211–19218. doi:10.1073/pnas.0908322106.
- Snelgrove PVR, Soetaert K, Solan M, Thrush S, Wei CL, Danovaro R, Fulweiler RW, Kitazato H, Ingole B, Norkko A, et al. 2018. Global carbon cycling on a heterogeneous seafloor. Trends in Ecology & Evolution. 33:96–105. doi:10.1016/j.tree.2017.11.004.
- Somerfield PJ, Warwick RM. 1996. Meiofauna in marine pollution monitoring programmes: A laboratory manual. Lowestoft: Ministry of Agriculture, Fisheries and Food. 71.
- Stewart TJ, Stagpoole VM, Wood RA, Carter L. 2016. Ploughmarks and pits on the Chatham Rise: a record of deep-keeled Antarctic icebergs at 43° 20′ S. In: M Jakobsson, BJ Todd, EK Dowdeswell, K Hogan, editor. Atlas of submarine glacial landforms: modern, quaternary and ancient. London: Geological Society; p. 275–276.
- Thiel H, Schriever G. 1990. Environmental protection of the deep sea and the DISCOL project Ambio. 19:245–250.
- Thrush SF, Ellingsen KE, Davis K. 2016. Implications of fisheries impacts to seabed biodiversity and ecosystem-based management. ICES Journal of Marine Science. 73:i44–i50. doi:10.1093/icesjms/fsv114.
- Thurber AR, Sweetman AK, Narayanaswamy BE, Jones DOB, Ingels J, Hansman RL. 2014. Ecosystem function and services provided by the deep sea. Biogeosciences. 11:3941–3963. doi:10.5194/bg-11-3941-2014.
- Tiano JC, van der Rejiden K, O’Flynn S, Beauchard O, ven der Ree S, van der Wees J, Ysebaert T, Soetaert K. 2020. Experimental bottom trawling finds resilience in large-bodied infauna but vulnerability for epifauna and juveniles in the Frisian Front. Marine Environmental Research. 159:104964. doi:10.1016/j.marenvres.2020.104964.
- Tiano JC, Witbaard R, Bergman MJN, van Rijswijk P, Tramper A, van Oevelen D, Soetaert K. 2019. Acute impacts of bottom trawl gears on benthic metabolism and nutrient cycling. ICES Journal of Marine Science. 76:1917–1930. doi:10.1093/icesjms/fsz060.
- Vonnahme TR, Molari M, Janssen F, Wenzhofer F, Haeckel M, Titschack J, Boetius A. 2020. Effects of a deep-sea mining experiment on seafloor microbial communities and functions after 26 years. Science Advances. 6:eaaz5922. doi:10.1126/sciadv.aaz5922.
- Weaver PPE, Aguzzi J, Boschen-Rose RE, Colaco A, de Stigter H, Gollner S, Haeckel M, Hauton C, Helmons R, Jones DOB, et al. 2022. Assessing plume impacts caused by polymetallic nodule mining vehicles. Marine Policy. 139:105011. doi:10.1016/j.marpol.2022.105011.
- Whomersley P, Huxham M, Schratzberger M, Solan S. 2009. Differential response of meio- and macrofauna to in situ burial. Journal of the Marine Biological Association of the United Kingdom. 89:1091–1098. doi:10.1017/S0025315409000344.
- Wulff A, Sundback K, Nilsson C, Jonsson B. 1997. Effect of sediment load on the microbenthic community of a shallow-water sandy sediment. Estuaries. 20:547–558. doi:10.2307/1352613.