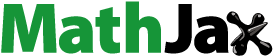
Abstract
Objectives
The outcome of balloon-based atherosclerosis thermoplasty is closely related to the temperature/stress distribution during the treatment. For precise prediction of a required thermal lesion in the heterogeneous and thin atherosclerotic vessel, a numerical model incorporating heat-induced tissue expansion or shrinkage and the strain caused by balloon dilation is necessary.
Methods
A fully coupled thermal-electrical-structural new model was established. The model features a heterogeneous structure including eccentric plaque, healthy artery and surrounding tissue. Tissue expansion/shrinkage and hyperelasticity material model were taken into consideration. Different heating strategies and plaque mechanical properties were investigated. The temperature distribution was compared with the traditional thermal-electrical coupled model. The possibility of thermoplasty treatment using balloons with different sizes was also explored.
Results
The temperature, the electrical intensity and the stress during the thermoplasty were obtained. Lower stress was found in the heating region where tissue shrinkage occurred. The ablation depth was predicted to be ∼0.42 mm larger without coupling the biomechanical influence. The mechanical properties and input condition significantly affect the temperature and stress distribution considering the small dimensions of the tissue. Besides, with a 12.5% reduction of balloon diameter, the largest Von Mises stress decreases by 25.4%.
Conclusions
It is confirmed that a coupled thermal-electrical-structural model is needed for precise temperature prediction in the balloon-based thermoplasty of the heterogeneous and thin tissue. The model presented may help with future development of optimized treatment planning considering both ablation depth and minimum stress.
Introduction
Atherosclerosis is a disease in which plaque builds up in the artery, narrowing the lumen. It results in almost 30% of all mortalities worldwide [Citation1]. Balloon angioplasty and stenting, which mechanically open and support the narrowed artery are the conventional therapies. However, it would cause artery dissection and restenosis due to the mechanical force and the following series of inflammation [Citation2,Citation3]. To treat atherosclerosis effectively, the therapy should open the narrowed blood passage, avoid artery dissection, ablate the plaque and kill the proliferated cells for long-term patency.
Combined with the balloon-based technique, thermal treatment has been utilized to treat atherosclerosis. In the traditional balloon-based thermoplasty, the balloon opens the narrowed lumen and provides close contact between the radiofrequency electrodes and the tissue. The energy acts on the atherosclerotic lesion and heats the entire vessel wall [Citation4]. The preclinical studies demonstrated that the high temperature could mold the plaque and obtain a larger lumen diameter than plain balloon angioplasty [Citation5]. Moreover, thermal energy could fuse the tunica media and intima and thus maintain the structure’s integrity [Citation4,Citation6]. A recent study confirmed that heating the artery could decrease the intra-plaque vessel density and SMCs’ contents [Citation7]. However, the long-term outcome of the existing thermal treatment of atherosclerosis was reported to be no better than conventional treatments [Citation8]. Thus, a novel thermal therapy for atherosclerosis: heating the deeper site while sparing the intimal tissue [Citation9] has been proposed to decrease the rate of restenosis.
However, according to the thermal effect studies, the outcomes were closely associated with the temperature and stress distributions. Previous study demonstrated that the proliferation of smooth muscle cells would be inhibited at 50 °C for 1 min [Citation10], indicated by the level of ki67, as hyperthermia would induce significant DNA damage to cells in G1-phase [Citation11]. The thickness of artery walls can be significantly decreased, and elastic fiber fusion happened when heated at 70 °C [Citation12]. When heated at a power of 30 W for 30 s, the perforating ulcer occurs in the esophagus, which suggests that high dosage of heat may result in thin wall perforation [Citation13]. Besides, the magnitude of stress was the main factor of artery dissection (a tear along the inside wall of an artery) and plaque rupture [Citation14]. While the existing measurement technique is difficult to obtain the temperature and stress distribution in the thin wall (only 1 ∼ 2 mm thick). A numerical simulation of both temperature and stress distribution during the thermal treatment of plaque is necessary.
In the balloon-based treatment, the temperature, structural and electrical fields are fully coupled (). The joule heat induced by radiofrequency energy is the heat source. In turn, as the temperature increases, the increase of the electrical conductivity would alter the electric field distribution. The elevated temperature would induce thermal expansion [Citation15,Citation16]. When the tissue temperature exceeds 60 °C, the collagen would be denatured, and the tissue would shrink (about 52–74% of initial volume) [Citation17]. The thermal expansion and shrinkage would add extra strain in the deformation and stress fields. The deformation of tissue induced by the balloon expansion and temperature alteration would change the relative position of electrodes and tissue, and then alter the electrical distribution.
Current numerical models for radiofrequency ablation are thermal-electrical coupled models [Citation17,Citation18] and not involved with the large deformation induced by balloon dilation and the considerable shrinkage caused by the elevated temperature. An ablation model incorporating tissue contraction in microwave ablation with a treatment needle-probe has been proposed [Citation18]. However, it is for a large homogeneous tissue, and is not suitable for the heterogeneous and thin tissue, such as an atherosclerotic lesion. Nevertheless, as a fiber-reinforced material [Citation19], the artery’s deformation induced by the balloon dilation was large and distinct. The mechanical response of the artery should be described with the continuum mechanics.
Therefore, this study aims to propose a fully coupled temperature-electrical-structural model to investigate the temperature and stress distribution in the balloon-based thermoplasty for atherosclerosis. Both the temperature and stress distribution in different heating modes would be obtained. The temperature distribution would be compared with the thermal-electrical model. The impact of mechanical properties, input heating condition and balloon with different sizes will be assessed.
Methods
Geometry
The stenotic artery with the surrounding tissue is simulated by a 3-layer hollow cylinder according to the clinical anatomic data (). The three layers are the eccentric plaque, the healthy artery and the surrounding tissue, respectively. A total length is 4 mm for decreasing computational cost. The artery has an inner diameter of 2.5 mm, and a thickness of 0.34 mm based on the statistical data [Citation20]. The surrounding tissue’s radius is 5 mm, which is much larger than that of the plaque. The dimension of plaque affected the blood flow [Citation21,Citation22]. Herein stenotic rate of 80% is chosen, which is classified as severe stenosis. Based on the evolution model of plaque [Citation23], the plaque has an inner diameter of 1.25 mm. The eccentricity of the plaque (the thinnest dimension of the plaque divided by the width of the opposite wall) is 0.35 [Citation24]. The bipolar RF electrodes in close contact with the plaque are 1 mm in width and 2 mm in length.
Physical model
The treatment process could be simplified into three steps:
Artery prestretch: There exists preexisting stress and strain in the artery wall in the physiological conditions. To account for the preexisting stress, a pre-stretch is usually used. The artery is imposed to tension and has 10% extension in the axial direction. Besides, the inner surface of blood is imposed by the average blood pressure (13.3 kPa) [Citation25].
Balloon dilation: the balloon inflation is modeled as a displacement-driven process used in the study [Citation26]. The balloon is modeled as a virtual balloon, imposing a displacement boundary condition in the inner surface.
Radiofrequency heating: two common control models were investigated: constant voltage heating without internal cooling and temperature control mode with internal cooling. The former was used in the traditional thermoplasty. The latter was proposed in our previous work [Citation9,Citation27].
Governing equation
In the model, there were three physical fields, namely solid mechanics, heat transfer and electrical fields.
Solid mechanics
The stress is calculated using the mechanical equilibrium equation, given the relevant loading and boundary conditions.
The mechanical property of artery and plaque depends on the load applied. In the physiological range, they are usually modeled as a linear elastic material. However, with the increase of load, the artery and plaque are stiffened and the elastic modulus increase [Citation25]. So the artery and plaque are modeled as the hyperelastic materials, based on Neo-Hookean constitutive equation:
(1)
(1)
where W is the strain energy function per unit of reference volume;
is the first deviatoric strain invariant; J is the elastic volume ratio;
is the coefficient and K is the bulk modulus. The strain energy density function is a scalar-valued function that relates the strain energy density of a material to the deformation gradient, from which we can get the stress by mathematical calculation.
(2)
(2)
where S is the second Piola–Kirchhoff stress, C is the right Cauchy–Green Tensor. The detailed description of continuum mechanics can be found in reference [Citation28].
In the study, a linear thermal expansion model is used to describe the thermally induced strain of the tissue. Considering thermal stress, the physical equation takes the following form:
(3)
(3)
(4)
(4)
(5)
(5)
where
is the strain tensor caused by the change in temperature, α is the thermal expansion/contraction coefficient, T is the current temperature, F, Fel and Fth are the total, elastic and thermal deformation tensor, respectively. The detailed description of function f is shown in the properties section.
Electrical field
A quasi-electrostatic equation is used to simulate the RF propagation in the tissue. The governing equation is [Citation29]:
(6)
(6)
where
and V are the electrical conductivity (S·m−1) and the electrical potential (volt).
Heat transfer
The thermal conduction equation is used to simulate the heat transfer process,
(7)
(7)
where
(kg/m3) and
(J·kg−1·K−1) are the density and the specific heat capacity,
represents thermal conductivity (W·m−1·K−1). Term
represents the displacement of tissue. Term
represents the heat source due to external radiofrequency volume heating (W·m−3). It is calculated based on Ohm’s law
(8)
(8)
where
represents the electrical conductivity (S·m−1) and V (volt) is the electrical potential.
Boundary conditions
The boundaries and loading conditions are set as follows:
Artery prestretch: One end of the tissue is fixed; a displacement of 0.4 mm along the axial direction is loaded to the other end of the tissue. An intra-luminal pressure of 13.3 kPa is applied on the inner surface during the whole simulation process. The outer surface of the tissue cylinder is assumed as a free surface.
Balloon dilation: A radial displacement is imposed on the inner wall of the stenotic artery to simulate the process of balloon expansion. In the full expansion case, the displacement is 0.75 mm, while it was 0.5 mm in the incomplete expansion case used a balloon with diameter of 3.75 mm.
Radiofrequency heating:
For the electrical boundary conditions, a RF voltage V =
0 is applied in one electrode while the other one is set to be 0 V. For the traditional thermoplasty cases, the V0 is set to be 25, 20 and 15 V. In the newly proposed thermoplasty, the V0 is determined by a PID algorithm. In the algorithm, the measurement value is the temperature of a point located in the middle of the positive electrode edge close to the negative electrode. The target temperature is set to be 42, 45 and 48 °C.
For the thermal boundary conditions, the outer boundary of the surrounding tissue is constant and at 37 °C. In the traditional thermoplasty, the adiabatic condition is applied to the inner surface. In the newly proposed thermoplasty, a convective condition was applied to the inner surface. The convective coefficient is 8000, 5000 and 2000 W/(m2·K), respectively. The temperature of the cooling water is 10 °C [Citation10].
Properties
Mechanical properties
In the study, a fibrous plaque was chosen. Three different mechanical properties representing the soft, median and hard plaque are used. The surrounding tissue is modeled as a linear elastic material. The mechanical properties are listed in .
Table 1. Material coefficients of tissue.
Thermal and electrical properties
The thermal and electrical conductivities vary linearly with temperature [Citation31].
(9)
(9)
(10)
(10)
where k and σ are the thermal and electrical conductivity (W·m−1·K−1, S·m−1). k0 and σ0 are the thermal and electrical conductivity at reference temperature Tref (W·m−1·K−1, S·m−1). k1 and k2 represent the temperature coefficient of thermal and electrical conductivity (%·K−1). The thermal and electrical properties are listed in .
Table 2. Electrical and thermal coefficients of tissue.
Thermal expansion and contraction properties
As the temperature increases from the physiological temperature to the ablation temperature, the tissue would go through two phases: expansion and contraction. When the temperature is below the transition point, tissue expands. The linear thermal expansion coefficient for the artery/normal tissue and plaque are 0.001 and 0.002 K−1, respectively [Citation16]. While when temperature goes higher than the transition point, with high temperature induced crosslink of tissue proteins and fusion of fibers, contraction happens. The transition temperature and shrinkage rate depend on the thermal dosage (duration and temperature) and the tension [Citation37]. As lack of the experimental data, 59.5–65.5 °C is set to be the shrinkage transition temperature, which was cited from a study on collagenous samples (tendon). When the tissue is exposed to heat for sufficient time, the maximum shrinkage rate is 35.35% [Citation37]. Assuming the shrinkage occurs evenly distributed in the range from 59.5 to 65.5 °C, the increment of the shrinkage rate is calculated to be 0.0589/oC.
For healthy artery and surrounding tissue:
(11)
(11)
where
represents the thermal strain. The thermal expansion coefficient
is
the reference temperature
is 37 °C.
For Plaque:
(12)
(12)
where
represents the increment of thermal strain.
represents the increment of temperature.
Numerical computation implement
The above equations describing the displacement, temperature, and electrical potential are solved together by the software ABAQUS version: 6.14-2 (Simulia, Providence, RI, USA). To apply the piecewise function in the thermal expansion process, the UEXPAND subroutine is used. The whole geometry is meshed with 8-node elements. A total 75,684 meshes are used after checking with the convergence. Of 6 s of heating is chosen for saving the computational cost. The solution takes ∼24 h for each case.
Results
Validation
illustrates the distribution of the circumferential stress from the exploded views of the stenotic fibrous artery after the full expansion of the angioplasty balloon (diameter: 4.0 mm). The eccentric plaque region shows a sandwich-like stress pattern in the circumferential direction. The stress in the inner surface of the plaque and the healthy layer is higher than in the plaque. The largest circumferential stresses appear in the lower part of the healthy artery. The results are similar to the layer-specific model in Holzapfeal’s study [Citation26].
The traditional thermoplasty
illustrates the temperature, electrical intensity, and Von Mises Stress distribution in X–Y and Y–Z cross-sections after 6 s of heating with constant RF voltage 25 V. The highest temperature is found to be 105.47 °C and appears on the inner surface of the artery close to the edge of the electrodes. Along the middle line of electrodes, the temperature decays quickly. Notably, the boundary between plaque and the healthy artery (the dotted line in ) is bent due to plaque shrinkage. The ablation depth (the black 60 °C isothermal line) is about 1.0 mm () which is about half depth of the plaque. The maximum electrical intensity also appears in the edges of electrodes, and it decayed to a quarter of the maximum value within only ∼0.2 mm depth. In , the largest Von Mises Stress appears in the lower region of the healthy artery, while the stress concentration occurs at the edge of electrodes.
Figure 4. The temperature, electrical intensity, Von Mises Stress distribution in X–Y and Y–Z cross-sections after 6 s heating with constant RF voltage 25 V. (a, b) the illustration of X–Y and Y–Z cross-sections; (c, d) the temperature distribution; (e, f) the electrical intensity distribution; (g, h) the Von Mises stress distribution. The dotted lines represent the boundary of the plaque and healthy artery, respectively. The solid black line represents the 60 °C isothermal line. Scale bar: 1 mm.

illustrates the Von Mises stress distribution in the X–Y cross-section before and after 6 s of heating with RF voltage is 25 V. Seen from , the Von Mises stress larger in the lower part of the healthy artery and inner layer than that in other locations when there is no heating. While after 6 s of heating, the stress of the plaque and healthy artery in the heating region decreased significantly. The maximum stress in the upper part of the healthy artery is reduced by 44%. However, there appears two stress concentration points at the edge of electrodes which shall be due to the large temperature alteration.
Figure 5. The Von Mises distribution in the Z cross-section when the positive electrode voltage is 25 V: (a) before heating; (b) after heating. The dotted lines represent the boundary of plaque and healthy artery, respectively. Scale bar: 1 mm.

illustrates the temperature distribution in the cross-section after 6 s of heating with different RF voltage (25, 20 and 15 V). The highest temperature is found to be 105.47, 75.38 and 59.41 °C, respectively. In the 15 V heating group, as temperature is below the transition, there is no contraction happened. The stress distribution is similar to that before heating.
The newly proposed thermoplasty
Full expansion case
illustrates the temperature distribution in the cross-section after 6 s of heating with newly proposed thermoplasty. Unlike the traditional thermoplasty mode, the convection cooling is imposed on the inner surface of the artery. It is found that by this heating strategy. The inner surface temperature is kept lower than 50 °C. The highest temperature reaches 90 °C and appears in the deeper sites. Compared with the constant RF voltage heating, the ablation region is enlarged and covers the plaque above the two electrodes while sparing the healthy artery.
Figure 7. The temperature distribution after 6 s heating in the newly proposed thermoplasty: (a) Z cross-section; (b) Y cross-section. Scale bar: 1 mm.

illustrates the temperature curve along the middle line of electrodes. Comparing results from the proposed thermal-electrical-structural model and that from the thermal-electrical model almost coincided. It is found that the highest temperature is 2.9 °C higher in the thermal-electrical model than the proposed thermal-electrical-structural model. As seen from the results in the original configuration (material coordinates), the ablation depth is about 2.0 mm. Nevertheless, in the deformed configuration (spatial coordinates) and the thermal-electrical model, the ablation depth is only 1.44 and 1.58 mm.
Influence of plaque stiffness, convective coefficient and target temperature
To find out how the heating process may be affected by the mechanical properties of the plaque, three different mechanical properties are used for the plaque with results shown in . It is found that the maximum temperature difference and the difference of ablation depth is 2.65 °C and 100 μm.
As shown in , when treated with the penetrating heating, the highest temperature appears in the deep plaque instead of on the plaque’s inner surface, which spares the healthy artery tissue and prevents the possible boiling process. To find out how the volume RF heating and convection cooling may influence the temperature and stress distribution in the tissue, more results with different convection coefficient and target temperature are obtained (shown in and ).
Figure 10. The temperature and stress distribution in the cross-section after 6 s of heating when convective coefficient is (a) 2000 W/m2·K, (b) 5000 W/m2·K and (c) 8000 W/m2·K with target temperature is 45 °C. Scale bar: 1 mm.

Figure 11. The temperature and stress distribution in the cross-section after 6 s of heating when target temperature is (a) 42 °C, (b) 45 °C and (c) 48 °C with convective coefficient of 8000 W/m2·K. Scale bar: 1 mm.

It is found that with the increase of the convective coefficient, the ablation region spreads and the ablation depths increases from 0, 1.10 to 1.43 mm. The third condition (8000 W/(m2.K)) almost cover the plaque above the electrodes and the region with stress decrease enlarges.
With the increase of target temperature, the ablation depth and the region of stress drop increases. It is worth noting that when the target temperature is set to be 48 °C, heating penetrates to the healthy artery had been ablated, which may need to be avoided in treatment planning. The region of lower stress expands simultaneously with the ablation edge.
Different treatment strategy: incomplete expansion plus thermal treatment
As heat would induce fusion of collagen and elastic fiber, which shall help decrease the plaque volume, thus the balloon may need not to expand to its full range before heating. illustrates the temperature and stress distribution in the X–Y cross-section after 6 s of heating with full and incomplete expansion. The ablation depth is found to be 0.1 mm less than that in the full expansion case, while the largest Von Mises stress decreased by 25.4%. While the ablation shape, the lower stress region, and stress concentration point are similar to those in the full expansion case.
Discussions
In the study, Distribution of the temperature/electrical intensity/stress with different heating mode has been obtained. The distribution of electrical intensity is similar to the results in thermal-electrical model [Citation27]. But, the depth of tissue that could be ablated was 0.42 mm larger than the predicted value in the thermal-electrical model. This value was about 21–42% of the total thickness (artery wall 1–2 mm). This is clearly attributed to the tissue deformation. The results confirm that a model coupling the temperature/electrical intensity/stress together is really important for preoperative temperature prediction process to avoid the perforation or insufficient heating in the artery.
Besides, the results also demonstrate that the heating could lower the stress in balloon-based thermoplasty ( and ), which favors decreasing the rate of media dissection and plaque rupture. Notably, in the newly proposed thermoplasty, the lower temperature of the inner surface decrease the stress of the inner surface compared with 25 V heating ( and ). But on the edge of electrodes, there appear the stress concentrations, which imply during the treatment, the edge of electrodes should avoid to be positioned in the weak sites, like the shoulder of the thin cap of plaque.
Moreover, it is found that when treated with the newly proposed thermoplasty, the perforation may be avoided, as the heating only covers the plaque and the heating lower the stress. When decreasing the convective coefficient, an insufficient ablation would appear and stress decreasing phenomenon disappears. With the target temperature increases, the ablation depth increases and exceeds the plaque, do harm to the healthy artery. The region of lower stress expands simultaneously with the ablation edge. Three different mechanical properties (citing from references) representing the soft, median and hard plaque are used. From the results, it could be found the difference of ablation depth is 100 μm. This suggests that when in real treatment process, other than the temperature sensor, mechanical property sensor may also need for a precise treatment.
The above results also show the dilation degree of balloon significantly affects the temperature and stress distribution. With the same treatment parameters, a larger expansion degree of balloon would obtain a larger ablation depth (0.1 mm larger) while increasing the highest stress by 25.4%. It implied that the high pressure in balloon-based ablation therapy could enlarge the ablation zone, suitable for the thick lesion ablation. In the future, together with accurate prediction of the heating caused volume decrease of the tissue, the model may be used to find an appropriate degree of balloon expansion to ensure adequate ablation and decrease the stress as much as possible.
Though with the presented model, a more realistic prediction of the temperature distribution than previous models have been obtained, further study with even more complicated geometries and experimentally measured material parameters of the fibrous plaque would help further improve the prediction accuracy when computational cost allows. And this is worthy of studying as when treating the thin tissue of the plaque, control of temperature is quite important and difficult. Moreover, during the ablation, boiling of the tissue may result in excessive steam [Citation38] and thus additional unstable mechanical forces on the artery which may add the risk of perforation in clinic. A careful plan of heating process to prevent formation of boiling would help.
Conclusion
In this study, the fully coupled temperature-electrical-structural model has been established. It is confirmed that a coupled thermal-electrical-structural model is needed for the precise temperature prediction in the balloon-based thermoplasty of the heterogeneous and thin tissue. Neglecting the deformation of the artery and plaque would result in significant error in prediction of the ablation depth. Heating through newly proposed RF thermoplasty is found to decrease the stress in the artery while the penetrating heating (with the highest temperature locates in the deep plaque) may also decrease the stress in the plaque but two stress concentration points are found close to the electrodes. The mechanical properties and other heating conditions significantly are found to influence both the temperature and stress distribution. The model would help with future development of optimized treatment planning considering both ablation depth and minimum stress.
Acknowledgments
The authors would like to thank the National Natural Science Foundation of China.
Disclosure statement
No potential conflict of interest was reported by the author(s).
Additional information
Funding
References
- Benjamin EJ, Muntner P, Alonso A, et al. Heart disease and stroke statistics—2019 update: a report from the American heart association. Circulation. 2019;139(10):e56–e528.
- Torrado J, Buckley L, Durán A, et al. Restenosis, stent thrombosis, and bleeding complications: navigating between Scylla and Charybdis. J Am Coll Cardiol. 2018;71(15):1676–1695.
- Armstrong EJ, Brodmann M, Deaton DH, et al. Dissections after infrainguinal percutaneous transluminal angioplasty: a systematic review and current state of clinical evidence. J Endovasc Ther. 2019;26(4):479–489.
- Becker GJ, Lee BI, Waller BF, et al. Radiofrequency balloon angioplasty. Rationale and proof of principle. Invest Radiol. 1988;23(11):810–817.
- Rosen A, Walinksy P, Nardone D, et al. Microwave thermal angioplasty in the normal and atherosclerotic rabbit model. IEEE Microw Guid Wave Lett. 1991;1(4):73–75.
- Lee BI, Becker GJ, Waller BF, et al. Thermal compression and molding of atherosclerotic vascular tissue with use of radiofrequency energy: implications for radiofrequency balloon angioplasty. J Am Coll Cardiol. 1989;13(5):1167–1175.
- Ellenbroek G, van Hout GPJ, de Jager SCA, et al. Radiofrequency ablation of the atherosclerotic plaque: a proof of concept study in an atherosclerotic model. J Cardiovasc Trans Res. 2017;10(2):221–232.
- Sreeram N, Townsend P, Morton D. Radiofrequency thermal balloon angioplasty in an experimental model of peripheral arterial stenosis. Int J Cardiol. 2000;74(1):25–32.
- Zhao S, Zou J, Zhang A, et al. A new RF heating strategy for thermal treatment of atherosclerosis. IEEE Trans Biomed Eng. 2019;66(9):2663–2670.
- Brasselet C, Durand E, Addad F, et al. Effect of local heating on restenosis and in-stent neointimal hyperplasia in the atherosclerotic rabbit model: a dose-ranging study. Eur Heart J. 2008;29(3):402–412.
- Hildebrandt B, Wust P, Ahlers O, et al. The cellular and molecular basis of hyperthermia. Critic Rev Oncol/Hematol. 2002;43(1):33–56.
- Fram DB, Gillam LD, Aretz TA, et al. Low pressure radiofrequency balloon angioplasty: evaluation in porcine peripheral arteries. J Am Coll Cardiol. 1993;21(6):1512–1521.
- Koruth JS, Kuroki K, Kawamura I, et al. Pulsed field ablation versus radiofrequency ablation. Circ Arrhythm Electrophysiol. 2020;13(3):e008303.
- Badel P, Avril S, Sutton MA, et al. Numerical simulation of arterial dissection during balloon angioplasty of atherosclerotic coronary arteries. J Biomech. 2014;47(4):878–889.
- Guinea GV, Atienza JM, Elices M, et al. Thermomechanical behavior of human carotid arteries in the passive state. Am J Physiol Heart Circ Physiol. 2005;288(6):H2940–H2945.
- Guinea GV, Atienza JM, Fantidis P, et al. Increases of corporal temperature as a risk factor of atherosclerotic plaque instability. Ann Biomed Eng. 2008;36(1):66–76.
- Chen SS, Humphrey JD. Heat-induced changes in the mechanics of a collagenous tissue: pseudoelastic behavior at 37 °C. J Biomech. 1997;31(3):211–216.
- Liu D, Brace CL. Numerical simulation of microwave ablation incorporating tissue contraction based on thermal dose. Phys Med Biol. 2017;62(6):2070–2086.
- Holzapfel GA, Gasser TC, Ogden RW. A new constitutive framework for arterial wall mechanics and a comparative study of material models. J Elasticity Phys Sci Solids. 2000;61(1):1–48.
- Gervaso F, Capelli C, Petrini L, et al. On the effects of different strategies in modelling balloon-expandable stenting by means of finite element method. J Biomech. 2008;41(6):1206–1212.
- Abraham JP, Sparrow EM, Lovik RD. Unsteady, three-dimensional fluid mechanic analysis of blood flow in plaque-narrowed and plaque-freed arteries. Int J Heat Mass Transf. 2008;51(23–24):5633–5641.
- J.P A. Numerical analysis of arterial plaque thickness and its impact on artery wall compliance. J Cardiovasc Med Cardiol. 2015;2(2):026–034.
- Ota H, Takase K, Rikimaru H, et al. Quantitative vascular measurements in arterial occlusive disease. Radiographics. 2005;25(5):1141–1158.
- Honye J, Mahon DJ, Jain A, et al. Morphological effects of coronary balloon angioplasty in vivo assessed by intravascular ultrasound imaging. Circulation. 1992;85(3):1012–1025.
- Schulze-Bauer CAJ, Mörth C, Holzapfel GA. Passive biaxial mechanical response of aged human iliac arteries. J Biomech Eng. 2003;125(3):395–406.
- Holzapfel GA, Stadler M, Schulze-Bauer CAJ. A layer-specific three-dimensional model for the simulation of balloon angioplasty using magnetic resonance imaging and mechanical testing. Ann Biomed Eng. 2002;30(6):753–767.
- Zhao S, Zou J, Wang H, et al. A new radiofrequency balloon angioplasty device for atherosclerosis treatment. BioMed Eng OnLine. 2020;19(1):1–18.
- Tadmor EB, Miller RE, Elliott RS. Continuum mechanics and thermodynamics: from fundamental concepts to governing equations. Cambridge: Cambridge University Press; 2012.
- Tungjitkusolmun S, Staelin ST, Haemmerich D, et al. Three-dimensional finite-element analyses for radio-frequency hepatic tumor ablation. IEEE Trans Biomed Eng. 2002;49(1):3–9.
- Noble C, Carlson KD, Neumann E, et al. Patient specific characterization of artery and plaque material properties in peripheral artery disease. J Mech Behav Biomed Mater. 2020;101:103453.
- Rossmann C, Haemmerich D. Review of temperature dependence of thermal properties, dielectric properties, and perfusion of biological tissues at hyperthermic and ablation temperatures. 2015-02-06. Crit Rev Biomed Eng. 2014;42(6):467–492.
- Valvano JW, Cochran J, Diller KR. Thermal conductivity and diffusivity of biomaterials measured with self-heated thermistors. Int J Thermophys. 1985;6(3):301–311.
- Duck FA. Physical properties of tissues: a comprehensive reference book. Cambridge (MA): Academic Press; 2013.
- Slager CJ, Phaff AC, Essed CE, et al. Electrical impedance of layered atherosclerotic plaques on human aortas. IEEE Trans Biomed Eng. 1992;39(4):411–419.
- Gabriel C, Gabriel S, Corthout E. The dielectric properties of biological tissues: I. Literature survey. Phys Med Biol. 1996;41(11):2231–2249.
- Stiles DK, Oakley B. Simulated characterization of atherosclerotic lesions in the coronary arteries by measurement of bioimpedance. IEEE Trans Biomed Eng. 2003;50(7):916–921.
- Wall MS, Deng XH, Torzilli PA, et al. Thermal modification of collagen. J Shoulder Elbow Surg. 1999;8(4):339–344.
- Abraham J, Sparrow E. A thermal-ablation bioheat model including liquid-to-vapor phase change, pressure-and necrosis-dependent perfusion, and moisture-dependent properties. Int J Heat Mass Transf. 2007;50(13–14):2537–2544.