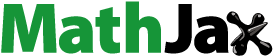
Abstract
Purpose
While radiofrequency catheter ablation (RFCA) creates a lesion consisting of the tissue points subjected to lethal heating, the sublethal heating (SH) undergone by the surrounding tissue can cause transient electrophysiological block. The size of the zone of heat-induced transient block (HiTB) has not been quantified to date. Our objective was to use computer modeling to provide an initial estimate.
Methods and materials
We used previous experimental data together with the Arrhenius damage index (Ω) to fix the Ω values that delineate this zone: a lower limit of 0.1–0.4 and upper limit of 1.0 (lesion boundary). An RFCA computer model was used with different power-duration settings, catheter positions and electrode insertion depths, together with dispersion of the tissue’s electrical and thermal characteristics.
Results
The HiTB zone extends in depth to a minimum and maximum distance of 0.5 mm and 2 mm beyond the lesion limit, respectively, while its maximum width varies with the energy delivered, extending to a minimum of 0.6 mm and a maximum of 2.5 mm beyond the lesion, reaching 3.5 mm when high energy settings are used (25 W–20s, 500 J). The dispersion of the tissue’s thermal and electrical characteristics affects the size of the HiTB zone by ±0.3 mm in depth and ±0.5 mm in maximum width.
Conclusions
Our results suggest that the size of the zone of heat-induced transient block during RFCA could extend beyond the lesion limit by a maximum of 2 mm in depth and approximately 2.5 mm in width.
1. Introduction
Radiofrequency (RF) catheter ablation (RFCA) is a minimally invasive technique that has been in use for more than 40 years and has become the standard for the treatment of many types of cardiac arrhythmias. During RFCA, RF current is applied through an ablation electrode on the catheter tip. RF current provokes that the temperature raises above 50 °C in the myocardium around the electrode, resulting in cell death by denaturing both functional and structural proteins [Citation1]. Although it is generally accepted that tissue temperatures ≥50°C in RFCA cause irreversible thermal injury [Citation2], it is also known that hyperthermia-induced thermal damage is dependent not only on temperature but also on exposure time [Citation1]. Both the 50 °C isotherm and the damage index Ω have traditionally been used to estimate lesion size in computer modeling of RFCA [Citation3]. The goal of RFCA is to eliminate the focus of the arrhythmia, i.e., the group of cells involved in the irregular heartbeat. The arrhythmia will remain if these cells are not affected and the ablation will not be successful. In practice, various circumstances can cause the process to fail, e.g., the focus might be too far from the electrode and the lesion might not be deep enough to be destroyed.
Hyperthermia-induced alteration of the electrophysiological activity of cardiac cells is not always an irreversible process, and the alterations may be hence transitory in the case of moderate hyperthermia (<50 °C), e.g., reversible loss of excitability and transient block of electrical conduction [Citation4–6]. In fact, it is reasonable to assume that the cells around the lesion have been subjected to moderate RFCA heating that has caused transient changes in their electrophysiological activity (see ). In practice, the effects of ablation will be transient if the focus of the arrhythmia is subjected to moderate heating only.
Figure 1. RF-induced lesion groups those cardiac cells that have been subjected to a lethal thermal dose which has resulted in irreversible changes in their electrical activity (e.g., irreversible loss of excitability and block). Around, other cells have been subjected to sublethal (moderate) heating, with the consequent transient electrical change (e.g., reversible loss of excitability and block) [Citation4,Citation5]. Further on are those cells that have been subjected to much milder heating and have also temporarily increased their rate of electrical conduction [Citation4] and finally cells that have not undergone temperature-induced changes since their temperature hardly changed during RFCA.
![Figure 1. RF-induced lesion groups those cardiac cells that have been subjected to a lethal thermal dose which has resulted in irreversible changes in their electrical activity (e.g., irreversible loss of excitability and block). Around, other cells have been subjected to sublethal (moderate) heating, with the consequent transient electrical change (e.g., reversible loss of excitability and block) [Citation4,Citation5]. Further on are those cells that have been subjected to much milder heating and have also temporarily increased their rate of electrical conduction [Citation4] and finally cells that have not undergone temperature-induced changes since their temperature hardly changed during RFCA.](/cms/asset/fbeb452e-2a3c-4b37-a9b1-d1209898f6f6/ihyt_a_2163310_f0001_c.jpg)
While all the previous RFCA computer models focused on assessing the temperature distributions and predicting lesion size [Citation3], no previous computational or experimental study has so far focused on estimating the area of tissue subjected to sublethal heating capable of causing transient electrophysiological changes. Despite the limitations inherent in computational models, an initial estimation is certainly relevant scientific information with practical clinical implications. Our objective was therefore to use computer modeling to estimate how far the zone of heat-induced transient block extends beyond the lesion during RFCA.
2. Methods
2.1. Thermal injury model
Most RFCA studies that used computer modeling have estimated the lesion zone using either a lethal isotherm (50–55 °C) or a mathematical function to take the ‘thermal history’ at each point in the tissue into account [Citation3]. The most frequently used function models the tissue damage as an irreversible first-order chemical reaction, with the rate constant following the Arrhenius relationship [Citation7], specifically, with the thermal injury index Ω, which relates the number of undamaged cells C(0) present before heating to the number of undamaged cells remaining at time t, indicated by C(t) as follows:
(1)
(1)
This can be computed from the temperature T (in Kelvin) reached at each instant t (s) of the heating period (including the cooling period after the RF pulse):
(2)
(2)
where A is the frequency factor, Ea is the activation energy and R the universal gas constant (8.3143 J/mol·K). The values of A and Ea are particular for each tissue type and analyzed process. In previous modeling studies of RFCA, values of A = 7.39 × 1039 s−1 and Ea = 2.577 × 105 J/mol were used to model the irreversible thermal damage and to estimate hence the lesion size. It was checked that these values provide a Ω = 1 isoline more or less coincident with the 72 °C isotherm for 5 s heating [Citation8] and more or less coincident with the 55 °C isotherm after 60 s of heating [Citation2,Citation9], which is in line with the experimental data. Lesion size can hence be calculated by considering all the points in the tissue at which Ω ≥ 1. Using EquationEquation (1)
(1)
(1) , a value of Ω = 4.6 is equivalent to 99% cell death probability, while a value of Ω = 1 is equivalent to 63%. In the present study, we used the Arrhenius function to estimate the tissue area affected by a sublethal dose of thermal damage capable to cause a transient block in impulse conduction.
2.2. Modeling of the heat-induced transient block zone
As far as we know, only three experimental studies have measured temporary physiological changes in the myocardium associated with heating, including loss of excitability and conduction [Citation4–6]. To estimate the tissue volume subjected to sublethal heating capable to cause transient block during RFCA, we first searched for the values of Ω of a thermal history (i.e., combinations of temperature and time) compatible with reversible loss of excitability and conduction. If the tissue is subjected to a constant temperature (TH) for a specific time (tH), EquationEquation (2)(2)
(2) is greatly simplified and can be expressed as the following simple formula:
(3)
(3)
Of the three studies mentioned, only the first two kept the tissue at a constant temperature during heating, since they used a tissue bath to determine the effect of changing the superfusate temperature [Citation4,Citation5]. In the third, the tissue bath was kept perfused at 37 °C while heating was induced by gradually increasing RF power, which progressively raised the temperature throughout the entire heating period, thus making it impossible to use EquationEquation (3)(3)
(3) . The experimental setup in the first two studies took care to ensure that the tissue temperature rapidly reached the target value (TH): t1/2 (i.e., time required to reach half the value of TH) was only 0.9 ± 0.3 s in [Citation4] and ∼0.75 s in [Citation5], which indicates the appropriateness of EquationEquation (3)
(3)
(3) by assuming that the tissue sample was actually subjected to a constant temperature for a fixed time.
shows the electrophysiological changes seen in the experimental groups considered in the above-mentioned studies, as well as the Ω values derived from TH and tH for each electrophysiological change. The values shown are quite in tune with the value of Ω = 1 used in previous computational studies to delineate lesion size: above 0.93 excitability is irreversibly lost, according to Nath et al. [Citation4] and above 0.77 permanent block occurs according to Simmers et al. [Citation5]. In the tissue associated with sublethal heating, Ω above 0.1 there would be reversible loss of excitability (with immediate restoration of normal excitability after returning to 37 °C, according to Nath et al. [Citation4]), while transient block (absence of impulse conduction for ≤ 5 min) occurs above 0.4, according to Simmers et al. [Citation5]. These two values of 0.1 and 0.4 were assumed to be the lower thresholds to delineate the tissue zone associated with sublethal heating that could cause transient block. The upper threshold was the one due to the permanent block induced by the thermal lesion (i.e., Ω = 1.0). In short, the lesion size will be the one with Ω > 1.0, while the heat-induced transient block zone will be the one shown by 0.1–0.4 < Ω < 1.0 (see ). We also checked that the short time the tissue needed to reach the target temperature (TH) in the experiments [Citation4,Citation5] barely affected the Ω value (e.g., Ω decreases by only 0.02 when tH is reduced by a couple of seconds).
Table 1. Relation between electrophysiological changes observed in previous experimental studies and the in silico model parameters.
2.3. Model description
Three-dimensional computational models were built that included a round-tip 7 Fr 3.5 mm irrigated electrode placed on the myocardium and surrounded by blood. Three angles were considered between the tissue surface and catheter axis: perpendicular (90°), oblique (45°) and horizontal (0°). Due to the existence of one symmetry plane, the model only considered half of the real volume. We considered electrode insertion depths of 0.5 and 0.7 mm at the 90° angle, mimicking low and medium contact forces [Citation10]. The insertion depths for the other angles were adjusted to keep the contact surface constant, as it is the parameter most closely correlated with the lesion area per ablation [Citation11]. shows the model geometry with the catheter at 45°.
2.4. Ablation protocols
Computer simulations were conducted to model RF delivery at different settings (i.e., combinations of power and pulse duration). The first set of simulations considered three power-duration settings typically used in clinical practice: low power-moderate duration (LPMD, 25 W–20 s) [Citation12], high power-short duration (HPSD, 50 W–6 s) [Citation13] and very high power-very short duration (vHPvSD, 90 W–4 s) [Citation12]. The second set of simulations considered three power-duration settings with energy balanced at 360 J: moderate power-short duration (LPSD, 30 W–12s), high power-short duration (HPSD, 50 W–7.2 s) and very high power-very short duration (vHPvSD, 90 W–4 s) [Citation7]. The power value used in all the simulations was reduced by 20% as the model did not include the entire torso [Citation14]. The simulation data were obtained for up to 90 s after RF onset to examine the extra lesion growth due to thermal latency [Citation8].
2.5. Tissue properties and governing equations
Tissue properties were taken from the IT’IS Foundation database [Citation15], while the ablation catheter properties were taken from Pérez et al. [Citation16] (see ). The tissue electrical conductivity was assumed to increase by 1.5%/°C up to 99 °C, at which point it decreased by two orders of magnitude (from 99 to 101 °C) to simulate dehydration by vaporization. The latent heat associated with liquid-to-gas phase change was also considered, assuming the myocardium to have a 70% water content. The models were constructed using the Finite Element Method on ANSYS software (ANSYS, Canonsburg, PA, USA). Laplace’s Equation and Bioheat Equation were used to solve the electrical and thermal problem, respectively (see [Citation3] for further details).
Table 2. Characteristics of tissues and materials of each element used in the models [Citation15,Citation16].
A sensitivity analysis was conducted to take into account the dispersion in tissue characteristics, for which we chose the maximum and minimum myocardium values reported in the ITIS database (0.5 and 0.6 W/m·K for thermal conductivity, 1059 and 1143 kg/m3 for density, 3614 and 3724 J/kg·K for specific heat) [Citation15]. Myocardium and blood electrical conductivity were considered to have a ± 10% variation, which is of the same order as the dispersions of the rest of the characteristics, even though the IT’IS database does not include high frequency dispersion (500 kHz). Two very different cases were considered in terms of energy delivery for the sensitivity analysis: 25 W–20 s and 90 W–4 s (catheter at 90° and 0.5 mm insertion depth). Each model (half of the entire domain, see ) contained of ∼220,000 tetrahedral elements and ∼300,000 nodes. The outer dimensions (4 cm around the ablation electrode), mesh size (minimum of ∼90 µm around the electrode and maximum of ∼7 mm in the periphery) and the time step (ranging from 15 to 70 ms) were verified by means of convergence tests.
2.6. Boundary and initial conditions
The power value remained constant during the RF pulse, which meant that both current and voltage were suitably modulated as tissue impedance was gradually reduced. The dispersive electrode was simulated by conditions of 0 V on all the outer surfaces except the symmetry plane. Electrode irrigation was modeled by fixing a value of 25 °C in the electrode volume not embedded in the tissue and leaving the rest with a free temperature, to mimic a multi-hole electrode, since we assumed that irrigation occupied almost the entire electrode surface [Citation17]. The thermal problem was not solved in the blood, and thermal transfer coefficients for low blood flow condition (0.1 m/s) were used on the electrode-blood and myocardium-blood interfaces instead (3310 W/m2·K and 694 W/m2·K, respectively) to simulate ablation sites with low local blood flow, as in patients with chronic atrial fibrillation and dilated atria [Citation18]. A temperature of 37 °C was set at the outer contours of the tissue and the initial temperature was also 37 °C.
2.7. Statistics
This study used a physics-based mechanistic model in which the different factors (catheter orientation, power-duration setting and tissue properties) took different values to simulate a representative sample of what happens during RFCA ablation under varying conditions. The relationships between the variables were studied by simple regression using Excel. Coefficient of determination (R2) was reported to assess the goodness of fit, along with the p-value (P) to determine statistical significance (calculated by the two-tailed unpaired Student’s t test).
3. Results
shows the lesion sizes and of the heat-induced transient block zone with the typical power settings and 0.5 mm insertion depth. The lesion sizes and transient block zones were directly proportional to the energy delivered, with lesion depths of ∼3.2 mm at 50 W − 6s (300 J), ∼3.5 mm at 90 W − 4s (360 J) and ∼4.0 mm at 25 W − 20s (500 J). Overall, the transient block zone extended beyond the lesion limits by between 0.47 ± 0.08 mm and 1.91 ± 0.24 mm in depth, depending on the Ω threshold value considered (0.1 and 0.4, respectively) and between 0.64 ± 0.15 mm and 2.49 ± 0.55 mm in maximum width (see ). A strong linear correlation was found between the lesion depth and the depth of the transient block zone (R2 > 0.97, p < .001), while we did not find any statistical significance in the correlation between the maximum width of the lesion and that of the transient block zone.
Figure 3. Depth (A) and maximum width (B) of lesion and transient block zone for three catheter orientation (perpendicular 90°, oblique 45° and horizontal 0°) and different power-duration settings. Extension in depth (C) and maximum width (D) of the transient block zone beyond the lesion contour.

shows the lesion and transient block zone sizes with the same energy delivery (360 J) and 0.5 mm insertion depth. The lesions were quite similar regardless of the catheter angle and power setting, with mean values of 3.49 ± 0.08 mm in depth and 6.75 ± 0.36 mm in width. The sizes of the transient block zone were also similar at all the catheter angles and power settings, extending beyond the lesion limits by between 0.45 ± 0.02 mm and 1.86 ± 0.05 mm in depth and between 0.61 ± 0.06 mm and 2.36 ± 0.19 mm in maximum width (see ). We found a moderate negative linear correlation between the maximum lesion widths and that of the transient block zone (R2 > 0.65–0.72, p < .05). We did not find a statistically significant correlation between the lesion depths and the transient block zone. For illustrative purposes only, shows temperature progress at depths of 3 and 4.5 mm for three power-duration settings, at a 0.5 mm insertion depth and catheter at 90°. While the 3-mm depth is representative of an area subjected to lethal heating (located within the lesion limits), the 4.5-mm depth is the area exposed to sublethal heating capable to cause transient block.
Figure 4. Depth (A) and maximum width (B) of lesion and transient block zone for three catheter orientation (perpendicular 90°, oblique 45° and horizontal 0°) and power-duration settings delivering the same energy (360 J). Extension in depth (C) and maximum width (D) of the transient block zone beyond the lesion contour.

Figure 5. Temperature progress of the temperature at 3 and 4.5 mm depths during and after the RF pulse in case of perpendicular catheter (0° orientation) and three power-duration settings. Dashed line corresponds with the 50 °C isotherm (usually considered as the lethal threshold).

The results for the 0.7 mm insertion depth showed lesions slightly greater than at 0.5 mm, particularly at depths of ∼3.4 mm at 50 W − 6s (300 J), ∼3.7 mm at 90 W − 4s (360 J) and ∼4.2 mm at 25 W − 20s (500 J). However, the relationship between lesion and transient block zone sizes was almost identical for both insertion depths. The zone of transient block at 0.7 mm insertion and typical power-duration settings extended beyond the lesion limits by between 0.47 ± 0.07 mm and 1.92 ± 0.23 mm in depth (according to the Ω threshold value considered, 0.1 and 0.4, respectively) and between 0.64 ± 0.15 mm and 2.50 ± 0.54 mm in maximum width. The results for 360 J ablations and 0.7 mm insertion were almost identical: 0.45 ± 0.01 mm and 1.87 ± 0.04 mm in depth (according to the Ω threshold value considered, 0.1 and 0.4, respectively) and between 0.61 ± 0.06 mm and 2.37 ± 0.19 mm in maximum width. The dispersion in tissue thermal and electrical characteristics with the size of transient block zone had a moderate impact, since the maximum difference between using average and maximum/minimum values was less than ±0.3 mm in depth and ±0.5 mm in maximum width.
shows the lesion geometries (Ω = 1) and transient block zones (between Ω = 1 and Ω = 0.1–0.4). Apart from a slight asymmetry of the lesion geometries and the transient block zone with the catheter at 0°, the overall results show that the transient block zone extends between more than 0.5 and 2.0 mm in depth and lesion limits, respectively, regardless of catheter angle.
Figure 6. Distributions of the Ω value at 90 s for 25 W − 20s RFCA (0.5 mm insertion depth) at different catheter orientations: perpendicular (A), oblique (B) and parallel (C). Tissue points within the Ω = 1 isoline represent irreversible damaged zone (lesion), while the transient block zone would extend to at least Ω = 0.4 and at most to Ω = 0.1.

4. Discussion
4.1. Main findings
An RFCA model was used in this computer study to estimate how far the zone of heat-induced transient block extends beyond the lesion during RF catheter cardiac ablation. While the lesion zone is associated with irreversible loss of electrical impulse conduction characteristics, the transient block zone is associated with transient changes and therefore reversible. An assorted set of real features (such as catheter angle, power-duration setting, electrode insertion depth, dispersion in tissue characteristics) were incorporated into the model to generate applicable results to clinical practice. The study’s key findings are as follows:
An exact threshold value of the Arrhenius damage index Ω from heat-induced reversible changes cannot be inferred from the few experimental data available, but instead offer a relatively large range, between 0.1 and 0.4.
Even taking this relatively large range of Ω values into account, the computer results suggest that the transient block zone extends in depth to at least 0.5 mm and to a maximum of 2 mm outside the lesion limits.
The maximum width of the transient block zone seems to vary more with the energy delivered, while the results suggest that it extends beyond the lesion by a minimum of 0.6 mm and a maximum of 2.5 mm, reaching up to 3.5 mm at higher energy settings (25W − 20s, 500 J).
The dispersion in tissue thermal and electrical characteristics implies the scattering of the size of the transient block zone by ±0.3 mm in depth and ±0.5 mm in maximum width.
Although the existence of a zone peripheral to the RF-induced lesion and affected by transient electrophysiological changes is assumed in RF ablation, since it makes physical sense, there are no previous data that attempt to quantify its size. Our results thus offer this novel information, with important clinical implications for subendocardial ablation targets (i.e., in which the arrhythmic focus is deep), suggesting that when the arrhythmic focus is suspected to be 0.5–2 mm deeper than the lesion limit, a transitory disappearance of the arrhythmia can be expected. In the context of creating linear lesions (e.g., ablation of atrial fibrillation of flutter), our results suggest that two contiguous lesions separated by 1.2–5 mm (i.e., two-fold values of the maximum width of the transient block zone) could temporarily behave like a continuous lesion when in fact there is actually a gap between them.
These estimates are in agreement with the results reported by Simmers et al. [Citation19], who studied the effects of electrode–target distance and intracavitary blood flow on the radiofrequency (RF) power required to induce transient conduction block using a Langendorff-perfused canine model, a 7 Fr 4-mm standard catheter at 90° (5 g contact force) and 0.15 m/s blood flow. They found that 3 and 7 W were required to produce transient conduction block at 0 and 2 mm from the electrode, while it was not possible to induce block at 4 mm due to the RF power required being such that its delivery was terminated prematurely by a sudden impedance rise and coagulum formation at the catheter tip. Although neither the conditions nor the objective of the study by Simmers et al. [Citation19] were exactly like ours, their results suggest that the sublethal heating zone causing a transient block could reach 2 mm, but never 4 mm. It should be noted that our results suggest a range of 0.6–2.5 mm in terms of width and that Simmers et al. [Citation19] changed the distance between the RF catheter and the point at which transient block was assessed by moving the catheter over the tissue surface.
4.2. Experimental validation of the heat-induced transient block zone model
The ability of the current computational models to predict temperature maps and lesion size during RFCA has been extensively demonstrated, with errors of < 3–7 °C for tissue temperature at > 3 mm deep and 1–2 mm in terms of lesion size [Citation3]. We therefore focused on checking how our proposed transient block model (described in Section 2.2) is consistent with the results obtained in preclinical studies, especially those based on ex vivo models that measure the intramyocardial temperature progress at different depths below the electrode (e.g., [Citation20–22]). In theory, from this data we could determine the ‘thermal history’ associated with each point and so calculate the Ω value by EquationEquation (2)(2)
(2) . If this information is crossed with the lesion size reported in the same studies, it might be possible to identify the points in the tissue subjected to lethal heating capable to cause transient block.
As a simple illustration to emphasize how EquationEquation (2)(2)
(2) can compute the damage index Ω, we chose the temperature measurements obtained by Demazumder et al. [Citation20] at 3, 5 and 7 mm, in particular the four experimental groups that included blood flow around the electrode (Groups 2, 4, 6 and 8, see ). If we now simplify the temperature progress to a linear rise during the RF pulse (as has been reported) and a four times slower drop after the pulse, then we can apply EquationEquation (2)
(2)
(2) to estimate the Ω value in each group and depth (see ). Overall, we found that the Ω values obtained were consistent with the lesion sizes reported for each group.
Table 3. Summary of the results reported by Demazumder et al. [Citation20] for eight experimental groups and Ω values computed using the EquationEquation (2)(2)
(2) .
For instance, in Group 2, where lesion depth was 4.2 ± 0.3 mm, the Ω value at 3 mm deep was 0.938, i.e., almost 1, which represents the irreversibly damaged tissue. In contrast, at 5 mm the Ω value was 0.091, i.e., almost 0.1, which can be expected, since 5 mm is ∼1 mm beyond the lesion limit, i.e., the sublethal heating zone (note that our results suggest a range of 0.5–2.5 mm). At 7 mm, i.e., ∼3 mm beyond the lesion limit, Ω =0.021, which is much lower than 0.1 and thus is at a point outside the sublethal heating zone.
In Group 4 the Ω value at 3 mm was ∼9.1 (much higher than 1.0), which is consistent with the fact that the lesion limit is really 2 mm deeper (5 mm). The Ω value at 5 mm was 0.314, which in our model was in the sublethal heating zone and agrees with the fact that lesion depth is just around 5.0 mm (±1.0 mm). The Ω value at 7 mm was 0.126, which is associated with sublethal heating at a distance of ∼2 mm from the lesion limit. In the remaining cases (Groups 6 and 8) the Ω values were much higher than 1.0 at 3, 5 and 7 mm deep, since all these points were within the lesion boundary (8 mm).
In terms of clinical impact, we must recognize that the most important issue in the context of RFCA modeling is to predict the lesion size, i.e. the tissue volume delimited by >1. Although the main efforts have to be hence centered on optimizing the RF pulse parameters to increase the lesion size, we think that knowledge about how large the periablational zone responsible of transient block is worthy scientific information in the context of cardiac electrophysiology.
4.3. Limitations
The threshold Ω values used to determine the transient block zone were obtained from only two experimental studies and only 2 different heating durations (30 s [Citation5] and 60 s [Citation4]). One might wonder to what extent the Arrhenius function is valid for shorter exposure times, such as those associated with high-power short-duration (HPSD) ablation. In this regard, Simmers et al. [Citation5] evaluated the electrophysiological changes in transient block not only at 30 s but at 0, 10, 20 and 30 s heating durations and found a progressive drop in all the variables leading up to a transient block (including conduction velocity), which suggests that the combined effect of temperature and duration might be the key factors in the changes associated with reversible block and not just the threshold temperature. This was also confirmed by Cote et al. [Citation23], who found that time to transient block during the application of low-temperature short-duration RF pulses was significantly longer for 50 °C applications than 70 °C applications on the same site. The limitations inherent in the two experimental studies should also be considered: in vitro pig [Citation4] and a canine [Citation5] model, with only ventricular samples. In this regard, the great complexity of conducting this type of experimental study on human tissue under in vivo conditions should be recognized, in the same way that it would be very complex to experimentally assess the extension of the reversible block zone. Our results based on an in silico model certainly have many limitations, but they represent the first estimate made to date.
By assuming that the sublethal heating zone capable to cause transient block is the one with Ω values between 0.1–0.4 and 1.0, we are really using the Arrhenius thermal injury index Ω to model the transient electrophysiological changes induced by heating, not the irreversible thermal damage (lesion size), so that the percentage of living cells as expressed in EquationEquation (1)(1)
(1) is a meaningless parameter in our case. This limitation comes from the fact that specific values of A and Ea are not available for modeling the transient electrophysiological changes induced by moderate heating in the myocardium.
Our mathematical model was only based on the electrophysiological changes associated with a ‘progressive loss’ of electrical activity (excitability in Nath et al. [Citation4] and block in Simmers et al. [Citation5]). We did not consider the increasing conduction velocity found in the latter case [Citation5], when the tissue was subjected to very mild heating: from 38.5 to 45.4 °C for 30 s, which is equivalent to Ω values ranging from 0.01 to 0.12, according to EquationEquation (3)(3)
(3) . This assumption was made since we were only interested in estimating the size of the zone with RF-induced electrophysiological behavior similar to that of an ablated zone, which should itself manifest as a loss in excitability and block. It is also important to point out that the Arrhenius function only considered the RF-induced acute changes, and that hence late electrophysiological effects due for instance to microvascular injury [Citation24] might occur beyond the region of acute coagulation necrosis, causing the extension of the lesion.
Despite the fact that only a rounded-tip 7 Fr 3.5 mm catheter was considered, previous results have shown that both flat-tip and round-tip catheter lesions are quite similar [Citation7]. Neither is there any reason to assume that the relationship between lesion size and the transient block zone will be different when the electrode size varies slightly within the margins of the currently available catheters (7–8 Fr in diameter, 3–4 mm in length). Likewise, the fact that the model did not include other features (such as multi-parameter indexes − e.g., ablation index AI and lesion index LSI − and different blood flow rates) does not seem to affect the estimation of the relative size of the transient block zone in relation to lesion size.
Although the model assumed a homogeneous tissue instead of an arrangement based on different tissue layers (myocardium, epicardial fat, connective tissue, etc), it had already been found that this has little impact in terms of lesion depth [Citation7] and there is no physical reason to think that something different will happen with the transient block zone size. Finally, we did not consider extreme cases in which considerable energy is delivered, e.g., power ≥ 30 W or repetitive applications on the same point [Citation25], which we must recognize as being able to change the relative relationship between lesion size and the transient block zone.
5. Conclusions
Using a mathematical model based on the assumption that the sublethal heating zone capable to cause transient block is that of Arrhenius index values between 0.1 and 0.4, our simulation results suggest that the transient block zone might extend beyond the lesion limit by between 0.5 and 2 mm in depth and between 0.6 and 2.5 mm in maximum width. A ± 10% dispersion in the tissue characteristics would have a moderate impact on these dimensions (±0.3 mm in depth and ±0.5 mm in maximum width).
Disclosure statement
No potential conflict of interest was reported by the author(s).
Data availability statement
The data underlying this article will be shared on reasonable request to the corresponding author.
Additional information
Funding
References
- Viglianti BL, Dewhirst MW, Abraham JP, et al. Rationalization of thermal injury quantification methods: application to skin burns. Burns. 2014;40(5):896–902.
- Haines DE. Letter by Haines regarding article, “direct measurement of the lethal isotherm for radiofrequency ablation of myocardial tissue.” Circ Arrhythm Electrophysiol. 2011;4(5):e67; author reply e68.
- González-Suárez A, Pérez JJ, Irastorza RM, et al. Computer modeling of radiofrequency cardiac ablation: 30 years of bioengineering research. Comput Methods Programs Biomed. 2022;214:106546.
- Nath S, Lynch C 3rd, Whayne JG, et al. Cellular electrophysiological effects of hyperthermia on isolated Guinea pig papillary muscle. Implications for catheter ablation. Circulation. 1993;88(4 Pt 1):1826–1831.
- Simmers TA, De Bakker JM, Wittkampf FH, et al. Effects of heating on impulse propagation in superfused canine myocardium. J Am Coll Cardiol. 1995;25(6):1457–1464.
- Simmers TA, de Bakker JM, Wittkampf FH, et al. Effects of heating with radiofrequency power on myocardial impulse conduction: is radiofrequency ablation exclusively thermally mediated? J Cardiovasc Electrophysiol. 1996;7(3):243–247.
- Pérez JJ, D’Angelo R, González-Suárez A, et al. Low-energy (360 J) radiofrequency catheter ablation using moderate power - short duration: proof of concept based on in silico modeling. J Interv Card Electrophysiol. 2022. Epub ahead of print. DOI:10.1007/s10840-022-01292-z
- Irastorza RM, d‘Avila A, Berjano E. Thermal latency adds to lesion depth after application of high-power short-duration radiofrequency energy: results of a computer-modeling study. J Cardiovasc Electrophysiol. 2018;29(2):322–327.
- Pearce JA. Comparative analysis of mathematical models of cell death and thermal damage processes. Int J Hyperthermia. 2013;29(4):262–280.
- Pérez JJ, Nadal E, Berjano E, et al. Computer modeling of radiofrequency cardiac ablation including heartbeat-induced electrode displacement. Comput Biol Med. 2022;144:105346.
- Masnok K, Watanabe N. Catheter contact area strongly correlates with lesion area in radiofrequency cardiac ablation: an ex vivo porcine heart study. J Interv Card Electrophysiol. 2022;63(3):561–572.
- Kotadia ID, Williams SE, O'Neill M. High-power, short-duration radiofrequency ablation for the treatment of AF. Arrhythm Electrophysiol Rev. 2020;8(4):265–272.
- Barbhaiya CR, Kogan EV, Jankelson L, et al. Esophageal temperature dynamics during high-power short-duration posterior wall ablation. Heart Rhythm. 2020;17(5 Pt A):721–727.
- Irastorza RM, Gonzalez-Suarez A, Pérez JJ, et al. Differences in applied electrical power between full thorax models and limited-domain models for RF cardiac ablation. Int J Hyperthermia. 2020;37(1):677–687.
- Hasgall PA, Di Gennaro F, Baumgartner C, et al. IT’IS database for thermal and electromagnetic parameters of biological tissues, Version 4.1. 2022. DOI:10.13099/VIP21000-04-1
- Pérez JJ, Ewertowska E, Berjano E. Computer modeling for radiofrequency bipolar ablation inside ducts and vessels: relation between pullback speed and impedance progress. Lasers Surg Med. 2020;52(9):897–906.
- González-Suárez A, Pérez JJ, Berjano E. Should fluid dynamics be included in computer models of RF cardiac ablation by irrigated-tip electrodes? Biomed Eng Online. 2018;17(1):43.
- Nakagawa H, Ikeda A, Sharma T, et al. Comparison of In vivo tissue temperature profile and lesion geometry for radiofrequency ablation with high power-short duration and moderate power-moderate duration: effects of thermal latency and contact force on lesion formation. Circ Arrhythm Electrophysiol. 2021;14(7):e009899.
- Simmers TA, de Bakker JM, Coronel R, et al. Effects of intracavitary blood flow and electrode-target distance on radiofrequency power required for transient conduction block in a Langendorff-perfused canine model. J Am Coll Cardiol. 1998;31(1):231–235.
- Demazumder D, Mirotznik MS, Schwartzman D. Comparison of irrigated electrode designs for radiofrequency ablation of myocardium. J Interv Card Electrophysiol. 2001;5(4):391–400.
- Demazumder D, Mirotznik MS, Schwartzman D. Biophysics of radiofrequency ablation using an irrigated electrode. J Interv Card Electrophysiol. 2001;5(4):377–389.
- Wong WS, VanderBrink BA, Riley RE, et al. Effect of saline irrigation flow rate on temperature profile during cooled radiofrequency ablation. J Interv Card Electrophysiol. 2000;4(1):321–326.
- Cote JM, Epstein MR, Triedman JK, et al. Low-temperature mapping predicts site of successful ablation while minimizing myocardial damage. Circulation. 1996;94(3):253–257.
- Nath S, Whayne JG, Kaul S, et al. Effects of radiofrequency catheter ablation on regional myocardial blood flow. Possible mechanism for late electrophysiological outcome. Circulation. 1994;89(6):2667–2672.
- Sánchez-Muñoz EJ, Berjano E, González-Suárez A. Computer simulations of consecutive radiofrequency pulses applied at the same point during cardiac catheter ablation: implications for lesion size and risk of overheating. Comput Methods Programs Biomed. 2022;220:106817.