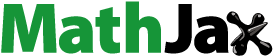
ABSTRACT
Rapid determination of the dissolution behavior of a compound fertilizer is difficult in agricultural industry. In this work, we describe a simple and rapid method for quantifying the disintegration properties of compound fertilizer for the indirect evaluation of their dissolution. The effects of screen mesh, disintegration time, temperature, fertilizer-water ratio, stacking mode and particle size on fertilizer disintegration test were studied. Under standardized conditions, the maximum and minimum relative standard deviation (RSD) were 12.7% and 0.9%, respectively. The mean deviations of the measured data were > 4%, standard deviations > 4.6%, and interquartile ranges > 6.7%. The feasibility, accuracy, error tolerance, and consistency of this lift disintegration method meet industrial requirements and can be utilized for the rapid determination of the solubility of compound fertilizers.
Introduction
Compound fertilizer is a common type of high-concentration fertilizer containing two or more nutrients, such as nitrogen, phosphorus, and potassium. It significantly increases crop yields, leading to economic and social benefits (Hunter and Schultz Citation1995; Jarosiewicz and Tomaszewska Citation2003; Group ns Citation2013; Guo et al. Citation2021; Yubo Citation2021). The solubility and dissolution rates of water-soluble fertilizers are particularly important because their incomplete and slow dissolution can lead to lower efficiency, increased time consumption, and nutrient loss. The dissolution behavior of fertilizers is determined by the quality of the raw material, technical preparation route, and production conditions (da Silva RC et al. Citation2018; Sigtryggsson et al. Citation2020; Çelikbilek Ersundu et al. Citation2022). Rapid determination of product solubility is crucial for quality control and efficient industrial production.
Compound fertilizers are commonly composed of hybrid solid granules with particle sizes of 1–5 mm. They are formed by mixing several salts, either molten or solid powders, followed by granulation using condensation or pressure (Ivanov et al. Citation1983; Taran et al. Citation2009). Thus, the size, uniformity, compactness, and composition of the granules affect the solubility of the compound fertilizer and cause complex interactions when dissolving large granules in water (Ma et al. Citation2021). However, fertilizer dissolution is difficult to measure accurately, requiring the use of specialized instruments, such as a titrator or a spectrophotometer, whose results are influenced by the presence of non-solvated particles (Yang and Zhou Citation2008; Song et al. Citation2014). Furthermore, complete dissolution requires a long period of time, limiting our ability to rapidly determine the dissolution kinetics and dissolvability for production purposes (Bajpai and Mishra Citation2008, K. G.; Karapetyan et al. Citation2005; Kogan and Karapetyan Citation2018; Olanipekun Citation2002). Thus, it is important to develop a straightforward, rapid, and accurate method to measure the dissolution properties of compound fertilizers.
The dissolution process of compound fertilizers has an intermediate disintegration step, where large granules break into small pieces that disperse in water to form a uniform suspension. Next, the suspended fertilizer dissolves to form a transparent solution. The uniform dispersion of small fertilizer particles leads to a constant concentration increase throughout the solution, indirectly indicating the dissolution performance of an arbitrary fertilizer. Thus, disintegration kinetics can be used to evaluate the dissolution behavior of a compound fertilizer, circumventing the difficult and time-consuming process of complete dissolution analysis. However, this requires the development of a suitable method for evaluating the disintegration of compound fertilizers.
Currently, there are no reported methods to determine compound fertilizer disintegration. However, analogous methods of rock disintegration for geotechnical mechanics and tablet disintegration in the pharmaceutical industry can be used as references (Hiroyuki et al. Citation2010). The disintegration kinetics can be determined via rotational and lifting disintegration using the centrifugal force and lift motion resistance, respectively (Dick et al. Citation1994; Ankara et al. Citation2015; Zhang et al. Citation2020). The most common parameters measured to evaluate disintegration include shape, mass, and volume.
The disintegration of compound fertilizers is more similar to that of tablets than to that of rocks because of their dissolution behavior after disintegration. Therefore, the development of an analogous determination method for compound fertilizers should be based on current tablet disintegration analysis. However, the different dissolution behavior, shape, and composition influences disintegration, thus requiring changes to the operation mode and parameters of the lifting disintegration method. Its applicable range and usable operational parameters for compound fertilizer analysis must be determined prior to use.
In this work, we propose a method called the reformed lift disintegration (RLD) to evaluate the disintegration degree of compound fertilizers based on the mass loss after disintegration. This method is a quick and simple detection method to evaluate the problem of fertilizer dissolution. The operation mode and parameters of this method were optimized, and its accuracy, sensitivity, and validity were evaluated.
Material and methods
Materials
Ten samples of different types of compound fertilizers were evaluated for disintegration performance: 17-5-17 (Sample 1: 17 wt.% N, 5 wt.% P2O5, 17 wt.% K2O, Xuzhou Huachang Fertilizer Industry Co. Ltd. China), 15-15-15 (Sample 2, 15 wt.% N, 15 wt.% P2O5, and 15 wt.% K2O, Huaqiang Biological Technology Co. Ltd. China), 18-18-18 (Sample 3, 18 wt.% N, 18 wt.% P2O5, 18 wt.% K2O, Shandong Dafeng Biology Co. Ltd. China), 19-19-19 (Sample 4, 19 wt.% N, 19 wt.% P2O5, 19 wt.% K2O, Stanley China), 13-6-21 (Sample 5, 13 wt.% N, 6 wt.% P2O5, 21 wt.% K2O, Yara International Norway), 15-5-23 (Sample 6, 15 wt.% N, 5 wt.% P2O5, 23 wt.% K2O, Jiuhe Co. Ltd. China), 15-7-23 (Sample 7, 15 wt.% N, 7 wt.% P2O5, 23 wt.% K2O, Jiuhe Co. Ltd. China), 15-15-15 type (Sample 8, 15 wt.% N, 15 wt.% P2O5, 15 wt.% K2O, Jiuhe Co. Ltd. China), 16-16-16 type (Sample 9, 16 wt.% N, 16 wt.% P2O5, 16 wt.% K2O, Jiuhe Co. Ltd. China), and 17-17-17 type (Sample 10, 16 wt.% N, 16 wt.% P2O5, 16 wt.% K2O, Jiuhe Co. Ltd. China).
Characterization
Scanning electron microscopy (SEM; JEM-F200, JEOL, Japan) were performed to study the surface of the fertilizers, respectively. The particle sizes of all samples were measured using the Vernier Scale (Huang et al. Citation2021). The compressive strength was measured using a mechanical strength meter (ZQJ-II-100N, Dalian Intelligent Test Machine Factory, China). Using sample 1–5 for method construction, and sample 6–10 for method verification.
Instrument optimization
Stainless-steel filters with mesh number ranging from 20 to 80 were evaluated using these criteria. The fertilizer samples were ground and sieved using meshes of different sizes. Approximately 1.00 g of each different fertilizer powder between specific meshes was dispersed in 300 mL of water at 25 °C with stirring at 660 rpm. The lower, middle, and upper layers of the suspension were sampled for microscopic examination.
Disintegration
All samples were dried to a constant weight before analysis. Approximately 5.00 g of dry fertilizer (M1) in an 80 °C oven were added to a container with stainless steel mesh of selected mesh size. The container was supported on a lifting metal bracket and immersed into a beaker containing 300 mL of water at a constant temperature of 25 ± 1°C. The container with residual fertilizer was removed after a measured time period 60 s and dried at 80°C in an oven to a constant weight for 4 hours. Finally, the mass of the residual fertilizer was measured (M2).
The disintegration degree (D) was calculated using EquationEquation 1.(1)
(1)
The operational parameters of the disintegration analysis, including time, temperature, fertilizer/water ratio, stacking mode, and fertilizer particle size were optimized for accurate testing.
Dissolution analysis
An adapted lift disintegration method was used to measure the dissolution. 5.00 g of dry fertilizer were combined with 300 mL of deionized water at 25 °C in a disintegration apparatus. The phosphate content of 1 µL solution removed every 30 s was determined using the molybdenum blue method with UV-vis diffuse reflectance spectroscopy (PerkinElmer, Lambda750s) (Salem Citation1996; Bogrekci and Lee Citation2006; Francisco et al. Citation2011; Ganesh et al. Citation2012).
The dissolution degree (D’) was calculated using EquationEquation 2.(2)
(2)
where and
are the initial and final phosphate concentrations, respectively.
Data analysis
Each fertilizer was tested in five groups and its measured disintegration degree recorded as . The arithmetic means (X), the Median (Me), mean deviations (MD), standard deviations (S), variances (σ), relative standard deviations (RSD), interquartile ranges (IQR), and full range (R) were calculated for our test data according to EquationEquations 3
(3)
(3) –Equation9
(9)
(9) :
where is the number of data sets,
is the maximum data point,
is the minimum data point,
is the upper quartile, and
is the lower quartile.
Results
The morphology and composition of 10 fertilizer samples were determined using SEM and XRD. The surface and internal cross-sections of these samples observed by SEM () were significantly different. Sample 1 is accumulation, sample 2 and 10 are loose, sample 3, 4, 7, 8, 9 are dense, sample 5 and 6 are porous, and sample. The XRD of sample 1-10 are shown in .
Additional information on the fertilizer samples, including the diameter and mechanical strength, is summarized in Supplementary Material 1 and Figure S1.
The particle appearance and size distribution are shown in Figures S2–S6. As shown in and Figures S7–S11, all particles that passed through a sieve with a mesh number > 60 maintained a stable suspension in water after 30 s of stirring with a uniform particle size distribution in the three liquid layers. In contrast, particles obtained from sieves with a mesh number of < 60 deposited quickly with a significant particle size difference in the different layers.
The weight loss of 5.00 g fertilizer in 300 mLwater for various times was measured by this method. As shown in ), it can be seen that the mass loss degree of samples 1 and 3 tend to maintain almost stable after 60 s, suggesting the stopping of disintegration. The mass loss degree of samples 2, 4 and 5 still continue increasing beyond 60 s.
Figure 4. Disintegration degree of samples 1–5 with the time (a), temperature (b) and the fertilizer to water ratio (c).

Then conducting the disintegration degree measurement under different temperature, 15°C, 25°C, and 35 °C, to determine the temperature effect, respectively. As shown in ), it can be observed that the effect of temperature on disintegration for same fertilizer sample at 60 s is negligible, all slightly increasing when water temperature raising from 15 °C to 35 °C. Sample 1 increased from 78.2% to 98.5%, sample 2 from 56.0% to 58.0%, sample 3 from 3.3% to 4.6%, and sample 4 from 28.9% to 37.6%, sample 5 increased from 35.7% to 37.6%.
Changing the fertilizer-water ratio of 1:300, 1:100, 1:60, 1:42.86, 1:30, and 1:20 when fixing fertilizer dosage is 5.00 g, and conducting the disintegration experiment. As shown in ), it can be determined that the higher the fertilizer-water ratio, the slower the disintegration degree. When the fertilizer-water ratio is more than 1:60, the disintegration degree tends to be constant.
The fertilizer stacking mode can also affect the disintegration process by decreasing the mass transfer of water. Thus, three stacking modes, single-, double-, and triple-layer stacking, were tested (). Using optimized operational conditions, we observed that the disintegration degree decreased almost linearly with the number of layers in all the samples ().
The particle size of fertilizer should be an important factor greatly influencing the disintegration performance of a fertilizer product. Grinding the fertilizer, and sieving to obtain small granules with different size and evaluating their disintegration properties. The results in ) show that the disintegration degree increases with the decrease of particle size. The smaller the fertilizer particle size, the faster the disintegration degree, but the smaller the discrimination between different fertilizers.
For each fertilizers, randomly sampling about 5 g particles as 1 group, conducting 30 times disintegration testing, and carrying out the repeatability and precision analysis based on 30 batch results. The resulting histograms of each fertilizer are shown in . It can be observed that the disintegration degree of sample 1 ranged from 96% to 99%, of sample 2 from 49% to 66%, of sample 3 from 4% to 7%, of sample 4 from 28.5% to 44.5%, and of sample 5 from 31% to 48%.
The RLD method was used to evaluate the disintegration properties of five different fertilizer samples obtained from Jiuhe Co. Ltd. Their disintegration degree was compared to the corresponding dissolution degree. As shown in ), the dmax of determined phosphate content in water emerges after 30 s. Sample 10 tended to reach dissolution equilibrium in 90 s, then sample 6 and sample 7 in 120 s, finally sample 7 and sample 9 in 360 s. Calculating the dissolving degree according to the phosphate degree change in arbitrary time interval, and plotting in ), it can be observed that sample 10 has the fastest dissolving degree, with about 96.3% of sample 10 dissolved into water in 60 s. The dissolved rates of other samples are 89.8% of sample 6, 85.8% of sample 8, 79.8% of sample 9, and 75.0% of sample 7.
Discussion
Characterization of fertilizer samples
The surface and internal cross-sections, main components, diameter size, and mechanical strength of samples 1–5 were significantly different. The distinct physical properties of our samples suggest that they are representative of the fertilizer class and are thus suitable for the development of a universal method for evaluating the disintegration and dissolution behaviors of compound fertilizers.
Instrument optimization
The screen mesh is a key component in lift disintegration analysis, determining the particle size that passes through the mesh after disintegration, and thus the amount of residual solids. An adequate mesh size must guarantee that all particles that pass through it remain suspended in water, meeting the definition of disintegration used when evaluating fertilizer dissolution.
All particles that passed through a sieve with a mesh number > 60 maintained a stable suspension in water after 30 s of stirring. In contrast, particles obtained from sieves with a mesh number of < 60 deposited quickly with a significant particle size difference in the different layers. Thus, meshes with a number of > 60 guarantee uniform small particles that disperse in water and dissolve rapidly. A 60-mesh net was used for all subsequent tests in this study.
A 60-mesh net was folded as shown in , forming a 4 × 4 × 4 cm cubic cage that can contain approximately 10 g of fertilizer granules and a 4 cm upper hanger with a 28 cm folded rope to suspend the cage. The disintegration performance was determined by submerging the cage containing a fertilizer sample into water and moving it up and down at a frequency of 1 s and an amplitude of 1 cm.
The operation mode and parameter optimization
After deciding the key component, all operation parameters of disintegration testing including time, temperature, ratio of fertilizer to water, and stacking mode as well as fertilizer particle size, should be investigated for constructing the most accurate, sensitive and effective testing program.
First, we measured the mass loss of 5.00 g fertilizer samples using our optimized lift disintegration analysis with time. To determine the difference between the maximum mass losses of different fertilizers, the disintegration degree was plotted against time and fitted with a suitable function.
Using the third-order function (show in Figure S12a, Table S1), the average t value for different fertilizers was 76.11 s, and the maximum (dmax) and minimum (dmin) differences were 90.6% and 0.4%, respectively. Using the fourth-order function (show in Figure S12b, Table S2, the average t value was 59.05 s, and dmax and dmin were 93.6% and 4.2%, respectively. The fourth-order function better estimated the disintegration degree.
The time for distinguishing the disintegration degree difference of different fertilizers was set as 60 s, corresponding to dmax and dmin of 91.0% and 1.5%, respectively, as fitted by the third-order function, and the dmax and dmin of 93.5% and 4.0%, respectively, as fitted by the fourth-order function.
The disintegration degree was measured at different temperatures (15 °C, 25 °C, and 35 °C) (), revealing its negligible impact on disintegration.
Next, disintegration was evaluated at variable fertilizer/water ratios (1:300, 1:100, 1:60, 1:42.86, 1:30, and 1:20). When the fertilizer-water ratio is more than 1:60, the disintegration degree tends to be constant.
Fitting the obtained data using a third – order function (show in Table S3) allowed us to determine the average fertilizer-/water ratio as 1:57.80, with a dmax of 94.2% and a dmin of 0.5%. Furthermore, fitting using a fourth-order function (show in Table S4) led to an average fertilizer/water ratio of 1:69.28, with a dmax of 92.3% and a dmin of 1.7%. Because the difference between average fertilizer/water ratios was not significant, and the difference between different fertilizers was noticeable in both experiments, a 1:60 ratio was considered advantageous because it reduced water consumption by 17%. This ratio was within the acceptable range for practical applications (1:100 to 1:33). At this optimal ratio, dmax and dmin of the third-order function were 94.2% and 0.7%, respectively, and dmax and dmin of the fourth-order function were 93.5% and 0.9%, respectively.
As shown in (c), it can be determined that the higher the fertilizer-water ratio, the slower the disintegration degree. When the fertilizer-water ratio is more than 1:60, the disintegration degree tends to be constant.
Fitting the obtained data by the third- and fourth-order functions, it can be observed that the average value of the fertilizer-water ratio is 1:57.80 with dmax of 94.2%, and dmin of 0.5%. However, when using the fourth-order function, the average fertilizer-water ratio value is 1:69.28, with dmax of 92.3% and t dmin of 1.7%. The difference between 1:60 and 1:70 is not big, and the difference between different fertilizers is also very obvious when the ratio is 1:60. When the ratio of fertilizer to water is 1:60, the experimental water consumption can be reduced by 17%. Moreover, considering a fact that the fertilizer-water ratio in practical application should be controlled between 1:100 and 1:33, thereby the employed fertilizer-water ratio can be selected as 1:60. Under this condition, dmax of the third-order function was 94.2% with dmin of 0.7%, and dmax of the fourth-order function was 93.5% with dmin of 0.9%, respectively.
The fertilizer stacking mode can also affect the disintegration process by decreasing the mass transfer of water. The maximum discrimination was achieved using single-layer testing. The relationship between the disintegration degrees of the different samples remained the same regardless of the stacking mode. Still, single-layer stacking is considered optimal because of lower fertilizer use and higher disintegration degree differences.
Fertilizer stacking mode could affect the disintegration process of fertilizer particles by impacting the mass transfer of water in measurement. All five samples show a similar trend, obtaining the maximum discrimination when the fertilizers are piled in single layer. The disintegrating degree sequence of different samples keeps the same at the different stacking modes, but the single layer stacking mode is the best applicable due to low fertilizer amount and great disintegration degree difference.
These results confirm the excellent error tolerance of this process. Despite significant differences in sample mass when testing different numbers of layers, the disintegration degree trend remained the same. However, the difference between samples decreased with higher mass, likely due to the reduced contact between water and stacked fertilizer particles and the trapping of small particles before reaching the mesh.
The effect of particle size of the fertilizer on its disintegration was evaluated. The disintegration degree of all samples except 1 increased linearly with smaller particle sizes. Thus, the differences between fertilizers were less noticeable for smaller particle sizes.
Our results show that the particle size significantly affects disintegration because small particles have a larger surface area and improved contact with water. Still, large particles are required for the practical application of this test because most commercial fertilizers have a standardized particle size of over 1 mm for simpler packaging and storage. Thus, our proposed method for evaluating the disintegration of fertilizers must use their original particle size to obtain results that are closer to practical use.
Precision test
Conducting the precision testing of RLD method. The data were analyzed according to formula (2)-(8).The details are shown in the Table S5-S9. The results are as .
Table 1. Statistical analysis of testing result of sample 1–5.
The dmax between and Me of the 30 batches was only 1.1% of all samples, indicating that the data was concentrated and the average values reflect the disintegration degree. The
was less than 4% and the maximum
was 4.6%, indicating a dmin between the data and
that confirms that the average value is equally representative. The relatively high R value (16.3%) reflected high data dispersion, greatly influenced by extreme values. However, the small
of 7.5% indicated a small dispersion degree. Finally, an RSD lower than 12.7% was representative of the excellent precision of this RLD method.
Application of the RLD method
Samples 6–10 contained monoammonium phosphate as the main component. Because this compound has the slowest dissolution degree among common compound fertilizers, the dissolution behavior of these samples was evaluated based on the concentration of phosphate in water.
The same samples were tested using the RLD method to determine their disintegration rates after 60 s and compared with their dissolution rates. The two sets of data were consistent, with the corresponding rates of dissolution and disintegration. The maximum discrimination of the disintegration rate occurred between samples 10 and 6. The highest disintegration rate was observed for sample 10 (89.5%), and the lowest for sample 6 (75.6%). Their difference was 13.9%, which is larger than that obtained directly from the phosphate concentration (6.5%). The minimum discrimination occurred between samples 6 and 8 (3.7%), and was similar to their dissolution differences (4.0%). These results show that disintegration can be used to evaluate the dissolution rate of compound fertilizers, using a simpler and faster method and obtaining more differentiated results.
This confirms that determining the disintegration rate using the RLD method can effectively reflect the solubility differences of different fertilizers without introducing complicated analytical processes or expensive equipment. This method is suitable for the rapid evaluation of fertilizer solubility and has potential for application in other fields.
Conclusion
In this study, we optimized a simple and rapid method for determining the disintegration rate of compound fertilizers from their mass loss, indirectly evaluating the dissolution performance of the fertilizer. This method is verified by the dissolution method. The time, fertilizer/water ratio, temperature, particle size, and stacking mode influenced the obtained results. And the feasibility, accuracy, error tolerance, and consistency of this lift disintegration method met industry requirements.
Under optimized conditions, testing 30 sets of data, the maximum and minimum relative standard deviation (RSD) were 12.7% and 0.9%, respectively. The measured data were less dispersed, with mean deviations of < 4%, standard deviations of < 4.6%, and interquartile ranges of < 6.7%.
This mass disintegration degree testing method provides a simple but accurate method for rapidly determining the dissolution properties of compound fertilizers, with potential industrial applications.
Supplemental Material
Download MS Word (7.3 MB)Acknowledgments
We are thankful for the financial support provided by the National Natural Science Foundation of China Project (No. 21978181 and No. 22178229). The authors acknowledge the support from the Center of Engineering Experimental Teaching, School of Chemical Engineering, Sichuan University, for SEM analysis, and the Institute of New Energy and Low-Carbon Technology, Sichuan University, for XRD analysis. Moreover, we would like to thank Jiuhe Co. Ltd for its support.
Disclosure statement
The authors declare that they have no known competing financial interests or personal relationships that could have appeared to influence the work reported in this paper.
Supplementary material
Supplemental data for this article can be accessed online at https://doi.org/10.1080/03650340.2023.2295359.
Additional information
Funding
References
- Ankara H., Kandemir S.Y., Çiçek F. 2015. Compression of slake durability index (SDI) values of sphere and rounded marl samples. Procedia Earth Planet Sci. 15:93–98. doi: 10.1016/j.proeps.2015.08.024.
- Bajpai J., Mishra S. 2008. Dynamics of controlled release of potassium nitrate from a highly swelling binary polymeric blend of alginate and carboxymethyl cellulose. J Applied Polymer Sci. 106(2):961–972. doi: 10.1002/app.26703.
- Bogrekci I., Lee W.S. 2006. Effects of soil moisture content on absorbance spectra of sandy soils in sensing phosphorus concentrations using UV-VIS-NIR spectroscopy. Trans ASABE. 49(4):1175–1180. doi: 10.13031/2013.21717.
- Çelikbilek Ersundu M., Kuzu B., Ersundu A.E. 2022. Structural properties and dissolution behavior of new generation controlled release phosphate glass fertilizers. J Non Cryst Solids. 576:121239. doi: 10.1016/j.jnoncrysol.2021.121239.
- da Silva RC, Baird R., Degryse F., McLaughlin M.J. 2018. Slow and fast-release boron sources in potash fertilizers: spatial variability, nutrient dissolution and plant uptake. Soil Science Soc Amer J. 82(6):1437–1448. doi: 10.2136/sssaj2018.02.0065.
- Dick J.C., Shakoor A., Wells N. 1994. A geological approach toward developing a mudrock-durability classification system. Can Geotech J. 31(1):17–27. doi: 10.1139/t94-003.
- Francisco P.P., Noelia C., Inmaculada C., Marta C., Sandra G., Isela L., Carlos B. 2011. Directly suspended droplet microextraction in combination with microvolume UV–vis spectrophotometry for determination of phosphate. Talanta. 85(2):1100–1104. doi: 10.1016/j.talanta.2011.05.032.
- Ganesh S., Khan F., Ahmed M.K., Velavendan P., Pandey N.K., Kamachi Mudali U. 2012. Spectrophotometric determination of trace amounts of phosphate in water and soil. Water Sci Technol. 66(12):2653–2658. doi: 10.2166/wst.2012.468.
- Group ns. 2013. Production of compound fertilizers. nitrogen + syngas. 38–39.
- Guo J., Fan J., Zhang F., Yan S., Zheng J., Wu Y., Li J., Wang Y., Sun X., Liu X., et al. 2021. Blending urea and slow-release nitrogen fertilizer increases dryland maize yield and nitrogen use efficiency while mitigating ammonia volatilization. Sci Total Environ. 790:148058. doi: 10.1016/j.scitotenv.2021.148058.
- Hiroyuki M., Tadashi M., Ryo K. 2010. Development of a New disintegration method for orally disintegrating tablets. Chem Pharm Bull (Tokyo). 58(7):885–890. doi: 10.1248/cpb.58.885.
- Huang C.N., Wu P. 2021. Segmentation of urea melt marbles and application of one-shot segmentation in batch production of large urea granules. ACS Sustainable Chem Eng. 9(43):14430–14442. doi: 10.1021/acssuschemeng.1c04409.
- Hunter M.D., Schultz J.C. 1995. Fertilization mitigates chemical induction and herbivore res. Ecology. 76(4):1226–1232. doi: 10.1043/0012-9658(1995)076[1226:[FMCIAH]2.0.CO.
- Ivanov M.E., Lindin V.M., Malkin B.I., Kucheryavyi V.I., Gorlovskii D.M. 1983. Ways of increasing the size of the urea granules in granulation towers. Khimicheskaya Promyshlennost 4:241–243.
- Jarosiewicz A., Tomaszewska M. 2003. Controlled-release NPK fertilizer encapsulated by polymeric membranes. J Agric Food Chem. 51(2):413–417. doi: 10.1021/jf020800o.
- Karapetyan K.G., Senichenkov V.A., Zenin G.S., Ryabova M.N. 2005. Kinetics of dissolution of glassy fertilizers. Russ J Appl Chem. 78(9):1383–1385. doi: 10.1007/s11167-005-0522-6.
- Kogan V.E., Karapetyan K.G. 2018. Theoretical analysis of the influence of particle size distribution on the kinetics of the dissolution of phosphorus-containing vitreous fertilizers. Glass Phys Chem. 44(5):394–397. doi: 10.1134/S1087659618050085.
- Li Yubo 2021. Current Status and Future Development of Main Chemical Fertilizers. China Chem Rep 32(10):11–16.
- Ma X., Li A., Wang X., Kong D., Qiu S., He Y., Yin Y., Liu Y., Liu R. 2021. Spectral method for predicting nitrogen, phosphorus, and potassium in small amount of compound fertilizer. Zhongguo Jiguang/Chin J Lasers. doi: 10.3788/CJL202148.2311003.
- Olanipekun E.O. 2002. The dissolution kinetics of single superphosphate fertilizer(Article). Pak J Sci Ind Res. 318–320. doi: 10.1248/cpb.58.885.
- Salem F.B. 1996. Determination of phosphate in water samples. Rev Anal Chem. 15(3):225–236. doi: 10.1515/REVAC.1996.15.3.225.
- Sigtryggsson C., Hamnér K., Kirchmann H. 2020. Laboratory studies on dissolution of nitrogen fertilizers by humidity and precipitation. Agricultural Env Letters. 5(1):e20016. doi: 10.1002/ael2.20016.
- Song L., Zhang H., Ni X., Wu L., Liu B., Yu L., Wang Q., Wu Y. 2014. Quantitative analysis of contents in compound fertilizer and application research using near infrared reflectance spectroscopy. Guang pu xue yu guang pu fen xi = guang pu. 73–77. doi: 10.3964/j.issn.1000-0593(2014)01-0073-05.
- Taran A.L., Dolgalev E.V., Taran Y.A. 2009. Testing of adequacy of mathematical description for lime ammonium nitrate granulation in towers. Theor Found Chem Eng. 43(4):457–463. doi: 10.1134/S0040579509040058.
- Yang Q.Q., Zhou C.H. 2008. The test of potassium oxide on mixed and compound fertilizer by flame atomic absorption spectrometry. Dangdai Huagong 36(2):209–211.
- Zhang Z.T., Gao W.H., Zeng C.F. 2020. Evolution of the disintegration breakage of red-bed soft rock using a logistic regression model. Transp Geotech. 24:100382. doi: 10.1016/j.trgeo.2020.100382.