Abstract
Underpinning almost every Paleozoic paleogeographic reconstruction and tectonic model of the Lachlan Orogen is the interpretation of the structural geology related to crustal collision and amalgamation. Bounding the eastern margin of the Lachlan Orogen is a north-trending corridor of Cambrian–Ordovician turbidites that in northeast Victoria are recognised as the Pinnak Sandstone or previously known as the Mallacoota Beds. This sequence contains an accretionary prism related early S* foliation, syn-sedimentary dykes, slump structures, early faults or décollements and early quartz veins. These indicate anisotropic compaction by fluid extraction in a shallow marine turbidite sequence that underwent localised deformation (D1), which can be attributed to the Benambran Orogeny (ca 450 Ma). There was a change in the rheological properties during the ensuing late Silurian D2 Bindian Orogeny (ca 420 Ma). This event produced north–south-trending upright, east-verging folds (F2) with a marked partitioning of strain between the different sedimentary units. Folds plunge south at ∼30°, are tight to isoclinal, with local collapsed hinges and limb thrusts. This D2 deformation along the paleo-Pacific margin involved dextral transpression and a southerly propagation of the deformation front. Superimposed on these structures is an east–west paleostress pattern and two episodes of faulting (D3 and D4). In the first, brittle strike-slip faults were accompanied by ductile folding related to east–west shortening during the Middle–Late Devonian Tabberabberan Orogeny (ca 385–370 Ma). These were accompanied by the emplacement of the Sandpatch Point Granite. The second episode (D4) of strike-slip faulting reactivated many of the earlier faults during further east–west shortening and was related to the Carboniferous Kanimblan Orogeny (ca 354–343 Ma). This is best seen close to the granite contact where the strong crystallised granite reinforced the contact metamorphosed greywacke sequence, inhibiting ductile deformation so that faults deformed in a brittle fashion.
KEY POINTS
Eastern Australia was at a convergent plate boundary where a Middle–Late Ordovician turbiditic sequence was accreted to an accretionary prism.
The Pinnak Sandstone contains a weak phyllosilicate alignment (S*), D1 faults and folds from localised deformation in the accretionary prism.
The Silurian Bindian Orogeny produced upright east-verging, south-plunging F2 folds. Brittle faults overprint the Bindian tectonic features and are accompanied by minor folding, marking the transition into Middle Devonian and Carboniferous deformation and intrusive events.
The kinematics and geometries associated with these deformation events reflect the changing tectonic regime along the paleo-Pacific margin of eastern Australia during east–west compression.
Introduction
Dynamic Paleozoic plate-tectonic activity in the Terra Australis Orogen involved the supercontinent Gondwana (). At this time, the Cambrian–Ordovician paleogeography in eastern Australia involved a protracted period of continental growth owing to accretion along a north-trending corridor (Cawood, Citation2005; Moore et al., Citation2015, Citation2016; Squire et al., Citation2006). This led to the development of a large depositional system adjacent to the eastern margin to the paleo-Australian craton (). Following deposition, these sediments experienced an extensive history of overlapping extensional and compressional tectonic cycles throughout the Paleozoic (Cawood, Citation2005; Glen et al., Citation2009; Gray & Foster, Citation2004; Wilson et al., Citation2020). In recent years, a new geodynamic model for this part of southeastern Australia has been developed invoking the presence of an orocline in the Victorian Paleozoic sequences. Oroclines are features of orogenic belts that exhibit regional-scale bends, and oroclinal bending resulted from rotation of an accretionary wedge section around a (near)-vertical axis. The Lachlan Orocline is focused on the underlying Proterozoic Selwyn Block in central Victoria and rotates around the northern apex of the pre-existing microcontinent (Cayley, Citation2011; Cayley et al., Citation2002; Moore et al., Citation2016; Moresi et al., Citation2014; Musgrave, Citation2015). The Cambrian and Ordovician sedimentary rocks of central Victoria were folded during the Benambran Orogeny (ca 440 Ma; Wilson et al., Citation2020) in a deformation that pre-dates initiation of the Lachlan Orocline.
Figure 1. (a) Location of eastern Australia in the Terra Australis Orogen in a portion of East Gondwana (after Cawood, Citation2005) and the depositional system (blue) prior to the Silurian deformation along the paleo-Pacific margin of eastern Australia. (b) Schematic reconstruction after the convergence between East and West Gondwana between ca 490 and 460 Ma and the location of major features associated with evolution of the Gondwana super-fan system that produced the deposition along the paleo-Pacific margin (modified after Squire et al., Citation2006). The super-fan system was a result of high rates of erosion in the Transgondwanan Supermountains, which were established from ca 650 and 515 Ma. The present-day areal extent of the Bengal and Nicobar fan systems (Fergusson & Coney, Citation1992) adjacent to the Sumatra subduction zone is shown for scale. (c) Early Paleozoic evolution of southeastern Australia (after Moresi et al., Citation2014). This outlines the development of an orocline in response to the presence of VanDieland and the retreat of a subduction zone. (d) Structural zones of southeastern Lachlan Orogen (after VandenBerg et al., Citation2000).
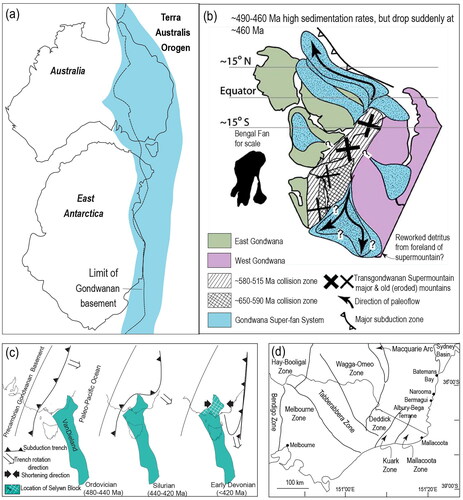
Deformation in eastern Victoria is seen to be part of this larger system, which is coupled to a clockwise rotation generated by local congestion in a subduction zone in the east of a pre-existing microcontinent such as VanDieland (Cayley, Citation2011; Moore et al., Citation2016; Moresi et al., Citation2014); this includes the Selwyn Block (). As the Selwyn Block migrated northwards and westwards with respect to the Gondwanan margin (Moresi et al., Citation2014), the subduction zone transitioned into asymmetric rollback north of the region of the initial collision at the Ordovician–Silurian boundary (). As the orocline developed, large volumes of sediments were still shed into an ocean basin from a Transgondwanan Supermountain system (). From the Late Ordovician (ca 450 Ma) to the Late Devonian (ca 370 Ma), the Selwyn Block in central Victoria slowly collided with Gondwana and was responsible for establishing the dominant Bindian (ca 420 Ma) and Tabberabberan (ca 385–370 Ma) aged deformation, which relate to plate boundary processes operating east of the Mallacoota Zone ().
Figure 2. Ordovician to Carboniferous stratigraphic column and link to tectonic events for the southeast Lachlan Orogen. The Pinnak Sandstone ranges from Early to Middle Ordovician and extends into Bendoc Group and Late Ordovician at Mallacoota (VandenBerg & Stewart, Citation1992). The period 460–445 Ma corresponds to the age range or stages of graptolite evolution (Gi = Gisbornian; Ea = Eastonian; Bo = Bolindian) identified in sediments deposited in the Late Ordovician and overlaps with the Benambran orogenic events identified in central Victoria (Wilson et al., Citation2020) and the D1 events described in this paper. The Devonian stratigraphy is after Young (Citation2007), where the Merimbula Group is composed of the Twofold Bay Formation (dark blue), Bellbird Creek Formation (broken horizontal lines) and Worange Point Formation (pink). The middle Carboniferous mega-kinging is the event identified by Powell (Citation1984).
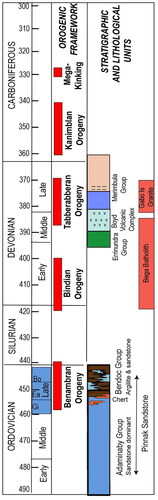
The Middle to Upper Ordovician sedimentary successions from Batemans Bay (New South Wales) into East Gippsland (Victoria) are part of this early Paleozoic convergent plate boundary sequence along >4000 km of the paleo-Pacific margin of East Gondwana (Cawood, Citation2005; Glen et al., Citation2004, Citation2009). The geodynamic setting of this section of the Lachlan Orogen has also been interpreted, predominantly from two-dimensional sections, as: (1) subduction mélange tectonics (Jenkins et al., Citation1982), (2) abyssal-plain deposits formed as outer-arc slope sediments prograding eastwards onto oceanic crust (Powell, Citation1983), (3) the Narooma Accretionary Complex (Glen et al., Citation2004; Miller & Gray, Citation1996) with an upper part of oceanic crust and foreland basin accreted to the Gondwana margin in the early Silurian, (4) a wide regional blanket of turbidites deposited on a passive margin (VandenBerg & Stewart, Citation1992), or (5) a thrusted passive margin accumulation (Packham & Hubble, Citation2016).
These interpretations described some of the geometries observed in two-dimensional model interpretations that were related to pure shear deformation components. Observations of present plate motions reveal that convergent plate boundaries are commonly transpressional in the sense of Harland (Citation1971), with the relative plate velocity oblique to the plate boundaries (Beck, Citation1983; Fitch, Citation1972; McCaffrey, Citation1992; Simoes & Avouac, Citation2006). Obliquity between the convergence direction, the paleostress field and the bounding blocks results in a triclinic transpression (Jones et al., Citation2004; Sanderson & Marchini, Citation1984). Consequently, the vorticity vector is neither parallel nor perpendicular to the vertical stretch of the pure shear component (Jiang et al., Citation2001). This produces an oblique stretch, so folds and lineations vary in plunge from horizontal to vertical depending on the simple shear to pure shear ratio, the total strain and the obliquity of the boundary simple shear component (Robin & Cruden, Citation1994). In this situation, the boundary of eastern Australia would have undergone simultaneous compression and a strike-slip transcurrent movement.
There is now some consensus that structures in this part of the Lachlan Orogen are the inevitable products of the tectonic events that assembled an accretionary terrane during the early Paleozoic (Resources Victoria, Citation2024, March 4). This is based on the interpretation of seismic reflection data (line 18GA_SL3 2D PSTM) acquired across the Deddick, Kuark and Mallacoota zones of the eastern margin of the Lachlan Orogen (). The seismic section illustrates a ∼40 km-thick portion of the paleo-Pacific convergence zone where tectonic processes have horizontally juxtaposed thrust slices of allochthonous Cambrian ocean floor and Cambrian–Ordovician marine sediments against a thick crustal body, the Macquarie Arc (Fergusson & Colquhoun, Citation2018). Interpreting the seismic section leads to the three main questions to be addressed in this article. (1) Did the paleo-Pacific margin undergo pure shear or simple shear-dominated transpression during deformation? (2) How were the accretionary prism sediments deformed? (3) What influence did the rheological conditions have on the structures developed in the sedimentary sequence?
Figure 3. Portion of the deep seismic reflections acquired in 2018 across the Kuark and Mallacoota zones and boundary with the Deddick Zone along the McLauchlan Creek Fault Zone (modified from data and interpretation in Resources Victoria, Citation2024, March 4) and also showing locations of the major faults mentioned in the text. The location of the seismic line is shown in . (a) Migrated seismic section along the western portion of line 2 and the total data acquired along line 3 (186 A-SL3 2D PSTM). PSTM = PreStack Time Migration. Display shows the vertical scale equal to the horizontal, assuming a crustal velocity of 6000 ms−1, TWT = two-way time. (b) Interpreted cross-section (modified after Resources Victoria, Citation2024, March 4). Blue (Oada) represent Ordovician sequences; mauve (Ox) = possible Macquarie igneous province; green (Cxv) = Cambrian mafic igneous rocks, the base of which must be faults; Cxv stipple = possible Cambrian–Ordovician; magenta = early Silurian – middle Devonian plutons; yellow = Devonian basin-fill (Dme) and volcaniclastic rocks. Legend data modified from Resources Victoria, Citation2024, March 4. The thick imbricated package of Cambrian and Ordovician units related to the accretionary prism abut the MacLauchlan Creek Fault Zone and are juxtaposed against units in the west related to the Macquarie Arc. The inferred subduction zone remnant may be a portion of the Tabberabberan or younger subduction zone.
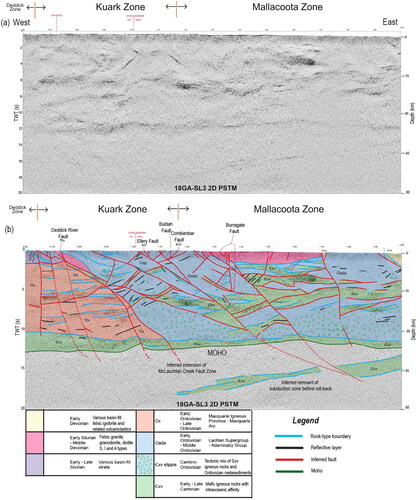
Structural analysis of small areas within this eastern segment of the Lachlan Orogen have delineated at least two main generations of folding (Etheridge et al., Citation1973; Hobbs, Citation1962; Miller & Gray, Citation1997; Williams, Citation1971; Wilson, Citation1968; Wilson et al., Citation1982). These folds have traditionally been recognised as F1 and F2 in each of these studies. However, they have also been observed to overprint an earlier localised foliation, identified as S* (Powell & Rickard, Citation1985; Wilson & de Hedouville, Citation1985). S* is a strong orientation of phyllosilicates that sub-parallels bedding. In this paper, it is suggested that S* was produced during an early D1 imbrication in the accretionary prism, and many of the later upright, north–south-trending non-cylindrical folds are a D2 event (). A later generation of folding (F3) recognised by the overprinting of earlier structures may have a similar style. However, as pointed out by Williams (Citation1970) in similar Ordovician rocks, north along strike at Bermagui (New South Wales), different fold generations may overlap in style and orientation, and this may lead to a misinterpretation of both geometry and history. In the Mallacoota region, it will be shown that numerous faults and shears overprint earlier localised accretionary prism structures (D1), rotate earlier F1 and F2 folds and are associated with later folds (F3 and F4) and a crenulation cleavage, particularly in the mudstone-rich rocks. This article focuses on the Mallacoota area, a region where overprinting relationships between structural events are clearer and provides field arguments allowing a discussion of several increments of the kinematic evolution of the Lachlan Orogen ().
Table 1. Structural history and deformation style, focussing on Mallacoota with references to other regional examples.
Adjacent to the Mallacoota township () and in areas to the southwest, there are extensive coastal outcrops (∼20–50 m wide) of an easily accessible sequence dominated by graded sandstones without significant metamorphic modification. These have previously been referred to as the Mallacoota Beds (Douglas, Citation1974), but are now incorporated into what is redefined as the Pinnak Sandstone (VandenBerg et al., Citation1992, Citation2000). Structural features in this rock sequence from Mallacoota to Bruces Creek () have been described by Wilson et al. (Citation1982). In the current paper additional structural features will be described in detail, from the north to the south, to further characterise the geometries in this part of the Lachlan Orogen. There is a complex relationship between folding and thrusting, with pre- and syn-cleavage thrusts (Wilson & de Hedouville, Citation1985), and upright folds (F2) with a marked cleavage (S2) with later post-cleavage high-angle strike- or oblique-slip faults.
Figure 4. Location of the structures immediately south of Mallacoota township (adapted from Wilson et al., Citation1982). (a) Location map of coastal exposures between Bastion Point and Bruces Creek. (b) A composite section in the region of Geology Point. The eastern portion is dominated by upward facing sandstones with intercalated shale containing south plunging F2 folds. The western portion is dominated by an F1 fold in laminated chert and mudstone, which is faulted and folded by F2 folds with steep axial surfaces (S2). The junction between the two sequences is faulted, and an undeformed porphyry intrusion is located in the northeastern chert outcrop. (c) A section adjacent to the car park at Quarry Beach in a laminated chert-pelagic mudstone succession with a landward or west F1 fold-vergence. (d) Refolded F2 folds in the turbidite sequence with an excellent crenulation cleavage associated with F3 folds. (e) Map of coastal exposures south of Bruces Creek and two cross-sections (A–A′ and B–B′) illustrating a slump unit in the turbidites folded by the south-plunging F2 folds. SL, sea-level. (f) Paleocurrent directions restored to pre-folding orientations in this section of coastal exposures. All stereonet plots of structural data in this and subsequent figures are equal-area lower-hemisphere projections.
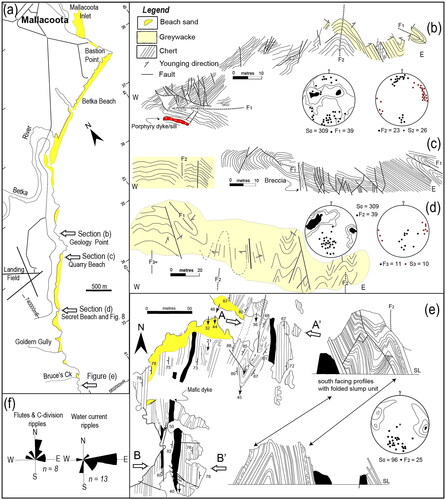
Geological setting
The Lachlan Orogen in eastern Victoria and southeastern New South Wales preserves remnants of Lower to Upper Ordovician quartz-rich turbiditic greywacke in an accretionary setting outboard of the Ordovician Macquarie Arc (Fergusson & Colquhoun, Citation2018). Sedimentation occurred along the eastern Gondwanan margin (), in an ocean basin that was consumed by a retreating subduction zone (). This easterly portion of the Lachlan Orogen hosts a collage of lithologies () with complex and commonly contested structural relationships (Glen et al., Citation2004; Packham & Hubble, Citation2016). These include Cambrian and Ordovician rocks of the Wagonga and Adaminaby groups in New South Wales (Glen et al., Citation2004; Wilson, Citation1968) and the Pinnak Sandstone in Victoria (). The earliest deformation fabric recognised in this coastal belt consists of an early syn-sedimentary bedding-parallel alignment of phyllosilicates identified as S* (Powell & Rickard, Citation1985; Wilson & de Hedouville, Citation1985), and isoclinal recumbent folds (Williams, Citation1970, Citation1971). In this paper, such structures will be included in an early Benambran D1 deformation event (). The main post-sedimentation deformation (D2) is constrained to the early Silurian by the presence of Ordovician sedimentary rocks at Narooma (Glen et al., Citation2004; Stewart & Glen, Citation1991) and by the onset of a later massive east–west shortening in the late Silurian Bindian Orogeny (; ).
Other evidence of an early D1 Benambran syn-sedimentary deformation () can be found at Batemans Bay (Fergusson & Frikken, Citation2003) in the Pinnak Sandstone in the Eastern Tabberabbera Zone (Willman et al., Citation2005) and at Narooma (Glen et al., Citation2004; Miller & Gray, Citation1996; Wilson, Citation1968). Chaotic rock-units have been described as broken formations with scaly fabrics (Willman et al., Citation2005), or boulder beds, which at Narooma host clasts and blocks of chert and amygdaloidal basalt containing secondary fillings of sub-greenschist facies sericite, chlorite, clinozoisite and calcite (Wilson, Citation1968). These sequences are similar to those described as mélange zones and are interpreted as parts of accretionary complexes above subsiding tectonic plates (Fisher & Byrne, Citation1987; Morgan et al., Citation2007). In the Narooma anticlinorium, there are also early bedding-parallel fabrics, isoclinal recumbent folds and décollements particularly between the Bogolo Formation and the main body of chert (Miller & Gray, Citation1996, Citation1997).
The Silurian–Devonian granites of the Bega Batholith were emplaced between 420 and 390 Ma (Collins et al., Citation2006; Stirling et al., Citation2022) generally as sheet-like bodies (). The granites intrude Ordovician sedimentary rocks and are non-conformable beneath the Middle to Late Devonian Boyd Volcanic Complex (Fergusson et al., Citation1979). The Bega Batholith has formed by multiple and protracted episodes of magma emplacement as a result of tectonic switching during successive phases of tectonic activity along the paleo-Pacific margin (Stirling et al., Citation2022 and references therein). The intrusion of these Silurian–Middle Devonian granites was accompanied by a variable deformation, and low-pressure contact metamorphism of the enclosing sedimentary rocks to form biotite, andalusite and knotted cordierite schists in metapelites. Margins of individual plutons may be foliated as in the Cann Valley granites and are crosscut by northeast-trending mylonite zones with dextral movement senses (Begg et al., Citation1987). Other granites, such as at Cape Conran, have undergone heterogeneous penetrative deformation with a steep west-dipping foliation (S) that has a northerly strike (Burg & Wilson, Citation1988).
In some zones, the granites are displaced along a set of oblique shear planes (C), which dip steeply (<60°) to the west with a steep down-dip stretching lineation. S^C fabrics indicate a reverse sense of shear. Burg and Wilson (Citation1988) concluded that the main foliation (S) in the more deformed granites and aplitic dykes formed concurrently with late folds in the enclosing Ordovician metasedimentary rocks. This coincided with eastward shearing causing major regional folding in the sediments under east–west compression in the Tabberabberan Orogeny ().
The Middle–Late Devonian Tabberabberan Orogeny caused regional compression and uplift, transforming the paleogeography into a subaerial one with numerous basins that appear to have originated in extension or transtension (VandenBerg et al., Citation2000). Along the south coast of New South Wales, there is a north–south-trending belt of Late Devonian bimodal rhyolitic and basaltic volcanic rocks and associated sedimentary rocks known as the Boyd Volcanic Complex (Fergusson et al., Citation1979). These rhyolitic and basaltic successions and accompanying porphyritic dykes are also intruded by the comagmatic and contemporaneous A-type Gabo Island Granite (Fergusson et al., Citation1979).
The Boyd Volcanic Complex is unconformably overlain by the shallow marine and fluvial clastic deposits of the Merimbula Group (). Next to the Combienbar Fault in eastern Victoria is a Lower Devonian shallow marine sedimentary basin containing a volcaniclastic sequence and limestone known as the Errinundra Group (). Unconformably overlying these is a weakly deformed Upper Devonian fluviatile sequence, and the basal section of Combyingbar Formation contains Famennian fish fossils (Douglas, Citation1974; VandenBerg et al., Citation1992; Young, Citation2007). Early Carboniferous deformation during the Kanimblan Orogeny has resulted in these Lower to Upper Devonian strata being folded into broad synclinal and anticlinal structures ().
Figure 5. Simplified geological map (after Mallacoota 1:250 000 Sheet SJ55-8) showing the location of seismic line 3. (a) Map and cross-sections (b and c) highlighting main rock units and structural trends identified in the Kuark and Mallacoota zones. PCF, Pleasant Creek Fault and together with Combienbar Fault is the location of the inferred boundary between the Kuark (in west) and Mallacoota zones (in east).
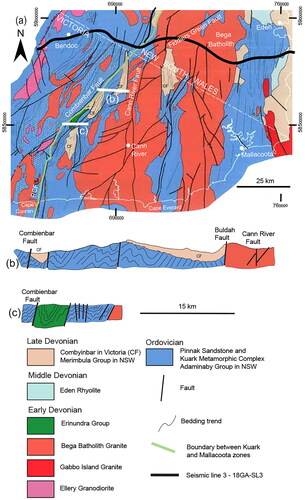
Major regional northeast-trending faults with dextral movements transect both the Ordovician sequences and the Early Devonian granites; these include the mylonite-bearing Fiddlers Creek Fault (Begg et al., Citation1987), which links up with the Burragate Fault in New South Wales (Collins et al., Citation2006; Fergusson et al., Citation1979). In the hanging wall of the northeast-trending Combienbar Fault (), the upper greenschist facies metamorphic rocks are intruded by variably foliated granites. These lie northwest of the Combienbar Fault and form part of the Kuark Zone (). The mesoscopic (F2) folds of the Kuark Zone deform a prominent earlier S1 schistosity, are sub-horizontal and plunge towards 040° or 220°. The F2 folds are locally deformed by later north- or northwest-trending kinks that have retrograde chlorites in their axial surfaces and may be related to the Carboniferous mega-kinking described by Powell (Citation1984).
The Middle to Upper Ordovician Pinnak Sandstone at Mallacoota typically crops out in a 20 km coastal section and is a thick metagreywacke–metasiltstone–shale sequence with minor intercalated cherts (Fenton et al., Citation1982; Kakuwa & Webb, Citation2007). The metamorphic grade varies between sub-greenschist facies (100–150 °C), particularly in the chert sequences, and greenschist facies. Greenschist facies conditions prevailed during the S2 cleavage development in areas affected by the D2 upright folding event (Wilson et al., Citation1982). Near Mallacoota, graptolite faunas in the rocks indicate they were deposited at the Gisbornian–Eastonian boundary (de Hedouville & Wilson, Citation1983). Further north, near Batemans Bay (Jenkins et al., Citation1982), the Wagonga Group have yielded slightly younger graptolites with ages spanning the Eastonian–Bolindian boundary (). At Cape Everard (Fry & Wilson, Citation1982), where there is a similar sequence, the conodonts suggest a Late Ordovician age, similar to conodonts identified at Mallacoota (Supplemental data, Figures S1 and S2). The cherts at Seal Creek are late Darriwilian to earliest Gisbornian, i.e. latest Middle Ordovician to earliest Late Ordovician (Kakuwa & Webb, Citation2007).
Lithology, stratigraphy and provenance of the Pinnak Sandstone
Seven lithostratigraphic entities () have been identified by Fenton and Wilson (Citation1985) in a sequence interpreted as turbidites (Fenton et al., Citation1982) and based on the subdivisions of Mutti and Ricci-Lucchi (Citation1972) and Walker (Citation1978). These are listed below:
Table 2. Characteristics and interpretation of the principal lithofacies from Mallacoota.
Massive sandstone as amalgamated beds representing turbidite channels.
Volumetrically dominant alternating mudstone and greywacke. The greywackes (Davidson, Citation2021) or flysch deposits (Bouma, Citation1962) are dominated by graded sandstones, varying from 0.1–3 m thick, beginning with a basal Bouma A-division. Bottom structures include flame structures and flute marks.
Beds with planar laminations or Bouma divisions BE or DE in places with episodic reworking by storm related wave currents.
Interbedded thin sandstone and mudstone, which are commonly rippled. There are differences in current directions between superimposed current rippling and underlying Bouma C-division ripples ().
These are alternating radiolarian-rich chert, silicified mudstone together with black hemipelagic muds and intercalations of chert nodules that contain graptolites and abundant conodonts (de Hedouville, Citation1984).
Slump units at scales from centimetres (with very common interlayered folds) to hundreds of metres, without the early cleavage (S*), but folded by the subsequent tectonic events.
Since the Pinnak Sandstone is composed of turbidites and cherts, many of these sediments have been assigned to a deep-water origin (Cas, Citation1983; Gray & Foster, Citation2004; Powell, Citation1983; VandenBerg & Stewart, Citation1992). These workers have used paleocurrent flow patterns identified in basal flute marks and Bouma C-division ripples to determine the sedimentation flow patterns and provenance. Despite the limited paleocurrent data, flute marks in the Pinnak Sandstone are predominantly north–south (), in sequences between Mallacoota and Cape Everard (Fry & Wilson, Citation1982). There are also traction structures, starved ripple pavements and hummocky cross-stratification, which suggests a much shallower origin for these marine sediments (Fenton & Wilson, Citation1985). The starved ripples and their dominant easterly paleocurrent direction, vs the north–south flute marks, suggest that some ripples formed during traction conditions and were subsequently buried in mud when turbidity currents waned. In contrast, a deep-marine pelagic environment has been interpreted for cherts at Seal Creek (Kakuwa & Webb, Citation2007).
Provenance studies of this portion of the Gondwanan super-fan system () have shown little variation in composition and source region over the eastern extent of the Ordovician turbidites (Fergusson & Fanning, Citation2002). This is despite a variety of dramatic changes in global environmental conditions and terrestrial weathering processes in the Transgondwanan Supermountains (Squire et al., Citation2006). The large volumes of Cambro-Ordovician quartz-rich turbidites deposited along the paleo-Pacific margin of Gondwana (i.e. Australia, Antarctica, and New Zealand) are generally considered to represent the product of convergent-margin orogenesis (Coney et al., Citation1990). This was particularly so during the Cambrian and Early Ordovician in central Victoria (Squire & Wilson, Citation2005), where there were deep-water facies (Cas, Citation1983). In eastern Victoria, the major influx of quartz-rich detritus occurred with ages of ca 516–514 Ma; detrital zircons as young as 473–453 Ma occur in the Pinnak Sandstone, from the Genoa River west of Mallacoota (Fergusson & Fanning, Citation2002). The Transgondwanan Supermountains at this stage were close to the equator (). The mountains would have been rapidly eroded between 490–460 Ma and accompanied by high depositional rates along the paleo-Pacific margin (Squire et al., Citation2006). A major change occurred in sedimentary facies and depositional environments from the Late Ordovician (ca 460 Ma) into the Silurian along the eastern margin of Gondwana where sedimentation was dominated by more argillites and chert () and evidence of shallower water depths (Fenton & Wilson, Citation1985). During the Middle to Late Devonian, these sediments were cratonised (Moresi et al., Citation2014), and continental facies sedimentation began in restricted sedimentary basins.
Syn-sedimentary structures and early quartz veins
The primary planar structures are bedding and a diagenetic compaction foliation, particularly in the mudstone and siltstone sequences (Fenton & Wilson, Citation1985; Wilson & de Hedouville, Citation1985). The earliest phase of deformation was syn-sedimentary and was accompanied by local bedding inversion; this could be worked out by noting the sedimentary way-up structures, particularly cross-stratification and/or graded bedding. All lithologies show considerable lateral thickness variations because of significant later deformation, making it impossible to accurately determine the original stratigraphic thicknesses. Pre-lithification deformation features include fold slumps (), rip-up clasts, convoluted bedding, ball-and-pillow structures () and dewatering conduits like sandstone dykes (), which were injected into adjacent mudstones or sandstones either up or down the stratigraphy.
Figure 6. Representative photos showing syn-sedimentary and D1 deformation features. (a, b) Ball-and-pillow structures that pre-date deformation at Secret Beach. Scale = 25 cm ruler and is the same in both images. The arrow in (b) indicates a quartz-filled bedding-parallel fault. (c) Syn-sedimentary sandstone dyke (D) in black shale unit north of Shipwreck Creek. (d) Folded early quartz veins, indicated by white arrows parallel to the S2 cleavage. (e) Folded and boudinaged early quartz veins (indicated by white lines I and II) overprinted by later sub-planar extensional veins. (f) Reactivated low-angle normal faults marking shearing in a chert sequence. Hammer scale = 34 cm.
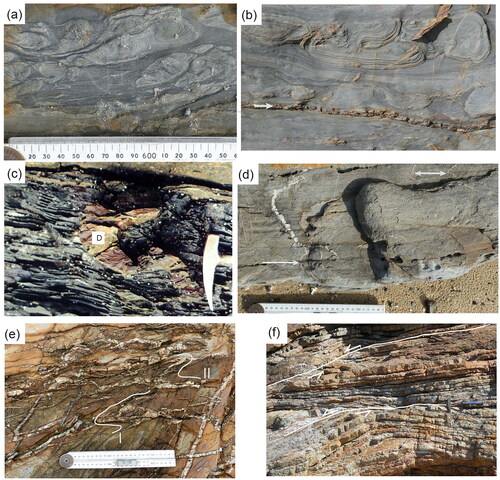
The primary structures also include a diagenetic foliation (S*) in mudstone and some siltstone units, and may be accompanied by a scaly fabric, which is at a low angle to bedding and transects crossbedding (Wilson & de Hedouville, Citation1985). In contrast, bedding that was folded during sedimentation into slumps, at scales from centimetres to hundreds of metres, is devoid of the S* cleavage. These structures and the sandstone dykes are syn-sedimentary because they are systematically deformed by younger D2 structures (). There are also numerous isolated interlayered F1 folds or isolated hinges associated with detachment or décollement surfaces that contain evidence of extensive fluid migration especially on the boundaries of chert units (Wilson & de Hedouville, Citation1985). These surfaces are generally marked by coatings of fibrous quartz with fine mica intergrowths. An early bedding-parallel phyllosilicate fabric S* also accompanies these highly deformed zones, which Wilson and de Hedouville (Citation1985) attributed to an early deformation or shearing environment, rather than to an inherited sedimentary feature. The S* surface is folded in the hinges of the first regionally developed meso- and macro-scopic F2 folds.
Quartz veins occur along and across bedding, along cleavage, as en échelon arrays and at boudin necks in competent sandy units. They both pre- and post-date the formation of the S* cleavage (Wilson & de Hedouville, Citation1985). The quartz veins that pre-date the S2 cleavage are variably folded, boudinaged, brecciated and crosscut by the S2 cleavage and by later quartz veins (). The origin of S* is debatable, with Powell and Rickard (Citation1985) suggesting a composite origin during fold hinge migration. On the other hand, Wilson and de Hedouville (Citation1985) related it to the early shear displacement in a deforming imbricate wedge in an accretionary environment. Normal faults, particularly in the cherts, show flat/ramp geometries with antithetic block rollovers (), with clear signs of inversion.
Early quartz veins also occur along bedding planes () with a marked increase where turbidite sequences are in contact with major chert units (). These quartz-rich chert contacts are also the focus of later deformation and may be brecciated, overprinted by steep-plunging fibrous quartz associated with D2 faults and low-angle quartz slickenfibres associated with later strike- or oblique-slip faults. Quartz veins may also have filled radial fractures and formed arrays normal to fold hinge lines with still later en échelon vein sets with the acute bisector normal to the fold hinge. The occurrence of early quartz veins and S* suggests that solution transfer and volume reduction may have been accommodated in an early accretionary phase and continued during the later tectonic events. Marques et al. (Citation2010) described comparable features, which they suggest initiated when the sequence was unconsolidated and composed of fluid-saturated sediments.
Figure 7. Folding and faulting in chert sequence north of Geology Point. (a, b) South facing section in the chert sequence containing isoclinal F1 folds many of which are refolded by F2 with a north–south-trending sub-vertical S2 axial surface. This chert sequence is thrust eastwards over a package of sandstones. Components of the fault zone include secondary faults, boudins of sandstone and bedding-parallel lenses of massive quartz (<20 cm wide). (c) North facing section illustrating boudinaged chert layers or wedges between several steep-east-dipping quartz-filled faults (F–F) that have a combination of both reverse and normal senses of movement. (d) Tight south-plunging F1 anticline in interbedded chert and pelagic mudstone and wedge of chert between two reverse faults. Scale = 25 cm ruler.
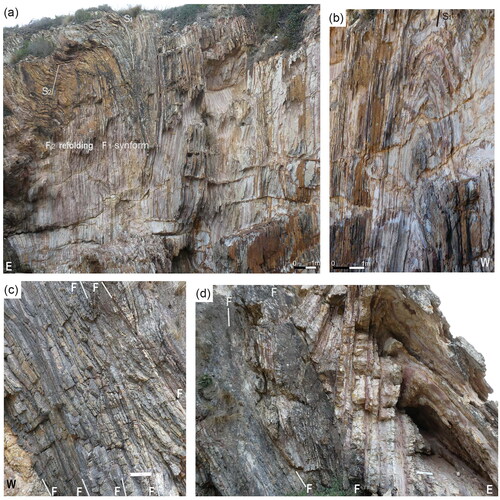
Section descriptions
Mallacoota to Bruces Creek
This section summarises the detailed maps and descriptions in Wilson et al. (Citation1982) and Fenton et al. (Citation1982), beginning in the north at Bastion Point and traversing south to Bruces Creek. At Bastion Point (), the turbidite sequence is deformed by upright, tight F2 folds that plunge at shallow angles to the southwest (Wilson et al., Citation1982, figure 5). Interbedded with the turbidites are thin nodular cherts and chert packages that are bounded by bedding-parallel faults, which generally preserve early steep-plunging and later sub-horizontal slickenside striations. F3 folds are confined to zones <20 m wide, which also contain northwest-trending faults, mostly with sinistral movement senses.
There are marked differences in strain distributions between the greywackes and the cherts. The cherts are typically more highly strained, notably just north of Geology Point. Here, the intercalated thin chert layers and pelagic mudstone have been folded into tight and isoclinal F1 folds (). The axial surfaces are sub-vertical except where refolded from the meso-scale to hundreds of metres scale, by F3 (). The F1 folds include limb thrusts, where elongate thin boudins have developed (). Quartz veins with dip-slip striations separate boudins and are parallel to bedding and fault planes. In the chert sequences, a rare cleavage is marked by quartz veins infilling brittle fractures and generally restricted to the hinge zone.
At Geology Point and Quarry Beach (), the area is dominated by isoclinally folded laminated chert and pelagic mudstone beds <6 cm thick. The sequence generally dips east, but has been locally modified by small-amplitude, south-plunging F2 folds. Faults with displacements from a few centimetres to 10 m parallel the axial surface and limbs of these folds. The recumbent F1 fold in the chert sequence at Quarry Beach lacks an obvious axial-planar foliation and closes to the west (). This is unlike the majority of upright or east-verging F2 folds in the greywacke sequences. It is considered that this fold and some other identical isolated folds with west-vergences in the chert sequences represent D1 structures.
At Secret Beach, there is a sequence of thick sandstone beds and minor shale with early deformation features, including ball-and-pillow structures () and F1 interlayered folds. The dominant structures are upright F2 folds with areas where the F2 folds have been refolded by F3 (). On the scale of individual outcrops (), the F2 hinges are thickened in the shale. Whereas, in the sandstone beds the limbs are highly thinned or collapsed and dismembered by thrust faults. The fold axes are markedly curved, plunging 40–70° to the southwest and locally refolded by co-planar, meso-scale, southerly plunging F3 folds with a vertical axial-planar crenulation cleavage in shale-dominated thinly layered sequences. This style of folding is typical of most sequences where thick sandstone is intercalated with minor shales, with local interference patterns between F2 and F3 folds.
Figure 8. Map showing the spatial variation of fold geometry and non-cylindricity in a dominantly sandstone sequence at Secret Beach. The southwestern outcrops are only accessible at low tide and northeast outcrops are islands. The collapsed tight to isoclinal abutting hinges of the major F2 folds are most likely formed by migration of shale and bedding-parallel thrusts, which induced greater variation of hinge plunge and is accompanied by a localised development of an S3 crenulation cleavage. The limb parallel faults comprise high-angle thrust faults infilled with brecciated quartz accompanied by marked changes in younging directions and are overprinted by oblique sub-vertical quartz-filled strike-slip faults. The stereonets illustrate the variation of the F2 and F3 fold plunges. Contour intervals for bedding (S0) are 0.5, 3, 5 and >10% per 1% area.
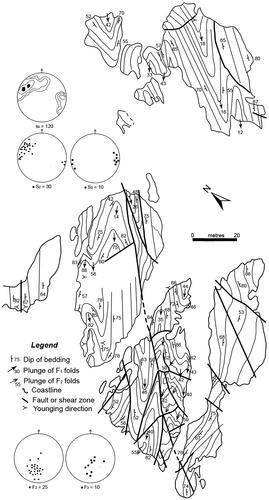
There are significant local style discrepancies between large-scale () and small-scale structures in the Pinnak Sandstone turbidites at Secret Beach and elsewhere. At small scales, significant shortening has produced buckling, a pervasive S2 cleavage and considerable curvature in the hinge lines and thickening of shale units in hinge zones. These F2 folds are accompanied by steep west-dipping, generally boudinaged quartz-filled limb thrusts () and folded early quartz vein sets (). The faults parallel the strike of the accompanying folds and may be limb wedge thrusts and out-of-syncline thrusts, which Mitra (Citation2002) considered to be common types of fold accommodation faults. Overprinting both the folds and faults are later small displacement (<2 m) D3 or later (D4) faults, generally quartz-filled, and associated with a crenulation cleavage ().
Figure 9. Structures observed in the vicinity of Secret Beach. (a) Elongate boudin and F1 folds in quartz-filled early north–south-trending fault reactivated during the D2 folding event. (b) Early D1 quartz veins folded (curved white lines) and brecciated by quartz-filled fracture sets and a related S3 crenulation in the shale unit. (c) Tight F1 fold in turbidites with the sandstone unit containing rip-up clasts of shale. (d) F1 folds offset by low-displacement sinistral faults. Scale = 25 cm ruler.
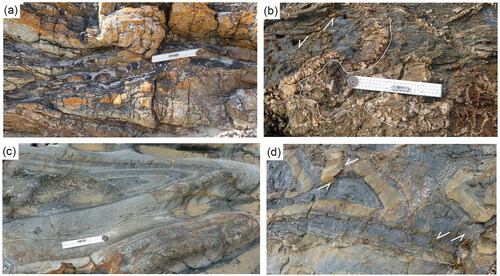
This style of folding (e.g. ) continues along the coast to south of Bruces Creek (Wilson et al., Citation1982), where a large F2 structure () folds slumped beds in a sequence of shale and sandstone with bed thicknesses ≤7 cm. These beds have sharp bases, and 27% of beds are composed of C(D)E divisions, which Fenton and Wilson (Citation1985) interpreted as an interchannel area with regular turbidite inputs from a distal channel. Mafic dykes (<4 m wide) crosscut the D2 structures and follow D3 fault zones.
Shipwreck Creek area
The rocks north of Shipwreck Creek () show F2 folds with wavelengths of 10–15 m that plunge south at 10–45° with concentric and rounded hinges in sandstone units and chevron hinges in the shales. Where thick sandstone units are intercalated with equally thick shale, shale units are thickened in fold hinges, while sandstone beds are thinned or attenuated in the fold limbs. There is a widely spaced S2 cleavage in the sandstones at a slight angle to the cleavage in the adjacent shale units (). F3 folds are locally developed, are open and rounded, and are associated with faults and, in the shale-rich units, a crenulation cleavage.
Figure 10. Trend surface maps in the coastal outcrops near Shipwreck Creek and representative structural data. Inset map shows location. (a) Shows trend in bedding in the folded sediments north (a, b) and south (c) of Shipwreck Creek. The narrow chert units in (c) are all associated with bedding-parallel faults. Contour intervals in (a, c) are 0.5, 3, 5, 7 and >10% per 1% area. Contour intervals in (b) are 1.5, 7 and >10% per 1% area. The location of is also shown in (c).
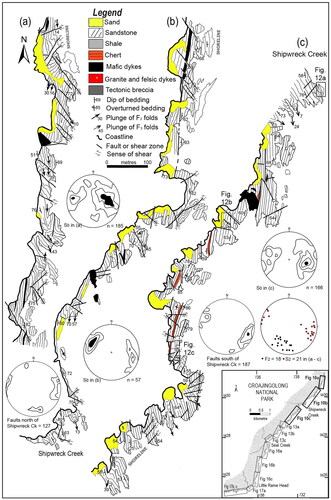
Figure 11. Representative structures from south of Shipwreck Creek. (a) Limb wedge thrust slices and radial quartz veins on the eastern limb of an F2 fold. The sandstone sequence is thrust over the anticlinal structure, outlined in white in shale unit. Scale = 25 cm ruler. (b) Steep west-dipping thrust between sandstone sequence in the east and a shale-rich sequence with prominent upright axial-planar S2 cleavage. Hammer scale = 34 cm. (c) Early bedding-parallel quartz veins (D1) folded by south-plunging F2 fold with S2 orientation indicated by white line. The fold is crosscut by later northeast–southwest-trending quartz veins. (d) South-southeast-plunging upright F2 folds in alternating beds of sandstone and siltstone warped by F3 folds and crosscut by later east- and west-dipping faults. Y = Younging direction in sandstones from southern area in . Hammer scale = 34 cm. (e) Quartz accumulations in a refolded early fault, between a sandstone and a shale unit showing oblique-slip quartz-rich slickenfibres. Hammer scale = 34 cm.
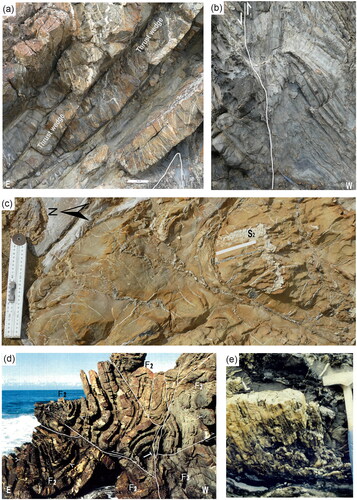
South of Shipwreck Creek, 10 cm- to 1 m-thick sandstone is interbedded with <5–30 cm-thick shales and in places ≤30 cm-thick chert layers of restricted length (). These cherts grade into mudstone and are associated with D1 bedding-parallel faults. F2 folds in the sandstones plunge to the south-southwest, verge to the east, have radial quartz-filled fracture sets (). Bedding maintains a constant orthogonal thickness in the hinges. The shale-siltstone units have a steep (∼80°) west-dipping cleavage and west-over-east thrusts generally occur where shale-rich units are juxtaposed against sandstone (). The cleavage is either a S2 crenulation cleavage or a disjunctive cleavage with parallel dark lamellae, which represent the insoluble residue of pressure solution (Powell, Citation1979). The cherts contain lenticular and flattened radiolarian-rich nodules and concentrations of pyrite. Numerous early bedding-parallel quartz-filled thrust faults (<15 cm wide) are folded by F2 folds and are more prominent in shale-siltstone and chert units next to the thick sandstones (). The boundaries between cherts and adjacent shales or sandstones contain stylolites, ptygmatically folded early-quartz veins and early bedding-parallel faults. Folds without cleavage in the thin chert units are tight to isoclinal D1 folds, and hinge regions are isolated by D1 limb thrusts.
On fold limbs in the greywacke sequence, D2 thrust wedges are common with slickenfibre lineations that mostly show dip-slip movement (). The thrust faults show up to four variably oriented lineations, suggesting multiple reactivations. These reactivations relate to brittle faulting and include: (1) northeast-trending dextral and southeast-trending sinistral strike-slip, steep-dipping faults that may also contain isolated lenses of highly deformed chert; (2) north-northwest dextral and less common northeast-trending sinistral faults that are associated with minor folds and kinks; and (3) en échelon vein sets and conjugate fault sets that have reactivated earlier faults with north-northwest sinistral and east–west-trending dextral oblique-slip movements and associated with ≤3 m-wide breccia zones.
Faults near Shipwreck Creek
A time–space framework for the faulting described above is shown in . It is based on the relative age relationships and proximity of neighbouring faults. The precise expression of each fault generation depends on the rheological contrast between the rock layers, which probably influenced the distribution and displacements mapped and varies from place to place. Early D1 faults are bed-parallel, in what may have been a largely conformable sedimentary pile. Interlayered folds are associated with sheared bed-parallel units and folded clastic dykes (Wilson & de Hedouville, Citation1985). The rocks show no evidence of cataclasis, but there are through-going slip surfaces with striations and quartz fibres showing reverse movements (). The slip surfaces are generally laterally continuous and can typically be constrained by matching bed markers across fault planes.
Figure 12. Fault geometries and structural data from typical sites immediately south of Shipwreck Creek. (a–c) Fault outcrop patterns in localities identified in (adapted from Schapper, Citation1991). Many of the bed-parallel D1 faults have either been folded, reactivated or crosscut by later faults. (d–f) Equal-area, lower-hemisphere stereoplots of D1 faults and lineations from the area displayed in , with early faults having steep-plunging lineations (black dots) and reverse west-over-east movement senses. Folded and reactivated D1 faults have both shallow plunging dextral (red dot) and sinistral (green dot) movement senses. (g) Rose diagram showing average trends of D1 faults identified in area shown in . (h) D3 faults showing dominant northerly trends and dextral movement senses. (i) Late D4 faults with both sinistral and reverse movement senses and a dominant east–west trend. The geometrical data have been replotted from measurements tabulated in Schapper (Citation1991, appendix 3).
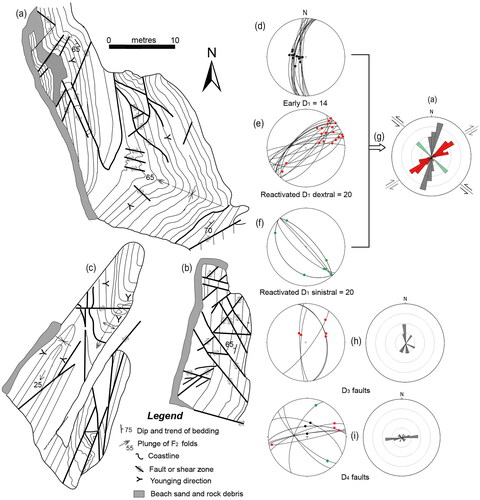
D1 faults appear to have initiated as low-angle bedding-parallel thrusts but have been rotated together with bedding to dips of >70° during D2 folding and consequently now have steep-plunging lineations (). Some faults now have round and circular outcrop patterns as a result of subsequent folding (), and the accumulated offsets across individual faults are difficult to establish as faults rarely have displacements at their termination. Such faults may have been reactivated several times and so do not represent a single slip event, with cumulative movements propagating from D1 faults into D2 folds and faults in the adjacent units (). The D2 fault movements are consistent with the F2 folds and accompanying S2 cleavage. The approximate north–south D1 and D2 fault orientation is probably related to the far-field east–west paleostress field that accompanied the end-Ordovician plate convergence ().
A range of relative overprinting relationships are observed within the population of D1 and D2 faults, and all of these are cut and offset by multiple strike-slip faults (). This combination has produced complex overprinting patterns like the ‘sawtooth’ profiles in . Steep-dipping dextral and sinistral strike-slip faults are the most prominent (). Late and small displacement (<50 cm) east–west-striking D4 dextral oblique-slip faults () associated with northwest-striking tension veins and iron oxide stained breccias appear to have reused and overprint favourably oriented segments of earlier D2 and D3 faults.
The rose diagrams in show the average strike of predominantly dextral fault planes, which strike northeast and east–west. Reanalysis of data obtained by Schapper (Citation1991) strongly suggests that the D1 faults (), formed in response to an approximately east–west paleostress field. After folding, these D1 faults have segments with orientations able to have been optimally reoriented and were reused for later sinistral- and dextral strike-slip movements. An Andersonian relationship (Anderson, Citation1951; Angelier, Citation1984) between the dextral and sinistral faults indicates approximately east–west compressive stress regime during the different faulting episodes.
Seal Creek area
Coastal outcrops at Seal Creek and immediately north (Clark, Citation1979) are dominated by upright F2 folds with sub-vertical axial surfaces with folds plunging 20–40° towards the south-southwest ( and ). Near Fold Beach (), large cylindrical and rounded F2 fold hinges are present in 10 cm- to 1 m-thick sandstone and shale units (). The thicker sandstone units commonly have quartz-filled fracture sets, oriented both parallel and perpendicular to the hinge lines (). These are overprinted by later quartz vein sets (e.g. X in ) that are quartz-filled joints related to crosscutting faults (). In shale-dominated packages, the profiles of F2 folds () vary from open chevrons in shale units to rounded tight folds cut by limb thrusts in the thin sandstone units (). Also, fold wavelength decreases from ∼10 to 3 m in the shale-dominated packages with a convergence of limbs in the sandstones.
Figure 13. Maps of coastal outcrops north of Seal Creek and representative structural data. The positions of cross-sections 1–5 illustrated in are shown and inset shows location of the detailed maps. Chert units occur as isolated lenses or boudins bounded by D1 bedding-parallel faults between the turbidites and are folded by F2. Contour intervals are 0.5, 3, 5, 9 and >12% per 1% area.
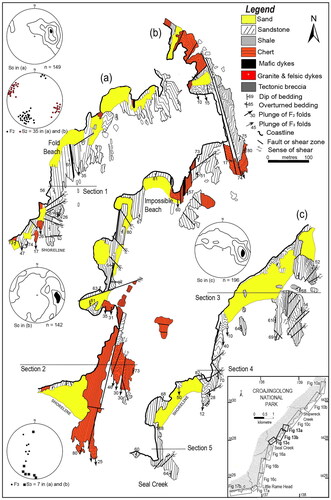
Figure 14. Schematic block diagram and cross-sections looking north showing the sequences and section lines identified in . (a) The chert unit in section 2 is part of an extended anticline that is crosscut by a large sinistral fault in the north. Further south in the cherts, reactivated D1 bedding-parallel faults bound blocks with different strain histories. (b) Sections illustrating structural relationships north of Seal Creek to Fold Beach.
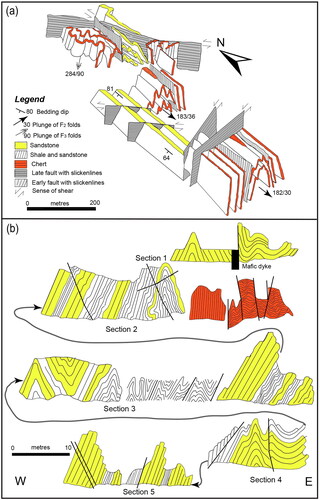
Figure 15. Representative structures in the vicinity of Fold Beach. (a) Cross-section of F2 anticline and syncline in thick sandstone with thin intercalated shale beds. Ruler scale = 25 cm. (b) Asymmetric F2 folds in a shale-dominated sequence with thin intercalated sandstone. (c) Enlargement of the area near the scale in (b). Collapsed F2 fold with thickening of shale in hinge and limb thrust in sandstone bed. (d) Longitudinal section through cylindrical F2 fold (width of photo 30 m) with extensional quartz-filled extensional veins sub-perpendicular to fold axis. White box = location of (e), which is enlargement, showing rectangular network of extensional veins parallel and perpendicular to the F2 fold axis. Superimposed on these are later irregular veins (X) associated with off-setting faults. (f) Sinistral offset of 70 cm of bedding and S2 fabrics along a weathered fault zone. The quartz veins are splays of hydrothermally filled sheared joints related to the fault zone.
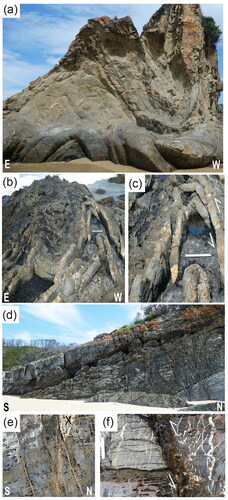
South of Seal Creek, the boundaries between the cherts and the turbidite rocks contain bedding-parallel faults. F2 fold hinges are abundant in the cherts, have a near vertical axial surface and ∼10°–40° plunges and are generally isolated by bedding-parallel faults (). The northern part of this structure is cut by a sinistral fault associated with locally developed F3 folds as broad open structures, plunging 80–90° (). To the west of the chert sequence, interbedded sandstone and grey shale contain Late Ordovician graptolites (de Hedouville & Wilson, Citation1983). These units are dominated by south-southwest-plunging asymmetric folds (). To date, only one graptolite locality has been identified in the Pinnak Sandstone in the Mallacoota region, and this was in the grey slate beds immediately to the north of Seal Creek.
West-dipping thrust faults control the geometries and kinematics of the F2 folds, which generally verge to the east (). However, because of the lack of continuity of outcrop, it is difficult to construct a balanced cross-section, as there is internal duplexing in the sequence, and strain magnitude varies with lithology. The shale- and chert-rich parts of the sections () have tighter folds with interlimb angles of 25–40°. In the sandstone dominant sequences, fold interlimb angles vary from 35 to 70°. The folding probably developed from shortening, with the later thrusting owing to flattening after fold lock-up (Hudleston & Treagus, Citation2010). Overall, the bulk shortening varies greatly between the different lithologies and can range from 30 to 60%.
North and west of Little Rame Head
In outcrops north of the Little Rame Head (), there are major F2 folds (Frew, Citation1979). These have parasitic folds that vary considerably from tight, with rounded hinges in 10 cm to 1 m sandstone beds (wavelength 5 m), to small chevron closures in shales (wavelength 10 cm). The F2 fold structures are cut by ∼20 gabbroic dykes (3–10 m wide), which generally have faults on one or both margins. Narrow (50 cm) andesitic dykes are present in the centres of some larger gabbroic dykes. One granophyric dyke is also present ().
Figure 16. Maps of coastal outcrops south of Seal Creek to Little Rame Head and representative structural data. The inset map shows location. Contour intervals in (a) are 0.5, 2, 5, 7 and >10% per 1% area. Contour intervals in (b and c) are 1, 3, 5 and >10% per 1% area.
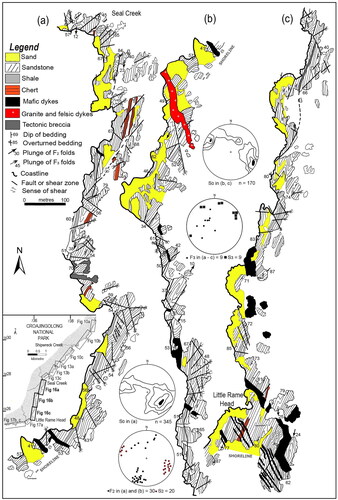
Strike-slip faults clearly displace F2 fold structures by <5 m and some have 1–4 m-wide D3 kink zones with a marked crenulation cleavage. The strike of the faults is quite variable (e.g. ), with prominent east–west and north-northwest fault sets. Six prominent mafic dykes on the Little Rame Head strike north-northwest () and are crosscut by small shear zones and quartz veins associated with D3 or a later D4 faulting event.
The sequence immediately west of the Little Rame Head () is on the western limb of a major F3 syncline exposed at Little Rame Head (). Further west (), tight, angular F2 folds and broad F3 folds are associated with a well-developed crenulation cleavage. The variations in structural style are shown in . F2 folds plunge 20–50° south-southwest, while the F3 folds are sub-horizontal north–south or have steep variable plunges when superimposed on the limbs of earlier F2 folds ().
Figure 17. Maps of coastal outcrops south of Little Rame Head and representative structural data. The inset map shows location of (a–c). (c) Trends in bedding south of Shipwreck Creek. Contour intervals in (a, b) are 0.5, 2, 5, 7 and >10% per 1% area. Contour intervals in (c) are 0.5, 2, 7 and >10% per 1% area. (d) Cross-section between X–X′–Xʺ in coastal outcrops illustrated in subarea (c).
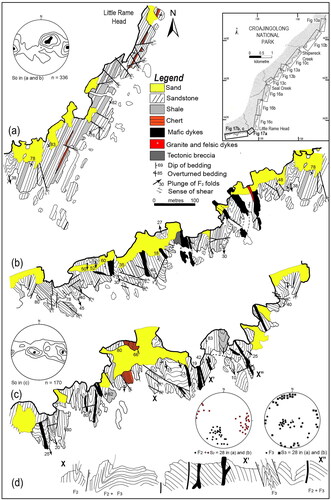
Benedore River to Sandpatch Point
Poorly sorted, quartz-rich sandstones with bed thicknesses of 10 cm to 3 m (average ∼75 cm) dominate the area. They show abundant grading, cross-beds, flute marks, shale rip-up clasts, flame and ball-and-pillow structures. The sandstones are interbedded with thin (15–30 cm) shale units and in places cherts (≤30 cm thick). The cherts contain lenticular and flattened radiolarian-rich nodules and localised concentrations of pyrite. Stylolites with crosscutting folded quartz veins (1–5 mm thick) mark the boundaries between the chert and adjacent shale or sandstone. The sequence south of Black Cocky Creek () is overprinted by the contact metamorphic effects of the Sandpatch Point Granite.
Figure 18. Maps between the Benedore River and coastal outcrops of the Sandpatch Point Granite with representative structural data. The inset map shows the location. (a–c) Trend surface maps and distribution of folded sediments with bedding and fold orientation data. Contour intervals in (a) are 0.2, 2, 5, 7 and 10% per 1% area. Contour intervals in (b and c) are 0.5, 2, 5 and 10% per 1% area.
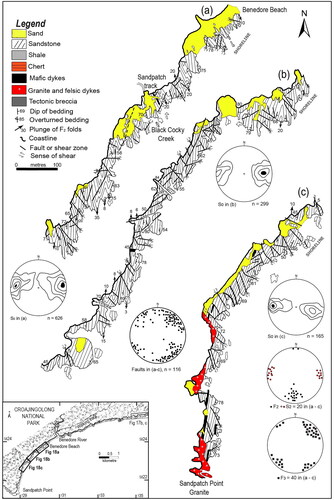
This section of the coast forms one homogeneous structural subarea with predominant south-plunging (<20°) tight, cylindrical F2 folds with wavelengths of 10–15 m. Where thick sandstone beds are intercalated with shale, beds are thickened in fold hinges, while beds in fold limbs are wedge-shaped or highly thinned, with adjacent syncline and anticlinal closures juxtaposed in places (). Variation in shale bed thickness within fold hinges indicates that during late F2 folding, the shale units largely migrated from the fold limbs into the adjacent anticlinal hinges to form a wide variety of shapes and allow the folds to tighten and flatten (). This may also have caused the local variations in hinge plunges in the shale units (Ramsay & Wood, Citation1973). The folds verge to the east (), with the area apparently on the eastern limb of a major south-plunging D2 anticlinorium.
Figure 19. Images showing structures south of the Benadore River. (a) Variations in F2 hinge geometry near Black Cocky Creek with a collapsed syncline sitting on top of the anticline in the foreground. Ruler scale is 25 cm. (b) Tight F2 fold in a laminated shale unit wedged between boudinaged sandstones. (c) East-verging F2 folds in interbedded shales and siltstones. (d) Pervasive quartz-filled tension gashes and en échelon vein arrays normal to F2 fold axes at the south end of Benadore Beach. (e) Early D1 bed-parallel fault with evidence of reactivation and folding of early quartz veins. (f) Reactivated dextral northeast-trending fault with cataclastic material and southerly plunging quartz fibres (white line). This fault obliquely crosscuts bedding that strikes 160°. Scale = 25 cm ruler.
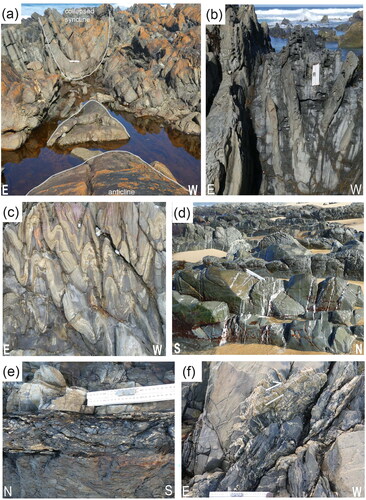
Dense quartz veining occurs along fold hinges and appears to be associated with the main regional folding. The veins are concentrated along and across bedding either as tension veins normal to fold hinges or in boudin necks in competent beds (). Some quartz veins, with ferruginous staining, vary greatly in shape, arrangement, structural position and age, and may be related to late fluids from the Sandpatch Point Granite. D1 faults that parallel bedding have been reactivated ().
The middle part of the area () is cut by vertical strike-slip faults with displacements >25 m that are accompanied by many smaller faults with displacements of 2 or 3 m, infilled with cataclasite, with variable strikes and both sinistral and dextral movement senses (). Cataclastic materials are composed of lenses of quartz in a muscovite-rich matrix or are a fault breccia that is clearly post-contact metamorphic.
The southern two-thirds of the exposure, about 400 m (), has been contact-metamorphosed by the Sandpatch Point Granite (Douglas, Citation1974). The sandstones were progressively changed to quartzite and, near the granite contact, the shale to hornfels with andalusite porphyroblasts containing retrograde white mica. Vertical strike-slip faults with dextral and sinistral movement senses cut the F2 folds and both pre-date and post-date the contact metamorphism. Rare D3 or D4 kinks and crenulation cleavage in shale units appear to be the same relative age and spatially related to the faulting.
Intrusions
Dykes or groups of dykes are typically spaced 2 km or so along the coast. Vertical mafic dykes (10 cm–20 m wide) intrude along the pre-existing D1 faults or post-date F2 folds. The age of many of these mafic dykes is uncertain, and no geochemical or geochronological studies are available to provide crucial information about their origin. In places, the dykes are crosscut by later shear zones and brittle faults (). In the Little Rame Head area ( and ), about 20 gabbroic dykes (3–10 m wide) have variable grainsizes. Some display 1–2 mm-long prismatic labradorite in a matrix of interstitial clinopyroxene with skeletal magnetite and ilmenite.
Figure 20. Schematic block diagram illustrating relationships between structural features in the Ordovician sedimentary rocks and the Late Devonian mafic dykes outcropping at the Little Rame Head ().
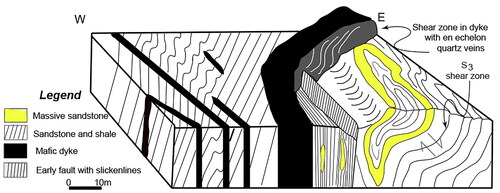
In the southern part of the region, mafic dykes are cut by the Sandpatch Point Granite, part of the Early Devonian Bega Batholith (). The granite includes a porphyritic granite phase, and a small area of biotite-rich granodiorite (Richards, Citation1979). The granite is predominantly of K-feldspar (35%), quartz (40%), albitic plagioclase (20%), biotite (5%) with muscovite, magnetite, zircon and apatite common minor phases. In the granodiorite, biotite (∼15%) and oligoclase are the major mineral phases. The granite grainsize is typically about 3 mm, while that of the granodiorite is 4–6 mm. The granite contacts with the host Ordovician bedrock dips ∼80° east and is generally concordant with strike of structures in the host bedrock. The contact is crosscut by aplitic dykes and rare pegmatite-rich dykes. Enclaves of deformed Ordovician turbidites, containing S2 foliations, are incorporated in the granite, which suggests the granite was emplaced during the Tabberabberan Orogeny.
The granite is cut by near-vertical north–south- and east–west-trending joints and numerous shallow-dipping (<20°) brittle fractures with variable strikes. There are also steep east–west-trending faults and 10 cm- to 4 m-wide shear zones with a pink discoloration from potassic alteration. The shear zones in places include <4 mm-wide closely spaced hydrothermally altered joints filled with fine (<3 mm) recrystallised quartz, pyrite, magnetite and muscovite aggregates with diameters ∼0.5 mm as minor phases. These infills have a similar composition to the quartz veins that cut the contact aureole. The larger shear zones also cut the contact aureole and are characterised by green cataclasite and breccia zones of quartz, sericite, epidote and chlorite. Their late timing indicates they formed in either late Tabberabberan or Kanimblan orogenic events.
At Little Rame Head, the shears and faults are also intruded by narrow fine-grained, porphyritic andesitic dykes containing <3 mm plagioclase laths. Both here and at Geology Point (), prominent porphyritic rhyolitic dykes contain phenocrysts of prismatic albite and quartz in a groundmass of sericitised alkali feldspar, plagioclase and quartz. These probably correlate with the Late Devonian bimodal Boyd Volcanic Complex in southern New South Wales (Fergusson et al., Citation1979).
A set of post-granite aged basaltic dykes (1–1.5 m wide) parallel the north–south joint set in the granites. These are probably related to Late Cretaceous volcanism associated with the formation of the Tasman Sea.
Discussion
Initial deformation in the turbidites
The earliest structures (D1) in the turbidite sequence are considered to have been generated in an accretionary prism along a convergent subduction-related system (). Data from the seismic section () indicate that the oldest and deeper sediments underwent a compressive stage at the commencement of the Benambran Orogeny at ca 450 Ma (Cayley pers. comm., 2024). Deformation features include bedding-parallel detachments or décollement surfaces, interlayered folds, local inversions in the sedimentary sequence and the development of the S* fabric. There is also evidence that some parts of the rock sequence at Mallacoota were deposited in waters as shallow as ≤200 m, prior to, during and after initial deformation (Fenton & Wilson, Citation1985).
During burial, these sediments would be unconsolidated, wet bodies with low viscosities and high-pore-fluid pressure conditions and experiencing very low confining pressures (Groshong, Citation1988). Their rheology is controlled by a weak matrix phase (e.g. clay, phyllosilicates and amorphous silica or chert) that deforms at high pore-fluid pressures (Cloos & Shreve, Citation1988; Marques et al., Citation2010). During burial and the associated increases in pressure and temperature, the rocks become less porous and unstable. This is particularly true for cherts and mudstones (Miliken & Olsen, Citation2017) and the quartz-rich detritus on any slope within a trench (Molenaar et al., Citation2021). The clay minerals break down and form other, less fissile, Al-rich silicates, making new fabrics. Whatever strain is present plays a critical role in defining the rheology, geometry and location of any décollement fault ruptures in such an accretionary prism environment (Chester et al., Citation2013; Vrolijk, Citation1990). Chert sequences, except for thin layers of nodular cherts, are all bounded or imbricated with bedding-parallel faults (e.g. ). Kakuwa and Webb (Citation2007) attributed the cherts to deep-ocean floor sedimentation. However, the cherts generally display imbricate thrusts, and D1 folds and boundaries may contain D1 bedding-parallel faults or décollements. This could explain the juxtaposition of some chert layers as allochthonous sheets or lenses occurring concurrently and incrementally throughout the evolution of the turbidite package.
The distribution and mode of deformation would have been strongly influenced by an interplay between material strength and stress evolution over time, at both large and small scales. A weakly aligned phyllosilicate fabric, such as S*, is initiated by tectonic dewatering under a small vertical compressive load, whereas mudstones in the deeper parts of the accretionary prism as in the Kuark Zone are more compacted and have a more prominent foliation than those in the shallower Mallacoota Zone sequences. The interstitial fluids and the physical behaviour of the sediments play critical roles in defining the nature of any subsequent deformation (Groshong, Citation1988; Morgan et al., Citation2007). The initial strength of the accretionary prism depends on the permeability and porosity differences between the dominant sandstone and lesser mudstone and chert sequences. Depositional porosity in mud is about twice as high as that in sand (Lundegard, Citation1992; Velde, Citation1996), which leads to heterogeneous fluid drainage. Muds and shales show large volume reductions and fluid expulsion on compaction. Sandstones compact less and may retain significant amounts of water, although this depends on the cementation state and the rates of buildup and dissipation of excess pore-fluid pressures (Lander & Walderhaug, Citation1999). As also noted by Marques et al. (Citation2010), sandstones can be fractured and boudinaged (with fractures later healed with quartz or sedimentary dykes), while shales rarely show fracturing or early quartz veining that crosses bedding.
Significant changes in mechanical conditions and sedimentary load would have influenced the initial gross accretionary wedge geometry, fault-spacing, porosity and permeability responsible for fluid transport into fractures (Morgan et al., Citation2007). The amount of strain attributed to the D1 fault offsets is compatible with the idea that the maximum horizontal stress is greater than the vertical stress provided by sediment loading. The array of D1 structures, such as slump beds, bedding-parallel faults and dewatering features are typical of systems where failure and unstable sliding along a fault are also driven by earthquakes (Molenaar et al., Citation2021; Scarselli, Citation2020). Active convergent margins are the locus of the largest damaging earthquakes on Earth (Moeremans et al., Citation2014; Morgan et al., Citation2007; Schellart & Rawlinson, Citation2013). As a consequence, a combination of the effects of low-angle fault geometries, a weak rheology and the compressional stress environment, these are the favoured sites for large seismic ruptures, and recurrence intervals can be thousands of years (Schellart & Rawlinson, Citation2013).
The field evidence indicates that all these processes took place in the sequences now cropping out at Mallacoota during D1. They would have been accompanied by seismic events, where the marine sediments were converted into rocks capable of unstable seismogenic sliding. There is no substantial recrystallisation in the quartz-rich turbidites, which implies a shallow depth of deformation and is consistent with long-timescale subduction processes (Morgan et al., Citation2007). During this time, intermittent earthquakes produced early faults, interlayered folds and quartz veins, which pre-date the F2 folds. Quartz precipitated along bedding, which would imply bedding-plane slippage on décollement or fault surfaces, aided early shearing and the formation of the S* cleavage (Wilson & de Hedouville, Citation1985). A major question relates to the origin of some of the tight to isoclinal folds in the mud-rich pelagic sediments and chert sequences (e.g. and ). It is not always possible to correlate fold structures in fault-bounded blocks of chert to F2 structures observed in the greywackes. Syn-sedimentary or D1 folding accompanying seismogenic sliding is the only explanation for the anomalous vergence changes observed in some chert units.
Significance of structures in the Pinnak Sandstone
Along the coastal section south of Mallacoota, F2 folds generally plunge south ∼10–30° with asymmetric folds displaying Z chirality, both looking down or horizontally ( and ). At a map scale, these asymmetric F2 folds are considered to have formed as part of a dextral shearing event. Along the coastal outcrops, the average strike of the S2 cleavage is ∼220° (), consistent with a dextral sense of shear.
Figure 21. Fold form surface map and distribution of major faults northwards from the Little Rame Head. The inset shows the location. (a) Map-scale outcrop pattern of F2 folds with a Z-shaped vergence (b) The map-scale pattern of the more prominent faults. (c–e) Rose diagrams from the north, central and southern areas, highlighting the sense of shear and strike-direction associated with the major faults. These indicate there was a near-east–west-trending compression event postdating the regional folding event producing the dominant northwest-trending sinistral faults.
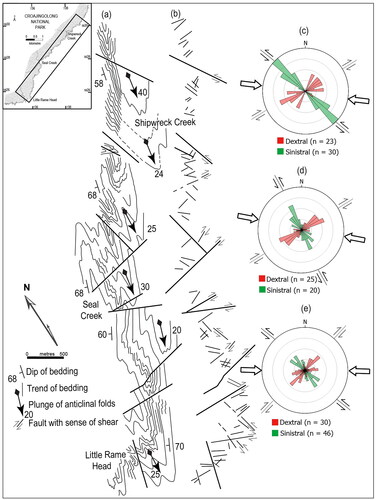
Figure 22. Fold form surface map and major fault pattern from the Benedore River to the Sandpatch Point Granite. The inset shows the location. (a) Outcrop-scale Z-shaped folds that plunge south and are progressively rotated to a north–south orientation, which parallels the contact with the Sandpatch Point Granite. Note the difference in fold style to that in . (b) Map-scale pattern of the more prominent faults. (c) Rose diagram highlighting the sense of shear and strike-direction associated with the major faults. The calculated paleostress was a near-east–west-trending compression postdating the regional folding event.
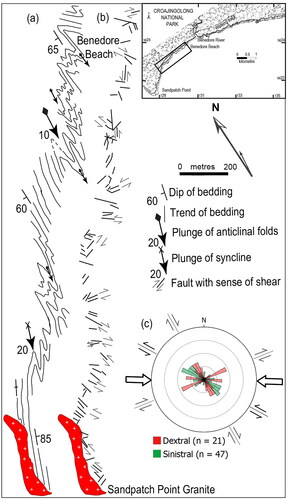
Figure 23. Representative orientation of foliations, folds, faults and paleostress in areas north of Seal Creek and south of the Benedore River. Superimposed on the equal area lower hemisphere projections are rose diagrams from and . (a) Data from south of Shipwreck Creek, which is typical of areas dominated by D2 structures northwards to Mallacoota. The paleostress related to the north-northeast-trending foliation is oblique to that calculated from the later faults. The folds generally plunge south-southwest, parallel to the flow plane and extension direction defined by the foliation. (b) Data from south of Benedore River where the foliation and F2 folds plunge north–south, and the two east–west paleostress directions coincide, related to the foliation development and the overprinting later faults.
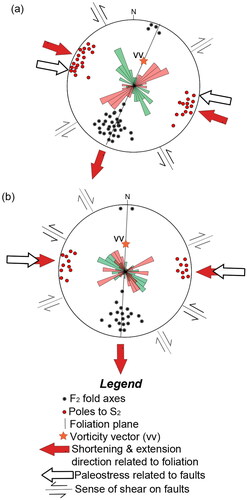
During the Early Devonian (), the eastern hinge of the Lachlan Orocline was outboard of the Selwyn Block (Cayley, Citation2011; Moore et al., Citation2016; Moresi et al., Citation2014; Musgrave, Citation2015). The transpressional environment that existed at this stage initially facilitated a pure shear deformation that controlled the developing S2 foliation with the orientation of the S2 foliation defining the XY-plane of the bulk finite strain ellipsoid. Because it was coaxial, the pure shear was more efficient in accumulating finite strain than the transpressional component of simple shear in producing the S2 foliation (Jones et al., Citation2004; Pfiffner & Ramsay, Citation1982). Accompanying this, fold axes rotated towards the extensional flow direction, parallel to the maximum principal strain rate axis and either parallel or perpendicular to the vorticity vector (rotation axis) and the shear direction (Sanderson & Marchini, Citation1984). The plunges of many F2 folds are oblique to both the vorticity vector and the paleostress axis, and the gross flow has a triclinic symmetry (). This triclinic component would have been superimposed on an earlier monoclinic pure shear component as the initial S2 foliation developed. D3 faults show a different paleostress change during progressive deformation () with a dominantly sinistral movement sense, which is compatible with lateral extrusion parallel to the maximum principal strain rate axis of the pure shear component during triclinic flow (e.g. Jones et al., Citation1997).
The complex relationships between the D3 and D4 brittle faults and accompanying folds and related crenulation cleavages or kinks suggest further coaxial flattening. The mostly sinistral D3 faults (e.g. ) overprint the regional F2 folds and associated axial plane cleavages (). These mimic changes in the shortening and extension directions. Similarly, the east–west-trending later extension-related veins, which crosscut F2 fold axes, can be interpreted as part of a regional D3 east–west-trending compressional event that may be attributed to the Tabberabberan Orogeny. Further rotation of the S2 foliation () and tightening of the F2 folds, along with conjugate faulting from east–west shortening, is particularly noticeable near the Sandpatch Point Granite (). Here, earlier faults and new conjugate faults ( and ) that contain breccias and cataclasites crosscut the contact aureole and are probably associated with the Kanimblan Orogeny.
Rheology during the Bindian folding and cleavage development
Post-consolidation and lithification of the sediments meant that the sediments were capable of undergoing significant compressional stresses in comparison with localised conditions initially present in the accretionary wedge. Coupled with this and the onset of the Bindian Orogeny, there was a marked increase in material strength and rheology that led to the D2 regional deformation structures. The mesoscopic F2 folds in the greywackes show a systematic change from tight asymmetric folds with limb thrusts in the northern areas (). Limb thrusts separate domains in which F2 folds have the same geometry and a common orientation dominates. In many folds, there are slickensided bedding surfaces, which are taken to indicate flexural slip during buckling, and very few folds have parallel geometries (Ramsay, Citation1967). In folds south of the Benedore River, there is a strong flattening strain adjacent to the Sandpatch Point Granite (). Until very late in the folding history, the shale-rich layers appear to have acted as a relatively low-viscosity fluid, that migrated into the hinge zones, producing collapsed synclines (e.g. ). In the chert sequences, the minor F2 folds have profiles that resemble a flattened class 1 C (Ramsay, Citation1967), with major thickening of shale layers in the hinge regions. Shale-rich multilayer sequences interlayered with thick sandstone commonly show fracturing normal to fold hinges ( and ). Quartz-filled radial veins () also show that brittle fracturing late in the folding process occurred in the hinges.
The consistent southerly plunges of ∼30° of the asymmetric fold trains ( and ) suggest a component of dextral transtension-related tilting and an obliquity to the regional east–west compression. This is consistent with a transpressional deformation, where the vorticity vector (vv in ) may not be parallel to the vertical stretch producing varying fold plunges depending on the magnitude of simple shear (Robin & Cruden, Citation1994). The curved and non-cylindrical fold hinges can also be attributed to syn-folding shale migration and volume reduction during shortening (Ramsay & Wood, Citation1973).
Paleostress and rheology during the episodes of faulting
The orientations of the brittle strike-slip faults can be used to evaluate the paleostress field that overprinted the gross architecture of the D2 ductile deformation (Anderson, Citation1951). During D2, the paleostress was related to the regional east–west shortening in combination with north–south extension. The rose diagrams () suggest that, in the Mallacoota region, the maximum principal paleostress during later brittle faulting was close to east–west.
South of the Benedore River towards the granite contact (), the density of faults increases and shows many orientations that crosscut and/or merge with one another. These show dextral oblique-slip reactivation and are infilled with coarse-grained quartz, suggesting the granite expelled quartz-rich fluids. Mapping showed the granite intruded after the D2 deformation and was associated with dextral deformation during east–west flattening. In this part of East Gippsland, this is a Tabberabberan granite belonging to the I-type suite, emplaced between 398 and 369 Ma (VandenBerg et al., Citation1995). As the granite crystallised, contact metamorphism strengthened the turbidite sequence. This strengthening resulted in the late reactivation of pre-existing faults during further east–west compression. The strike- and oblique-slip fault overprint of the contact aureole implies that this late reactivation was a Kanimbian event.
Regional significance of the structural evolution
The seismic section interpretation in , generated by Resources Victoria (Citation2024, March 4), provides key data on the location of markers for structural reconstruction of major lithological units. This section can be related to the landward portion of the Late Ordovician accretionary prism sequence, as recognised at Mallacoota and elsewhere in eastern Victoria (Willman et al., Citation2005), and to tectonically thickened and older Cambrian sequences in New South Wales. In the seismic profile (), contrasts in structural character and physical properties across multiple décollements point to contrasting juxtaposed lithologies. As mechanical boundaries, décollements are assumed to play important roles in defining the behaviour and geometry of units within an accretionary prism (Morgan & Karig, Citation1995). The interpretation () shows flatter, older faults, duplicating and disrupting the Cambrian volcanic and oceanic sedimentary sequences, equated with units identified at Narooma (Glen et al., Citation2004; Wilson, Citation1968). Duplication of Cambrian sequences in the wedge, tectonically sandwiched between older Cambro-Ordovician sediments of the Lower–Middle Ordovician Adaminaby Group, is greatest in the east. The distribution is asymmetric, and it appears that tectonic thickening occurred by progressive incorporation of allochthonous units during deformation of the prism. Slabs of the abyssal-plain must have been transported tens of kilometres inboard from the prism front, without much disturbance of the overlying sedimentary sequences. This passage on sub-horizontal fault offsets is preserved as localised syn-sedimentary D1 deformation.
The Pinnak Sandstone was not directly deposited on the earlier abyssal-plain turbidites but was a Middle–Upper Ordovician sequence broadly coincident with several shifts in paleoenvironmental conditions. In the Mallacoota area, there are few coarse sandstones, and those that occur tend to be grouped together, indicating repeated turbidite events along a south-to-north channel axis (Fenton et al., Citation1982). The supply of sediments was potentially controlled by the waning tectonic processes occurring in the Transgondwanan Supermountains and its final dispersion of detritus along the paleo-Pacific margin in the Gondwanan super-fan system (Squire et al., Citation2006). The sequence also shows evidence of both typical ‘fan-like’ and ‘shallow-water’ features (Fenton & Wilson, Citation1985). Paleo-bathymetric calculations suggest that portions of this sequence were deposited in ≤200 m of water (Fenton & Wilson, Citation1985). Collectively, all these features would suggest that the Pinnak Sandstone at Mallacoota is stratigraphically higher in the accretionary prism and could have been subject to large subaqueous slope failures and the formation of the D1 structures (Molenaar et al., Citation2021).
The seismic section images a series of multiple ∼50° east-dipping discontinuous fault strands that offset the flatter older faults in an extensional fashion and terminate below the inferred Moho and offset Cambrian units (). Units in the accretionary wedge have been thrust up against these large crustal-scale east-dipping faults, and against the inferred wedge of the Macquarie Arc (Fergusson & Colquhoun, Citation2018). The westernmost of these major east-dipping faults forms the western boundary to the Ordovician accretionary rocks mapped as the McLauchlan Creek Fault Zone, which separates the Deddick Zone from the Kuark Zone (Rawling et al., Citation2011; VandenBerg et al., Citation1992). This fault zone shows both extension and contractional features with an east-side up displacement (VandenBerg et al., Citation1992) and represents the backstop to the accretionary wedge (Resources Victoria, Citation2024, March 4). The evolution of such east-dipping faults has been demonstrated in the Moresi et al. (Citation2014) model and can be related to deformation at the apex of VanDieland. The older Ordovician sediments were under semicontinuous extension as they round the apex until a later subduction zone is established further east in the Silurian (), and the tectonic stress field then goes back to east–west compression. The east-dipping faults lie approximately orthogonal to the inferred orientation of the maximum D2 horizontal paleostress () during the Bindian Orogeny (ca 420 Ma) and were reactivated during the Tabberabberan Orogeny (ca 385–370 Ma).
From the late Silurian (Bindian Orogeny) to the Middle–Late Devonian (Tabberabberan Orogeny), the deformation included a component of oblique transpression along the paleo-Pacific plate boundary (Moresi et al., Citation2014). During these orogenies, local transtension took place in the upper part of the accretionary wedge, allowing for the emplacement of the sheet-like Bega Batholith. As shown by Moresi et al. (Citation2014) the Bindian and Tabberabberan orogenies are part of a long process, started in the Early Ordovician when VanDieland first came close to Gondwana and finished at the end of the Tabberabberan (or later), when west-dipping subduction completely re-established outboard of Gondwana (). Inside this system, dextral strike-slip movements gave rise to releasing and restraining bends. The releasing bends have granite present, such as the Sandpatch Point Granite, and the restraining bends show contemporaneous compression and the initiation of late D3 faults. On a large-scale, vertical faults transect the inferred flat sheet of the Bega Batholith and include the Combienbar, Fiddlers Green and Burragate faults ( and ).
During the Early Devonian, there was cratonisation of the accretionary prism and the establishment of a stable plate margin. Denudation and uplift of this eastern portion of the Lachlan Orogen during the Middle–Late Devonian (385–370 Ma) gave rise to the continental sediments and volcanic detritus, which filled restricted basins (e.g. ) bounded by strike-slip faults (Fergusson et al., Citation1979; Young, Citation2007). Further restricted east–west compression in the Kanimblan Orogeny (ca 354–343 Ma) reactivated earlier fault structures and gently folded the Middle and Upper Devonian basin infills (Fergusson, Citation2023). Strain reactivation, controlled by the localised strain reorientation, occurred along the boundary of the Sandpatch Point Granite and was accompanied by a significant change in crustal strength and the development of brittle faults.
Conclusions
The depositional and structural history of the Middle–Upper Ordovician Pinnak Sandstone at Mallacoota is a critical element in the evolution of the accretionary prism located in a trench on the paleo-Pacific margin of Gondwanaland. Deformation began with syn-sedimentary imbrication and thickening of the accretionary wedge that led to heterogeneous fluid drainage and formation of quartz-filled faults and fractures. Imbrication was accompanied by juxtaposition of allochthonous chert sequences within the turbidite package. After lithification, these sediments were overprinted by intense regional-scale D2 ductile deformation during the Bindian Orogeny and produced tight regional-scale F2 folds. These two events produced structures that are replicated in other parts of the paleo-Pacific margin of Gondwana. From the late Silurian Bindian to Middle–Late Devonian Tabberabberan orogenies, the oblique compression across the accretionary wedge meant this part of the trench propagated and tilted southwards. This took place while the sediments underwent dextral shear or transpression, as shown by the many Z-shaped east-verging F2 folds and lateral stretching parallel to the shallowly south-plunging (20–30°) fold axes. Some additional variation in fold plunges may be due to strain heterogeneities induced by the relative viscosities of chert and mudstone sequences compared with the sandstone sequences.
There are significant strain variations along the coastal section south from Mallacoota together with some obliquity between the D2 foliation development and the shear component during initial crustal shortening. This shortening included shear components in both early limb thrusts and later strike-slip faults during the Bindian and Tabberabberan orogenies. Superimposed on these are sets of Carboniferous Kanimblan event, conjugate faults related to an east–west compression, which also deformed the Tabberabberan granites and the overlying Middle to Upper Devonian sedimentary rocks. Other parts of this Ordovician sequence in the Lachlan Orogen from Batemans Bay to southwest of Mallacoota include similar rocks and may have undergone a similar tectonic history.
Access
Since the 2020 bushfire and subsequent vegetation regrowth in the Croajingolong National Park, combined with very restricted overland access provided by Parks Victoria, many of these coastal outcrops can now only be inspected with great difficulty. As a result, some locations of these rocks can only be accessed by boat.
Supplemental Material
Download PDF (940.5 KB)Acknowledgements
CW wishes to acknowledge the First Nations Bidhawal people and Krauatungalang people of the Gunaikurnai Nation on whose land this detailed mapping was undertaken in collaboration both with a group of excellent honours students and with Pierre de Hedouville from the University of Lille, France. Field data collection was achieved using aerial photographs, taken with a 70 mm Vinten camera hung over the side of a low-flying Cessna aircraft. Initial mapping of the coastal outcrops was undertaken at scales between 1:200 and 1:1500 from camp sites located in what is now known as the Croajingolong National Park. Members of the Mallacoota community are thanked for their support during various stages of the fieldwork and my numerous visits. A special thanks goes to Robin Clark, Max Frew, Michael Richards and Martin Schapper who were able to provide CW with copies of their honours work, which were either lost or pulped by Melbourne University. David Moore supplied a constructive review and insights into aspects of the regional geology, which greatly improved an early draft of this paper. The author gratefully acknowledges Paul Lennox for comments in review. Ross Cayley is thanked for providing , which has an initial interpretation of the line 3 seismic section, a very constructive review of this paper, followed up by some positive discussions. Chris Fergusson is also thanked for his editorial suggestions.
Disclosure statement
No potential conflict of interest was reported by the author(s).
Data availability
The author confirms that the data supporting the findings of this study are available within the article and/or its supplemental data.
References
- Anderson, E. M. (1951). The dynamics of faulting and Dyke Formation with applications in Britain (2nd ed.). Oliver and Boyd.
- Angelier, J. (1984). Tectonic analysis of fault slip data sets. Journal of Geophysical Research: Solid Earth, 89(B7), 5835–5848. https://doi.org/10.1029/JB089iB07p05835
- Beck, M. (1983). On the mechanism of tectonic transport in zones of oblique subduction. Tectonophysics, 93(1-2), 1–11. https://doi.org/10.1016/0040-1951(83)90230-5
- Begg, G., Burg, J-P., & Wilson, C. J. L. (1987). Ductile and brittle deformation in the Cann Valley granitoids, Victoria. Australian Journal of Earth Sciences, 34(1), 95–110. https://doi.org/10.1080/08120098708729395
- Bouma, A. H. (1962). Sedimentology of some flysch deposits (p. 168). Elsevier.
- Burg, J-P., & Wilson, C. J. L. (1988). A kinematic analysis of the southernmost part of the Bega Batholith. Australian Journal of Earth Sciences, 35(1), 1–13. https://doi.org/10.1080/08120098808729435
- Cas, R. A. F. (1983). A review of the palaeogeographic and tectonic development of the Palaeozoic Lachlan Fold Belt of southeastern Australia (Vol. 10). Geological Society of Australia Special Publication.
- Cawood, P. A. (2005). Terra Australia Orogen: Rodinia breakup and development of the Pacific and Iapetus margins of Gondwana during the Neoproterozoic and Paleozoic. Earth-Science Reviews, 69(3-4), 249–279. https://doi.org/10.1016/j.earscirev.2004.09.001
- Cayley, R. A. (2011). Exotic crustal block accretion to the eastern Gondwanaland margin in the Late Cambrian–Tasmania, the Selwyn Block, and implications for the Cambrian–Silurian evolution of the Ross, Delamerian and Lachlan orogens. Gondwana Research, 19(3), 628–649. https://doi.org/10.1016/j.gr.2010.11.013
- Cayley, R. A., Taylor, D. H., VandenBerg, A. H. M., & Moore, D. H. (2002). Proterozoic–Early Palaeozoic rocks and the Tyennan Orogeny in central Victoria: The Selwyn Bock and its tectonic implications. Australian Journal of Earth Sciences, 49(2), 225–254. https://doi.org/10.1046/j.1440-0952.2002.00921.x
- Chester, F. M., Rowe, C., Ujiie, K., Kirkpatrick, J., Regalla, C., Remitti, F., Moore, J. C., Toy, V., Wolfson-Schwehr, M., Bose, S., Kameda, J., Mori, J. J., Brodsky, E. E., Eguchi, N., Toczko, S., & Expedition 343 and 343T Scientists. (2013). Structure and composition of the plate-boundary slip zone for the 2011 Tohoku-Oki earthquake. Science, 342(6163), 1208–1211. https://doi.org/10.1126/science.1243719
- Clark, R. G. (1979). Structural geology near Shipwreck Creek, Croajingolong, Victoria [Unpublished BSc(Hons) thesis]. The University of Melbourne.
- Cloos, M., & Shreve, R. L. (1988). Subduction-channel model of prism accretion, melange formation, sediment subduction, and subduction erosion at convergent plate margins: I. Background and description. Pure and Applied Geophysics, 128(3-4), 455–500. https://doi.org/10.1007/BF00874549
- Collins, W. J., Wiebe, R. A., Healy, B., & Richards, S. W. (2006). Replenishment, crystal accumulation and floor aggradation in the megacrystic Kameruka Suite, Australia. Journal of Petrology, 47(11), 2073–2104. https://doi.org/10.1093/petrology/egl037
- Coney, P. J., Edwards, A., Hine, R., Morrison, F., & Windrim, D. (1990). The regional tectonics of the Tasman Orogenic System, eastern Australia. Journal of Structural Geology, 12(5-6), 519–543. https://doi.org/10.1016/0191-8141(90)90071-6
- Davidson, N. (2021). The greywacke. Pacific Books Ltd.
- de Hedouville, P. (1984). Etude Geologique des Mallacoota Beds (Ordovicien) Victoria, Australie [Unpublished thèse 3ème cycle]. L’Universite de Lille.
- de Hedouville, P., & Wilson, C. J. L. (1983). Late Ordovician graptolites from the Mallacoota Beds, East Gippsland. Proceedings of the Royal Society of Victoria, 95, 255–258.
- Douglas, J. G. (1974). Explanatory notes for the Mallacoota 1:250 000 geological map sheet SJ.55–8. Victorian Geological Survey.
- Etheridge, M. A., Ransom, D. M., Williams, P. F., & Wilson, C. J. L. (1973). Structural evidence of the age of folded rocks on the South Coast of N.S.W. Journal of the Geological Society of Australia, 19(4), 465–470. https://doi.org/10.1080/00167617308728814
- Fenton, M. W., Keene, J. B., & Wilson, C. J. L. (1982). The sedimentary and environmental deposition of the Mallacoota Beds, eastern Victoria. Journal of the Geological Society of Australia, 29(1-2), 107–114. https://doi.org/10.1080/00167618208729198
- Fenton, M. W., & Wilson, C. J. L. (1985). Shallow-water turbidites: An example from the Mallacoota Beds, Australia. Sedimentary Geology, 45(3-4), 231–260. https://doi.org/10.1016/0037-0738(85)90004-1
- Fergusson, C. L. (2023). Structure of the Upper Devonian Merimbula Group, Lachlan Orogen, southeastern costal New South Wales. Proceedings of the Linnean Society of New South Wales, 145, 55–73.
- Fergusson, C. L., Cas, R. A. F., Collins, W. J., Craig, G. Y., Crook, K. A. W., Powell, C. M., Scott, P. A., & Young, G. C. (1979). The upper Devonian Boyd Volcanic complex, Eden, New South Wales. Journal of the Geological Society of Australia, 26(1-2), 87–105. https://doi.org/10.1080/0016761708729270
- Fergusson, C. L., & Colquhoun, G. P. (2018). Ordovician Macquarie Arc and turbidite fan relationships, Lachlan Orogen, southeastern Australia: Stratigraphic and tectonic problems. Australian Journal of Earth Sciences, 65(3), 303–333. https://doi.org/10.1080/08120099.2018.1425909
- Fergusson, C. L., & Coney, P. J. (1992). Implications of a Bengal Fan-type deposit in the Paleozoic Lachlan Fold Belt of southeastern Australia. Geology, 20(11), 1047–1049. https://doi.org/10.1130/0091-7613(1992)020<1047:IOABFT>2.3.CO;2
- Fergusson, C. L., & Fanning, C. M. (2002). Late Ordovician stratigraphy, zircon provenance and tectonics, Lachlan Fold Belt, southeastern Australia. Australian Journal of Earth Sciences, 49(3), 423–436. https://doi.org/10.1046/j.1440-0952.2002.00929.x
- Fergusson, C. L., & Frikken, P. (2003). Diapirism and structural thickening in an early Palaeozoic subduction complex, southeastern New South Wales, Australia. Journal of Structural Geology, 25(1), 43–58. https://doi.org/10.1016/S0191-8141(02)00017-2
- Fisher, D., & Byrne, T. (1987). Structural evolution of underthrusted sediments, Kodiak Islands, Alaska. Tectonics, 6(6), 775–793. https://doi.org/10.1029/TC006i006p00775
- Fitch, T. J. (1972). Plate convergence, transcurrent faults, and internal deformation adjacent to southeast Asia and the western Pacific. Journal of Geophysical Research, 77(23), 4432–4460. https://doi.org/10.1029/JB077i023p04432
- Frew, M. R. (1979). Geology of Little Ram Head, Croajingolong, Victoria [Unpublished BSc(Hons) thesis]. The University of Melbourne.
- Fry, M. C., & Wilson, C. J. L. (1982). The geology of Cape Everard, Victoria. Proceedings of the Royal Society of Victoria, 94(4), 173–181.
- Glen, R. A., Percival, I. G., & Quinn, C. D. (2009). Ordovician continental margin terranes in the Lachlan Orogen, Australia. Implications for tectonics in an accretionary orogen along the east Gondwana margin. Tectonics, 28(6), TC6012. https://doi.org/10.1029/2009TC002446
- Glen, R. A., Stewart, I. R., & Percival, I. G. (2004). Narooma Terrane: Implications for the construction of the outboard part of the Lachlan Orogen. Australian Journal of Earth Sciences, 51(6), 859–884. https://doi.org/10.1111/j.1400-0952.2004.01090.x
- Gray, D. R., & Foster, D. A. (2004). Tectonic evolution of the Lachlan Orogen, southeastern Australia: Historical review, data synthesis and modern perspectives. Australian Journal of Earth Sciences, 51(6), 773–817. https://doi.org/10.1111/j.1400-0952.2004.01092.x
- Groshong, R. H. (1988). Low-temperature deformation mechanisms and their interpretation. Geological Society of America Bulletin, 100(9), 1329–1360. https://doi.org/10.1130/0016-7606(1988)100%3C1329:LTDMAT%3E2.3.CO;2
- Harland, W. R. (1971). Tectonic transpression in the Caledonian Spitsbergen. Geological Magazine, 108(1), 27–41. https://doi.org/10.1017/S0016756800050937
- Hobbs, B. E. (1962). Structural analysis of a small area in the Wagonga Beds at Narooma, N.S.W. Journal of the Geological Society of Australia, 9(1), 71–86. https://doi.org/10.1080/00167616208728516
- Hudleston, P. J., & Treagus, S. H. (2010). Information from folds: A review. Journal of Structural Geology, 32(12), 2042–2071. https://doi.org/10.1016/j.jsg.2010.08.011
- Jenkins, C. J., Kidd, P. R., & Mills, K. J. (1982). Upper Ordovician graptolites from the Wagonga Beds near Batemans Bay, New South Wales. Journal of the Geological Society of Australia, 29(3-4), 367–373. https://doi.org/10.1080/00167618208729220
- Jiang, D., Lin, S., & Williams, P. F. (2001). Deformation path in high-strain zones, with reference to slip partitioning in transpressional plate-boundary regions. Journal of Structural Geology, 23(6-7), 991–1005. https://doi.org/10.1016/S0191-8141(00)00170-X
- Jones, R. R., Holdsworth, R. E., & Bailey, W. (1997). Lateral extrusion in transpression zones. Journal of Structural Geology, 19(9), 1201–1217. https://doi.org/10.1016/S0191-8141(97)00034-5
- Jones, R. R., Holdsworth, R. E., Clegg, P., McCaffrey, K., & Tavarnelli, E. (2004). Inclined transpression. Journal of Structural Geology, 26(8), 1531–1548. https://doi.org/10.1016/j.jsg.2994.01.004
- Kakuwa, Y., & Webb, J. (2007). Trace fossils of a Middle to Upper Ordovician pelagic deep-ocean bedded chert in southeastern Australia. In R. G. Bromely, L. A. Buatois, G. Mangano, J. F. Genise, & R. N. Melchor (Eds.), Sediment–organism interactions: A multifaceted ichnology (Vol. 88, pp. 267–276). SEPM Society for Sedimentary Geology, Special Publication. https://doi.org/10.2110/pec.07.88.0267
- Lander, R. H., & Walderhaug, O. (1999). Predicting porosity through simulating sandstone compaction and quartz cementation. American Association of Petroleum Geology Bulletin, 83(3), 433–449. https://doi.org/10.1306/00AA9BC4-1730-11D7-8645000102C1865D
- Lundegard, P. D. (1992). Sandstone porosity loss – ‘Big picture’ view of the importance of compaction. Journal of Sedimentary Research, 62(2), 250–260. https://doi.org/10.1306/D42678D4-2B26-11D7-8648000102C1865D
- Marques, F. O., Burg, J-P., Lechmann, S. M., & Schmalholz, S. M. (2010). Fluid-assisted particulate flow of turbidites at very low temperatures: A key to tight folding in a submarine Variscan foreland basin of SW Europe. Tectonics, 29(2), TC2005. https://doi.org/10.1029/2008TC002439
- McCaffrey, R. (1992). Oblique plate convergence, slip vectors, and forearc deformation. Journal of Geophysical Research: Solid Earth, 97(B6), 8905–8915. https://doi.org/10.1029/92JB00483
- Miliken, K. L., & Olsen, T. (2017). Silica diagenesis, porosity evolution, and mechanical behaviour in siliceous mudstones, Mowry Shale Cretaceous, Rocky Mountains, U.S.A. Journal of Sedimentary Research, 87(4), 366–387. https://doi.org/10.2110/jsr.2017.24
- Miller, J. M., & Gray, D. R. (1996). Structural significance of sediment subduction-accretion in a Palaeozoic accretionary complex, southeastern Australia. Journal of Structural Geology, 18(10), 1245–1258. https://doi.org/10.1016/S0191-8141(96)00042-9
- Miller, J. M., & Gray, D. R. (1997). Subduction-related deformation and the Narooma anticinorium, eastern Lachlan Fold Belt, southeastern New South Wales. Australian Journal of Earth Sciences, 44(2), 237–251. https://doi.org/10.1080/08120099708728307
- Mitra, S. (2002). Fold-accommodation faults. American Association of Petroleum Geology Bulletin, 86(4), 671–693. https://doi.org/10.1306/61EEDB7A-173E-11D7-86450000102C1865D
- Moeremans, R., Singh, S. C., Mukti, M., McArdle, J., & Johansen, K. (2014). Seismic images of structural variations along deformation front of the Andaman–Sumata subduction zone: Implications for rupture, propagation and tsunamigenesis. Earth and Planetary Science Letters, 386(1), 75–85. https://doi.org/10.1016/j.epsl.2013.11.003
- Molenaar, A., Van Daele, M., Vandorpe, T., Degenhart, G., De Batist, M., Urrutia, R., Pino, M., Strasser, M., & Moernaut, J. (2021). What controls the remobilization and deformation of surficial sediment by seismic shaking? Linking lacustrine slope stratigraphy to great earthquakes in South-Central Chile. Sedimentology, 68(6), 2365–2396. https://doi.org/10.1111/sed.12856
- Moore, D. H., Betts, P. G., & Hall, M. (2015). Fragmented Tasmania: The transition from Rodinia to Gondwana. Australian Journal of Earth Sciences, 62(1), 1–35. https://doi.org/10.1080/08120099.2014.966757
- Moore, D. H., Betts, P. G., & Hall, M. (2016). Constraining the VanDieland microcontinent at the edge of East Gondwana, Australia. Tectonophysics, 687, 158–179. https://doi.org/10.1016/j.tecto.2016.09.009
- Moresi, L., Betts, P. G., Miller, M. S., & Cayley, R. A. (2014). Dynamics of continental accretion. Nature, 508(7495), 245–248. https://doi.org/10.1038/nature13033
- Morgan, J. K., & Karig, D. E. (1995). Décollement processes at the Nankai accretionary margin, southeast Japan: Propagation, deformation, and dewatering of naturally deformed rocks. Journal of Geophysical Research: Solid Earth, 100(B8), 15221–15231. https://doi.org/10.1029/95JB00675
- Morgan, J. K., Ramsey, E. B., & Ash, M. V. S. (2007). Deformation and mechanical strength of sediments at the Nankai Subduction Zone. In T. Dixon (Ed.). The seismogenic zone of subduction thrust faults. MARGINS Theoretical Institute Series (pp. 210–256). Columbia University Press.
- Musgrave, R. J. (2015). Oroclines in the Tasmanides. Journal of Structural Geology, 80(1), 72–98. https://doi.org/10.1016/j.jsg.2015.08.010
- Mutti, E., & Ricci-Lucchi, F. (1972). Le torbiditi dell’ Appenino settentrionale: Introduzione all’ analise di facies. Memorie della Societa Geologica Italina, 11, 161–199. (translated in International Geological Reviews, 20(2), 125–166 (1978).) https://doi.org/10.1080/00206817809471524
- Packham, G. H., & Hubble, T. C. T. (2016). The Narooma Terrane offshore: A new model for the southeastern Lachlan Orogen using data from rocks dredged from the New South Wales continental slope. Australian Journal of Earth Sciences, 63(1), 23–61. https://doi.org/10.1080/08120099.2016.1150346
- Pfiffner, O. A., & Ramsay, J. G. (1982). Constraints on geological strain rates: Arguments from finite strain states of naturally deformed rocks. Journal of Geophysical Research: Solid Earth, 87(B1), 311–321. https://doi.org/10.1029/JB087iB01p00311
- Powell, C. M. (1979). A morphological classification of rock cleavage. Tectonophysics, 58(1-2), 21–34. https://doi.org/10.1016/0040-1951(79)90320-2
- Powell, C. M. (1983). Tectonic relationship between the Late Ordovician and the Late Silurian palaeogeographies of southeastern Australia. Journal of the Geological Society of Australia, 30(3-4), 353–373. https://doi.org/10.1080/00167618308729262
- Powell, C. M. (1984). Terminal fold-belt deformation: Relationship of mid-Caboniferous megakinks in the Tasman fold belt to coeval thrusts in cratonic Australia. Geology, 12(9), 546–549. https://doi.org/10.1130/0091-7613(1984)12<546:TFDROM>2.0.CO;2
- Powell, C. M., & Rickard, M. J. (1985). Significance of the early foliation at Bermagui, N.S.W., Australia. Journal of Structural Geology, 7(3-4), 385–400. https://doi.org/10.1016/0191-8141(85)90043-4
- Ramsay, J. G. (1967). Folding and fracturing of rocks. McGraw-Hill Book Company.
- Ramsay, J. G., & Wood, D. S. (1973). The geometric effects of volume change during deformation processes. Tectonophysics, 16(3-4), 263–277. https://doi.org/10.1016/0040-1951(73)90015-2
- Rawling, T. J., Osborne, C. R., McLean, M. A., Skladzien, P. B., Cayley, R. A., & Williams, B. (2011). 3D Victoria final report (Geosciences Victoria 3D Victoria Report 14, p. 98). Victorian Department of Primary Industries.
- Resources Victoria. (2024, March 4). Southeast Lachlan Deep Crustal Seismic Reflection Survey. https://resources.vic.gov.au/projects/eastern-victoria-geoscience-initiative/southeast-lachlan-deep-crustal-seismic-reflection-survey
- Richards, M. N. (1979). The geology between the Benodore River and Sandpatch point, Croajingolong, Victoria [Unpublished BSc(Hons) thesis]. The University of Melbourne.
- Robin, P-Y F., & Cruden, A. R. (1994). Strain and vorticity patterns in ideally ductile transpressional zones. Journal of Structural Geology, 16(4), 447–466. https://doi.org/10.1016/0191-8141(94)90090-6
- Sanderson, D., & Marchini, R. D. (1984). Transpression. Journal of Structural Geology, 6(5), 449–458. https://doi.org/10.1016/0191-8141(84)90058-0
- Scarselli, N. (2020). Submarine landslides – architecture, controlling factors and environments. A summary. In N. Scarselli, D. Chiarella, A. W. Bally, J. Adam, & D. G. Roberts (Eds.), Regional geology and tectonics: Principles of geologic analysis (pp. 417–439). Elsevier. https://doi.org/10.1016/B978-0-444-64134-2.00015-8
- Schapper, M. C. (1991). Structural evolution of the Mallacoota Beds at Shipwreck Creek, Eastern Victoria [Unpublished BSc(Hons) thesis]. The University of Melbourne.
- Schellart, W. P., & Rawlinson, N. (2013). Global correlations between maximum magnitudes of subduction zone interface thrust earthquakes and physical parameters of subduction zones. Physics of the Earth and Planetary Interiors, 225(1), 41–67. https://doi.org/10.1016/j.pepi.2013.10.001
- Simoes, M., & Avouac, J. P. (2006). Investigating the kinematics of mountain building in Taiwan from the spatiotemporal evolution of the foreland basin and western foothills. Journal of Geophysical Research: Solid Earth, 111, B10401. https://doi.org/10.1029/2005JB004209
- Squire, R. J., Campbell, I. H., Allen, C. M., & Wilson, C. J. L. (2006). Did the Transgondwanan Supermountain trigger the explosive radiation of animals on Earth? Earth and Planetary Science Letters, 250(1-2), 116–133. https://doi.org/10.1016/j.epsl.2006.07.032
- Squire, R. J., & Wilson, C. J. L. (2005). Interaction between collisional orogenesis and convergent-margin processes: Evolution of the Cambrian proto-Pacific margin of East Gondwana. Journal of the Geological Society, 162(5), 749–761. https://doi.org/10.1144/0016-764904-087
- Stewart, I. R., & Glen, R. A. (1991). New Cambrian and Early Ordovician ages from New South Wales South Coast. Quarterly Notes of the New South Wales Geological Survey, 85, 1–8.
- Stirling, J. E., Kemp, A. I. S., & Denyszyn, S. W. (2022). U–Pb geochronology of the Silurian–Devonian Bega Batholith, south-eastern Australia: Insights into the origin and development of I-type granites. Gondwana Research, 111(1), 1–19. https://doi.org/10.1016/j.gr.2022.07.006
- VandenBerg, A. H. M., Caluzzi, J., Willocks, A. J., & O’Shea, P. J. (1995). The geology and prospectivity of the Mallacoota 1:250 000 sheet, Eastern Highlands VIMP area. Geological Survey of Victoria VIMP Report, 8.
- VandenBerg, A. H. M., Nott, R. J., & Glen, R. A. (1992). Bendoc 1:100,000 map geological report. Geological Survey of Victoria Report 90.
- VandenBerg, A. H. M., & Stewart, I. R. (1992). Ordovician terranes of the southeastern Lachlan Fold Belt: Stratigraphy, structure and palaeogeographic reconstruction. Tectonophysics, 214(1-4), 159–176. https://doi.org/10.1016/0040-1951(92)90195-C
- VandenBerg, A. H. M., Willman, C. E., Mather, S., Simons, B. A., Cayley, R. A., Taylor, D. H., Morland, V. J., Moore, D. H., & Radojkovic, A. (2000). The Tasman Fold Belt System in Victoria. Geology and mineralisation of Proterozoic to Carboniferous rocks. Geological Survey of Victoria Special Publication, Department of Natural Resources and Environment.
- Velde, B. (1996). Compaction trends of clay-rich deep sea sediments. Marine Geology, 133(3-4), 193–201. https://doi.org/10.1016/0025-3227(96)00020-5
- Vrolijk, P. (1990). On the mechanical role of smectite in subduction zones. Geology, 18(8), 703–707. https://doi.org/10.1130/0091-7613(1990)018%3C0703:OTMROS%3E2.3.CO;2
- Walker, R. G. (1978). Deep water sandstone facies and submarine fans: Modes for exploration for stratigraphic traps. Bulletin American Association of Petroleum Geology, 62(6), 932–966. https://doi.org/10.1306/C1EA4F77-16C9-11D7-8645000102C1865D
- Williams, P. F. (1970). A criticism of the use of style in the study of deformed rocks. Geological Society of America Bulletin, 81(11), 3283–3296. https://doi.org/10.1130/0016-7606(1970)81[3283:ACOTUO]2.0.CO;2
- Williams, P. F. (1971). Structural analysis of Bermagui area, N.S.W. Journal of the Geological Society of Australia, 18(3), 215–228. https://doi.org/10.1080/00167617108728761
- Willman, C. E., Cayley, R. A., VandenBerg, A. H. M., Haydon, S. J., Osborne, C. R., Seymon, A. R., & Thom, J. L. (2005). Dargo 1:100,000 map geological report. Geological Survey of Victoria Report, 126.
- Wilson, C. J. L. (1968). Geology of the Narooma area, N.S.W. Journal and Proceedings of the Royal Society of New South Wales, 101(3-4), 147–157. https://doi.org/10.5962/p.360921
- Wilson, C. J. L., & de Hedouville, P. (1985). Early cleavage development in the Late Ordovician of north-east Victoria, Australia. Journal of Structural Geology, 7(3-4), 401–408. https://doi.org/10.1016/0191-8141(85)90044-6
- Wilson, C. J. L., Harris, L. B., & Richards, A. L. (1982). Structure of the Mallacoota area, Victoria. Journal of the Geological Society of Australia, 29(1-2), 91–105. https://doi.org/10.1080/00167618208729197
- Wilson, C. J. L., Moore, D. H., Vollgger, S. A., & Madeley, H. E. (2020). Structural evolution of the orogenic gold deposits in central Victoria, Australia: The role of regional stress change and the tectonic regime. Ore Geology Reviews, 120, 103390. https://doi.org/10.1016/j.oregeorev.2020.103390
- Young, G. C. (2007). Devonian formations, vertebrate faunas and age control on the far south coast of New South Wales and adjacent Victoria. Australian Journal of Earth Sciences, 54(7), 991–1008. https://doi.org/10.1080/08120090701488313