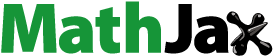
ABSTRACT
Rhizobia are soil bacteria that form nodules on legumes and fix atmospheric nitrogen, reducing the need for chemical fertilisers. In most studies rhizobia are described as alpha-proteobacteria and have been studied extensively for several decades. However, in recent years an increasing number of studies present beta- and gamma-proteobacteria strains as potential rhizobia-like bacteria. In this study we identify bacteria isolated from nodules of various legumes grown in Latvia, using a full-length 16S rRNA gene sequence, used for more precision, compared with a partial sequence of the gene. Bacterial strains in this study have been isolated from 1962–2019, part of which are rhizobia strains from a unique historical collection. This is the first time bacterial strains isolated from nodules in Latvian soils have been identified with molecular biology methods. A wide taxonomic diversity was detected – bacteria species from α-, β-, γ-proteobacteria classes and Paenibacillus polymyxa strains from the Bacilli class. P. polymyxa strains were detected only in the historical collection, while β- and γ-proteobacteria strains were obtained only from the newly isolated specimens, uncovering new potential strains for a commercial legume seed inoculum. Soil samples were collected, and phosphorus levels were determined. Several strains indicate phosphate solubilising properties.
Introduction
Legumes are a significant part of a sustainable agriculture. Therefore, the cultivation of legumes has been encouraged, especially in the European Union, through several programmes, such as EU Common Agricultural PolicyFootnote1 and the European Green Deal.Footnote2 Due to the increasing importance of legume cultivation, there is a growing need for effective legume seed inoculants, that include rhizobia bacteria. Rhizobia are soil bacteria, that attach to the roots of legumes and, in the case of a successful symbiosis, develop nodules that fix atmospheric nitrogen. The high added value of this rhizobia-legume symbiosis is the fixed atmospheric nitrogen that reduces the need for chemical nitrogen fertilisers, therefore reducing the cost for the farmer. Rhizobia-legume symbiosis is considered beneficial from the standpoint of a sustainable agriculture (Wang et al. Citation2019). Many genetically and/or phenotypically distinct rhizobium strains exist, differing in their efficiency to fix atmospheric nitrogen, affecting legume yield and protein content (Saleh et al. Citation2013). Genetic identification and taxonomic diversity determination of rhizobia gives an insight into the mechanisms of these bacteria, providing an opportunity to explain the phenotypic differences between different rhizobia strains, that cannot be done using classical agronomy and microbiology methods. The Latvia University of Life Sciences and Technologies (LBTU) Rhizobia Collection contains historical bacterial strains that have been isolated and tested for nitrogen-fixing efficiency since 1962. Up until now these historical strains have not been identified with molecular biology methods and their actual identity is practically unknown.
Although rhizobia bacteria are considered to be one of the most important microorganisms that form symbiotic relationship with legumes and fix atmospheric nitrogen, these are not the only soil bacteria capable of fixing atmospheric nitrogen (Wagner Citation2011). Therefore, when conducting studies on the diversity of legume nodule harbouring bacteria, it would be necessary to identify the presence of other nitrogen-fixing bacteria in the soil besides rhizobia belonging to the α-proteobacteria class, also known as α-rhizobia. Knowledge of the diversity of biological nitrogen-fixing bacteria, especially wild populations, is important to better understand the efficiency and role of biological nitrogen fixation in sustainable agriculture (Kawaka et al. Citation2018). Therefore, when identifying unknown native soil bacterial strains, the most commonly used marker is 16S rRNA gene.
The 16S rRNA gene consists of both conservative regions that are identical for many bacterial genera, and also hypervariable regions that can contain different genetic information not only between different species, but also within a species – between different strains (Janda and Abbott Citation2007; Pongsilp Citation2012). A 500–700 bp DNA fragment sequence of the gene is often sufficient for a successful classification of bacterial strains. However, choosing which gene region to target is critical, as identification results may vary depending on the choice of the gene fragment (Browman et al. Citation2015; Wagner et al. Citation2016). It has been argued that the use of this marker in bacterial classification is limited because of the incomplete 16S rRNA gene fragment (∼500 bp) sequencing (Earl et al. Citation2018), which is often used for rapid determination of rhizobia strain relatedness (Pongsilp Citation2012). Therefore, most of the information on environmental samples available in the open access databases consists of partial 16S rRNA gene sequences (Singer et al. Citation2016; Wagner et al. Citation2016). Although the use of individual variable 16S rRNA gene region sequences to identify new bacterial strains at the genus or species level is less time consuming, only the full or nearly full-length 16S rRNA gene sequence shows greater taxonomic accuracy and increases the phylogenetic resolution (Browman et al. Citation2015; Singer et al. Citation2016; Wagner et al. Citation2016; Earl et al. Citation2018; Johnson et al. Citation2019).
The main aim of this study was to supplement the LBTU Rhizobia Collection with new bacterial strains, isolated from nodules of a wide variety of host-plants, and for the first time in Latvia – identify these bacterial strains with molecular biology methods.
Materials and methods
Historical bacterial strain storage
Part of the bacterial strains used in the study were strains from the LBTU Rhizobia Collection (Institute of Soil and Plant Sciences). These strains have been isolated in Latvia from nodules of various legumes since 1962 up until 2015 (; Table S1). These strains have been stored in the refrigerator (4°C) on yeast mannitol agar (YMA) containing Petri dishes. To maintain the viability of these bacterial strains, they have been plated onto new Petri dishes at least once per year.
Figure 1. Sites in Latvia where bacteria isolation from legume nodules has been carried out. The percentage of the circle chart indicates the time period during which the bacteria were isolated at the specific sampling site. Sampling sites: 1. Priekuli; 2. Skriveri; 3. Koknese Municipality; 4. Kūka Parish; 5. Griškāni Parish; 6. Stolerova Parish; 7. Bauska; 8. Olaine Minicipality; 9. Jelgava; 10. Dimzas; 11. Peterlauki; 12. Vecauce; 13. Jurmala; 14. Tume Parish; 15. Pure; 16. Plavas; 17. Talsi; 18. Stende; 19. Edole Parish; 20. Jurkalne Parish; 21. Medze Parish; 22. Tadaiki Parish.

Bacterial isolation
New bacterial strains were isolated from different territories of Latvia in 2016, 2017 and 2019. The main purpose of the isolation was to complement the existing LBTU Rhizobia Collection with strains isolated from a wide variety of host-plants. Sampling from legume nodules was done randomly from different territories in Latvia (). In order to isolate new strains, the host plant was excavated in a radius of about 15 cm around it and about 20 cm in depth. Nodules were cut off together with a small part of the root (approx. 0.5 cm in each direction from the nodule). All nodules from one plant were stored together in one centrifuge tube containing silica gel and cotton wool (ration 1:1). The nodules were rinsed in distilled water, surface sterilised and then rinsed in sterile distilled water. Sterile nodules were crushed, and the bacterial suspension was smeared on a YMA Petri dish supplemented with one of the indicator dyes (0.0025% Congo Red or 0.002% bromothymol blue). Petri dishes were incubated in the dark at 25°C–30°C until slimy bacterial cultures appear (3–10 days). A pure culture of the newly isolated bacterial strain was obtained using the dilution method (Tampakaki et al. Citation2014).
DNA extraction and 16S rRNA gene amplification
DNA was extracted from the pure cultures of the bacterial strains using PrepMan™ Ultra Sample Preparation Reagent (Applied Biosystems; ThermoFisher Scientific; U.S.A.), according to the kit protocol. Amplification of the 16S rRNA gene was done using MicroSeq® Full Gene 16S rDNA Bacterial Identification PCR Kit (Applied Biosystems, U.S.A.) according to the provided protocol. To ensure a high quality, full-length gene sequence, it was amplified in three separate PCR reactions, resulting in three fragments. Amplified PCR products were purified from dNTPs and primers using a mixture containing 0.5 µL exonuclease Exo I (20 units µL−1; ThermoFisher Scientific, U.S.A.) and 1 µL alkaline phosphatase Fast AP (1 unit µL−1; ThermoFisher Scientific, UNITED STATES). 1.5 µL of this mixture was added to 5 µL of PCR product, mixed and processed at 37°C for 15 min and 85°C for 15 min.
16S rRNA gene sequencing
Sequencing reactions of purified PCR products were performed using the MicroSeq Full Gene 16S rDNA Bacterial Identification Sequencing kit (Applied Biosystems, U.S.A.) according to the provided protocol. Each of three amplified fragments of one bacterial strain sample were sequenced using both forward and reverse primers resulting in six sequencing samples per one strain. After sequencing, PCR products were cleaned using cartridges (MicroSEQ™ ID Sequencing Clean-up Cartridges; Applied Biosystems™; U.S.A.) following the provided protocol. The electrophoresis of sequencing PCR products was performed using a Sanger genetic analyzer (3500 Genetic Analyzer; Applied Biosystems/HITACHI, U.S.A.). Parameters of the genetic analyzer: capillary length 50 cm; 96-well plates (MicroAmp Optical 96-Well reaction plate; ThermoFisher Scientific, U.S.A.); polymer POP–7™ (Applied Biosystems™, U.S.A.). Identified full-length 16S rRNA gene sequence data of the bacterial strains has been submitted to the NCBI GenBank database, and can be found under the accession numbers listed in Table S2.
16S rRNA gene sequence analysis
Analysis of the sequencing results was performed using CLC Main Workbench 20.0.2. (Qiagen, U.S.A.). All sequences were manually proofed to confirm that the programme has performed an accurate determination of the nucleotide sequences. Sequencing for each fragment of the gene was performed in both directions, thereby confirming the nucleotide sequence of the fragment. All six sequences of one strain sample were compiled and the complete sequence was used for strain identification as well as for phylogenetic analyses. Identification of bacterial strains was performed using the publicly available US NCBI BLAST® database (https://blast.ncbi.nlm.nih.gov/Blast.cgi).
A multiple sequence alignment of all full-length 16S rRNA gene sequences was done using the CLC Main Workbench 20.0.2 programme using the following parameters: gap open cost = 10.0; gap extension cost = 1.0; end gap cost = as any other; alignment mode = very accurate. In addition to the bacterial strains included in this study, full-length or nearly full-length 16S rRNA gene sequences of closely related bacteria from the NCBI BLAST® database were used as reference strains.
Prior to the construction of the phylogenetic tree, strains with identical 16S rRNA sequences were removed from the sequence alignment, leaving only one representative sample (further – genotype) for each unique full-length 16S rRNA gene sequence. Based on the multiple sequence alignment, a phylogenetic tree was created using the parameters: Neighbour Joining phylogenetic dendrogram construction method; nucleotide substitution model = Kimura 80. Bootstrap analysis method was used in 1000 repetitions – values of 70% or higher indicate the reliability of the phylogenetic tree branching (Sleator Citation2011).
Conserved and hypervariable regions of the 16S rRNA gene
Hypervariable regions of the full-length 16S rRNA gene were determined by calculating how often the most frequent nucleotide is at the given position (bp) in a multiple sequence alignment of the particular strain genotype:
where: a = occurrence of the nucleotide at the given position in the sequence comparison; k = maximum frequency index ( = 1); n = total number of samples in the sequence alignment.
Soil phosphorus analysis
Soil samples were collected simultaneously with the isolation of new bacterial strains. Phosphorus (P2O5) content in the soil was determined in LBTU Institute of Soil and Plant Sciences according to the standard Egner-Reihm method (LV ST ZM 82-97). Results were reviewed both according to the host plants of the isolated bacterial strains and according to the genera or species of the identified strain. For data visualisation, MS Excel programme Box and Whisker diagrams were used, showing the distribution of data within the given group.
Results
Bacterial strain isolation and genetic identification
In addition to 46 rhizobia strains from the pre-existing historical LBTU Rhizobia Collection, 143 new bacterial strains were isolated from nodules of various legumes. In total, the renewed collection includes bacterial strains isolated from 14 different legume genera, from which 59 strains were isolated from Vicia faba, 31 strains from Glycine max, 23 strains from Trifolium spp., 22 strains from Pisum sativum and 15 strains were isolated from Medicago spp. Less than 10 strains were isolated from each of the remaining 10 legume genera (Table S1). The isolation of new strains was done based on a set of considerations. First, bacterial strains were mainly isolated from the existing ongoing trials in Latvia working on legumes – mainly V. faba, G. max and P. sativum. The rest of the legumes were chosen to expand the genetic diversity of the Rhizobia Collection, by isolating them both from field experiments, pot experiments, from various agricultural production areas, as well as from adjacent areas of these field trials. Most of the on-going legume trials were located in Zemgale, Kurzeme and Latgale, therefore bacterial strains were mostly isolated from these regions in Latvia ().
Identification of the full-length 16S rRNA gene of bacterial strains was performed. In total, 173 bacterial strains were included in the identification process, conducting 1038 sequencing reactions. Not all sequencing samples resulted in high quality chromatograms, therefore, from the analysed 173 samples, successful identification could be done for 146 bacterial strains (Table S2). All identified bacterial strains show at least 99% similarity with the 16S rRNA gene sequences of organisms available in the NCBI database.
Conserved and hypervariable regions of the full-length 16S rRNA gene
Sequencing results between bacterial strain genotypes within a proteobacteria class were compared to determine the hypervariable and conserved regions of the full-length 16S rRNA gene. Gene fragments, where nucleotides in the particular position of all genotypes were identical (nucleotide frequency index = 1), were considered as conserved regions. While gene fragments, in which different nucleotides were observed in the specific position (nucleotide frequency index <1), were considered as variable regions.
Results show () that full-length 16S rRNA gene hypervariable regions of α-proteobacteria match the data found in literature (Singer et al. Citation2016). 16S rRNA sequence variability of β-proteobacteria class genotypes can be observed less; however, these regions match with data in literature and is consistent for all of the researched α-proteobacteria genotypes. However, the variability in the case of β-proteobacteria can be observed less, as the number of bacterial strains identified as β-proteobacteria is significantly lower, compared to α-proteobacteria. High 16S rRNA sequence variability can be observed in the case of γ-proteobacteria. When comparing the sequence variability of α- and γ-proteobacteria genotypes, there are some fragments that are conserved in the case of α-proteobacteria (e.g. fragment between M5 and M6), but show high sequence variability in the case of γ-proteobacteria.
Figure 2. 16S rRNA gene conserved and hypervariable regions. Hypervariable regions (M1–M9) are coloured light blue, according to literature data (Singer et al. Citation2016). Bacterial strains from α-, β- and γ-proteobacteria classes (‘α’, ‘β’, ‘γ’) were analysed separately.

Taxonomic diversity of legume nodule harbouring bacterial strains
Prior to the phylogenetic analysis, identified strains with identical 16S rRNA gene sequences were combined into one phylogenetic group (genotype) – using one representative sample for the phylogenetic analysis. As a result, 62 unique genotypes were obtained (Table S2). Some of these genotypes are unique to individual samples, while some genotypes (e.g. RhL01, RhL02) combine several strains with identical 16S rRNA gene sequences. In the phylogenetic tree, which includes genotypes of all the bacterial strains, as well as reference genotypes, four clusters can be observed (). These genotypes have not grouped randomly, but rather according to their appropriate taxonomic affiliation. Genotype clusters belong to the α-proteobacteria, β-proteobacteria, γ-proteobacteria and Bacilli classes.
Figure 3. Full-length 16S rRNA gene phylogenetic tree, constructed using maximum likelihood method with JTT matrix and gamma rate distribution, indicating the overall legume nodule harbouring strain diversity of identified bacterial genera in Latvia.

Of the 96 bacterial strains identified as α-proteobacteria class, 88 strains represent genus Rhizobium – 43 of them are identified as R. leguminosarum and four as R. gallicium, while the affiliation of the remaining strains could only be identified at the genus level. Among the identified α-proteobacteria strains, one is identified as Ensifer adhaerens, three Neorhizobium galegae, two Mesorhizobium sp., one Azospirillum sp. and one Bradyrhizobium japonicum strain. Of the 14 strains identified as β-proteobacteria, seven strains are identified as Paraburkholderia terricola, one strain as Paraburkholderia caledonica, five as Burkholderia sp. and one strain as Achromobacter sp. Out of 28 bacterial strains identified as γ-proteobacteria, seven are Enterobacter genus representatives, from which three are Enterobacter ludwigii and one Enterobacter asburiae; eight strains are identified as Pseudomonas sp. (including Pseudomonas frederiksbergensis); six Rahnella aquatilis strains; four Serratia plymuthica and three strains identified as Pantoea agglomerans. Seven of the bacterial strains were identified as Bacilli – six Paenibacillus polymyxa and one Paenibacillus peoriae. shows the overall taxonomic diversity that has been detected in this study, showing all the detected bacterial genera, and their phylogenetic relatedness, within each bacterial class.
To enable a more in-depth phylogenetic analysis and genetic comparison of closely related bacterial strains, phylogenetic trees were created separately for each of the proteobacteria classes. Bootstrap values are indicated at the branching points – if the value is greater than 50%, it indicates that the clusters are significantly different. The distance between the branches indicates the genetic similarity of the strains – the shorter they are, the more genetically similar the genotypes are.
Full-length 16S rRNA gene phylogenetic analysis of the alpha-proteobacteria class strains
In the phylogenetic analysis, bacterial strains identified as α-proteobacteria () form a dendrogram that can be separated into 11 smaller clusters. Cluster II includes the most strains from historical Rhizobia Collection, compared to the other clusters. This cluster consists of three genotypes (RhSP05, RhSP06 and RhSP07), and the branch lengths of the dendrogram among these three genotypes are relatively larger, indicating a greater evolutionary distance between these strains. All of the genotypes within this cluster include both historical and newly isolated strains. Genotype RhSP05 includes 16 Rhizobium sp. strains, isolated from a wide spectrum of host plants, isolated from 1973 to 2018 – Pisum sativum, Vicia faba, Lupinus sp., Galega sp., Glycine max, Medicago sativa, Lotus sp., Trifolium sp. Genotype RhSP07 is evolutionarily the furthest from the other two genotypes within Cluster II, including 13 strains, identified as Rhizobium sp., with identical full-length 16S rRNA genes. Only two of these strains are newly isolated in 2016 from Trifolium sp. The rest of the strains are from the historical Rhizobia Collection, isolated from 1963 until 2014, from Phaseolus vulgaris, Vicia faba, Lupinus sp., Medicago sativa, Galega sp., Pisum sativum, Glycine max, and Trifolium sp.
Figure 4. Alpha-proteobacterium, isolated from legume nodules in Latvia, full-length 16S rRNA phylogenetic tree, constructed using maximum likelihood method with JTT matrix and gamma rate distribution. Bootstrap values ≥70 is given in nodes. The scale indicates the number of nucleotide substitutions in the position. The genotypes used as representative sequences are indicated with the GenBank accession numbers in brackets. The number of identical strains within the genotype is indicated in italics. The circle next to the genotype indicates the time period when the strains in the particular genotype have been isolated.

Cluster XI combines three genotypes (RhL01, RhL02, RhL03), as well as the reference sample Rhizobium leguminosarum (MRDL01000029). All samples of this cluster were identified as R. leguminosarum. RhL01 is the most represented genotype – it includes 31 rhizobia strains isolated from a wide range of host plants (Pisum sativum, Vicia faba, Lathyrus pratensis, Lotus sp., Phaseolus vulgaris, Vigna unguiculata, Medicago sativa, Trifolium sp., Ononis arvensis). This genotype was identified in the NBCI database as R. leguminosarum bv. trifolii and R. leguminosarum bv. viciae with equally high confidence – 100% sequence similarity. Strains of this genotype were isolated in 2016, 2018 and 2019. Genotype RhL02 combines six rhizobia strains, all identified as R. leguminosarum bv. viciae (100% sequence identity) and R. leguminosarum bv. trifolii (99% sequence identity). Unlike the RhL01 genotype, which includes only newly isolated strains, this genotype is represented by both – strains from the historical Rhizobia Collection (isolated from peas, 1964) and newly isolated strains (isolated from peas and clover, 2016).
Full-length 16S rRNA gene phylogenetic analysis of the beta-proteobacteria class strains
Bacterial strains identified as belonging to the β-proteobacteria class () form a dendrogram that can be divided into 5 different clusters. In total, 14 bacterial strains in this study were identified as β-proteobacteria (Burkholderia sp. and Paraburkholderia sp.), isolated from Glycine max (8 strains) and Vicia faba (5 strains) and one strain isolated from Medicago sativa, in the period from 2016 to 2019. Cluster II includes the reference strain Paraburkholderia nodosa (AM284971) and genotype BSP07 (Burkholderia sp.). The strain (RS2030/19) belonging to this genotype was isolated in 2019 in a pot trial, in which strain RS2030 (identified as Mesorhizobium sp., Table S2) was used to inoculate Glycine max seeds. In turn, bacterial strain RS2041 (isolated in 2018, from G. max) was used for G. max seed inoculation in a pot experiment in 2019. Nodules from this experiment were collected and bacterial strain isolated (RS2041/19). In this study it was determined that both of these strains are identified as Paraburkholderia terricola, with identical full-length 16S rRNA genes, belonging to the Cluster V.
Figure 5. Beta-proteobacterium, isolated from legume nodules in Latvia, full-length 16S rRNA phylogenetic tree, constructed using maximum likelihood method with JTT matrix and gamma rate distribution. Bootstrap values ≥70 is given in nodes. The genotypes used as representative sequences are indicated with the GenBank accession numbers in brackets. The scale indicates the number of nucleotide substitutions per position. All identified genotypes have been isolated from various host plants from 2016 to 2019. The number of identical strains within the genotype is indicated in italics.

Full-length 16S rRNA gene phylogenetic analysis of the gamma-proteobacteria class strains
Bacterial strains identified as γ-proteobacteria (), form a dendrogram with seven clusters. In total, 28 bacterial strains are identified as Enterobacter sp. (incl. E. asburiae and E. ludwigii), Pseudomonas sp. (incl. P. frederiksbergensis), Rahnella aquatillis, Serratia plymuthica, and Pantoea agglomerans. These strains are isolated from a wide host plant spectrum (Glycine max, Trifolium sp., Medicago sativa, Pisum sativum, Vicia faba, Melilotus officinalis, Vicia cracca, Lupinus sp.). The most represented host plant within this proteobacteria class is Glycine max (eight strains in total). All of these bacterial strains are newly isolated strains in 2016–2019.
Figure 6. Gamma-proteobacterium, isolated from legume nodules in Latvia, full-length 16S rRNA phylogenetic tree, constructed using maximum likelihood method with JTT matrix and gamma rate distribution. Bootstrap values ≥70 is given in nodes. The genotypes used as representative sequences are indicated with the GenBank accession numbers in brackets. The scale indicates the number of nucleotide substitutions per position. Genotypes marked with asterisks (*) have been detected as chimeric sequences according to GenBank. All identified genotypes have been isolated from various host plants from 2016 to 2019. The number of identical strains within the genotype is indicated in italics.

Bacteria-host plant specificity
In order to determine the specificity of the host plant and the identified bacteria, the diversity of bacteria isolated from the nodules of the specific host plant was evaluated (). Bacterial strains isolated from Vicia faba showed the highest taxonomic diversity, compared to other host plants – out of thirteen examined genera, ten were detected; 35 bacterial strains isolated from V. faba were identified by molecular genetic methods, of which 20 strains were identified as Rhizobium sp. Of these strains, 10 belong to the genotype RhL01 (Rhizobium leguminosarum; Table S2) – all of these strains are newly isolated (2016–2019). Two of the genotypes (RhSP06 and RhSP07), that were isolated from V. faba, include only strains from the historical Rhizobia collection, isolated in 1972 and 1973. Bacterial strains isolated from V. faba were also identified as Bradyrhizobium sp. (1 strain), Rahnella aquatilis (2 strains), Serratia plymuthica (2 strains), Pantoea agglomerans (1 strain), Achromobacter sp. (1 strain), Paenibacillus sp. (2 strains), Paraburkholderia sp. (2 strains) and Burkholderia sp. (3 strains). All bacterial strains identified as Burkholderia sp. were isolated from one trial field. All bacterial strains that do not belong to the α-proteobacteria class, are bacterial strains isolated between 2016 and 2019. An exception is one strain (RV06902) isolated in 1970 and identified as Paenibacillus polymyxa.
Table 1. Bacterial genera diversity isolated from commonly grown host-plant nodules.
Bacterial strains isolated from Glycine max showed the second highest taxonomic diversity – out of thirteen examined genera, eight were detected; 26 bacterial strains isolated from G. max were identified by molecular genetics methods, of which nine belong to the α-proteobacteria class. Six strains were identified as Rhizobium sp., belonging to two closely related genotypes – RhSP05 and RhSP07 (; Table S2). One strain was identified as Rhizobium gallicum, two as Mesorhizobium sp. (belonging to two various genotypes), and only one strain was identified as Bradyrhizobium japonicum. Seven strains isolated from G. max nodules belong to the β-proteobacteria class: two were identified as Burkholderia sp. (both genetically different from each other), while the remaining four strains – Paraburkholderia terricola. Ten identified strains belong to the γ-proteobacteria class: two identified as Pseudomonas sp. (genetically different), one as Pantoea agglomerans, and seven as Enterobacter sp. All identified Enterobacter sp. strains were isolated in 2019 and are genetically different.
Significantly smaller taxonomic diversity could be observed for the rest of the examined host plants. In the case of Medicago sp, out of 13 examined genera, six were detected: from 13 identified strains, 7 were Rhizobium sp. (belonging to various genotypes), one Azospirillum sp, one Paraburkholderia sp., one Pseudomonas sp., one Rahnella aquatilis, and one Paenibacillus sp. Three bacteria genera out of 13 were detected for both – Pisum sativum and Trifolium sp.
Phosphorus content in soil samples
In this study soil samples were collected while isolating new bacteria strains from legume nodules, and phosphorus levels (P2O5) were analysed. Data were grouped and analysed by (1) the host plants extracted from the given soil sample and (2) the identified bacterial species/genera isolated from nodules of those host plants, assessing and comparing the variability of the data within each of these groups. It should be noted that when collecting new bacteria strains from nodules, the aim was to supplement the already existing rhizobia bacteria collection with strains isolated from a wider range of host plants, as well as to cover a wider territory of Latvia, so each group (host plants and identified bacteria) does not contain the same number of samples. Nevertheless, it is possible to compare the soil phosphorus levels between the researched bacteria genera.
The amount of phosphorus (P2O5) in soil samples varied from 33 mg to 835 mg kg−1 (; Table S3). Overall, large data distribution was observed, with results being grouped both by host plants and by identified bacteria. The smallest data variability, according to identified bacterial strains, can be observed in soil samples of Rhizobium gallicum and Serratia plymuthica variants. The largest data variability is observed in the Rhizobium sp. group. Soil samples collected together with the roots of Vicia faba show both the lowest and highest phosphorus levels. The smallest data distribution can be observed for Rhizobium gallicum and Serratia plymuthica while the largest data distribution is observed in the Rhizobium sp. group.
Figure 7. Phosphorus (P2O5) content in soil samples (mg kg−1). The coloured result bars show data distribution within the particular host plant (A) and identified bacteria genus/species (B) groups. Line (-) indicates data median value; cross (x) indicates date mean value; bar whiskers indicate the max and min values; data outliers are marked with a coloured circle.

Discussion
Full-length 16S rRNA gene – the appropriate marker for unknown bacteria identification
Knowledge about the location of the variable and conserved regions of the 16S rRNA gene sequence could justify gene fragment sequencing for the identification purposes, without sequencing the complete 16S rRNA gene. However, it should be noted that selecting a gene fragment with a very high degree of variability might overestimate the diversity of these bacteria (Singer et al. Citation2016). While the variable and conserved 16S rRNA gene regions for α- and β-proteobacteria genotypes () are consistent with the data shown in literature, the variable regions of γ-proteobacteria () often overlap with the conserved regions indicated in the literature (Singer et al. Citation2016).
Various studies show which of the 16S rRNA gene hypervariable regions gene are most often used for bacterial taxonomic affiliation identification, as well as for the phylogenetic analyses (Browman et al. Citation2015; Rosselli et al. Citation2016; Wagner et al. Citation2016; Pootakham et al. Citation2017; Earl et al. Citation2018), but no information is available on the genetic differences in these variable regions between different genera of rhizobia. In recent years, bacterial 16S rRNA gene hypervariable regions have been increasingly used in microbiome studies. However, there is no consensus among scientists which of these regions to use (Hassler et al. Citation2022). One of the reasons could be the differences in variable regions between various bacterial genera (Singer et al. Citation2016), which can also be observed in the results of this study comparing hypervariable and conserved regions between different classes of bacteria (). Results of our study support the use of full-length 16S rRNA gene sequencing for bacteria identification and phylogenetic analysis, especially for bacteria isolated from legume nodules with unknown class affiliation.
Host plant and bacterial specificity
The rhizobia-host plant specificity differed between α-proteobacteria clusters. Cluster II and IX (each combines three genotypes), identified as Rhizobium sp. and Rhizobium leguminosarum, have been isolated from the widest range of host plants, indicating low host specificity of these genotypes. The highest host specificity is demonstrated by strains from Cluster IV (Sinorhizobium sp., host plant Lotus sp.), Cluster VI (Mesorhizobium sp., host plant Glycine max) and Cluster VIII (Neorhizobium galegae, host plant Galega sp.) (; ). N. galegae is known to have a high host plant specificity, with some strains forming nodules solely on Galega species (Österman et al. Citation2014). Contrary to α-proteobacteria, strains identified as β-proteobacteria showed a greater host plant specificity – seven out of 14 strains were isolated from G. max, six – from Vicia faba and one from Medicago sativa (). Moreover, G. max was the only host plant for strains in Clusters I and II, while V. faba was the only host plant for strains in Cluster III (). Similar to Latvia, Glycine max is a relatively new crop in Venezuela, where it was identified that Burkholderia is the dominant bacteria that forms nodules on G. max roots. Moreover, these Burkholderia sp. strains showed high nitrogen-fixing efficiency in acidic soil (pH 4.5) (Ramirez et al. Citation2019). Phylogenetic analysis of γ-proteobacteria did not show host-plant specificity for particular clusters, as the genotypic fragmentation was greater within this class – most of the genotypes included mostly single bacterial strain (). However, it can be observed that all of the Enterobacter sp. strains were isolated from G. max (). A study assessing the diversity of bacteria strains nodulating Vigna unguiculata, using 16S rRNA gene sequencing, resulted in a high bacterial diversity – α-proteobacteria (incl. Rhizobium, Bradyrhizobium, Ensifer, Mesorhizobium genera) and β-proteobacteria (incl. Burkholderia), as well as Enterobacter (γ-proteobacteria). It was shown that the bacterial diversity was not affected so much by the host plant, as by the soil type. Some of the bacteria from α- and β-proteobacteria classes showed the ability to form nodules and fix atmospheric nitrogen, while other isolated strains were not capable to nodulate legumes by themselves. Nevertheless, most of these strains were shown to participate in nitrogen-fixation, improving plant growth (Leite et al. Citation2017). Similar studies have been conducted, researching various host-plants. A recent study determined a high bacterial diversity harbouring Medicago spp. root nodules, including such α-rhizobia as Sinorhizobium/Ensifer, Rhizobium, Mesorhizobium, as well as strains representing other bacterial classes. This study proved the plant growth promoting abilities of these strains, including the phosphate-solubilisation ability of genera that have been identified also in our study (Table S2), such as Paenibacillus sp. and Ensifer sp. (Martinez-Hidalgo et al. Citation2022). Supporting that the idea of β- and γ-proteobacteria, as well as Paenibacillus sp. strain inclusion in a commercial legume inoculum could be a significant investment in a sustainable agriculture practice.
Taxonomic diversity of nodule harbouring bacteria
In this study, bacterial strains isolated from various legume nodules were identified belonging to four bacterial classes – α-, β- and γ-proteobacteria, as well as Bacilli. As the study included both – strains from a historical Rhizobia Collection (1962–2015), as well as newly isolated strains (2016–2019), a question was raised whether strains isolated from the historical collection and newly isolated strains would show differences in taxonomic diversity. It was observed that most α-proteobacteria phylogenetic tree clusters contain both newly isolated strains and historical strains (). However, when comparing α-proteobacteria genotypes, a much wider taxonomic diversity can be observed among the newly isolated bacterial strains, compared to the historical strains. Bacterial strains isolated from 1962 to 1982 represent 5 genetically different genotypes out of a total of 19 identified genotypes; in turn – newly bacterial strains (2016–2019) represent 18 different α-proteobacteria genotypes.
The results obtained in this study can help interpret previously obtained results, where bacterial strains from the LBTU Rhizobia Collection were used for legume inoculation. For example, in one study soya been inoculation efficiency was compared between bacterial strains RS2081 (genotype BSP06), RS00301 (genotype RhSP07), as well as a commercial soya seed rhizobium inoculum BASF HiStick® (Petrēvics Citation2021). No significant effect on soya growth could be observed for either of the LBTU Rhizobia Collection strains, compared with the commercial inoculum that showed a slightly higher efficiency. By identifying the taxonomic affiliation of these strains, it is now known that the RS00301 strain corresponds to the genus Rhizobium, while the RS2081 strain is identified as Paraburkholderia terricola (Table S2). Mesorhizobium sp., as well as Bradyrhizobium japonicum (such as corresponding genotypes MhSP01, MhSP02 and BhJ01; Table S2) are known to be more effective for soybean inoculation (Pongsilp Citation2012).
Relatively many studies in Latvia researching rhizobia-legume symbiosis, have used LBTU Rhizobia Collection strains RP023, RV407, RV408, RV501 and RV505. The following rhizobia strains in this study were identified as the respective genotypes: RhL02; RhSP06; RhSP07; RhSP05; RhSP07 (Table S2; ). According to the 16S rRNA gene sequencing results, strains RV408 and RV505 are included in the same genotype, which could explain studies in which these strains do not show significantly different efficacy. Compared to these genotypes, genotype RhL02 that includes strain RP023, is genetically the most distant (). Studies that include strains RP023 and RV407, which are significantly genetically different, according to the phylogenetic analysis of the full-length 16S rRNA gene, differences in the efficiency of seed inoculation could be observed more often (Dubova et al. Citation2015a, Citation2015b; Dubova Citation2020; Šenberga et al. Citation2017). In turn, no difference in inoculation efficiency could be observed between the strains RV407 and RV505 (Dubova et al. Citation2016), which now are known to be closely related according to the 16S rRNA gene phylogenetic analysis (). Regarding to the rest of the identified bacteria classes in this study, six out of seven Paenibacillus sp. genotypes are solely strains from the historical Rhizobia Collection. No strains from the historical Rhizobia Collection were identified as β- or γ-proteobacteria (Table S2). Overall, strains isolated from Latvian soils in this study from 2016 to 2019, show higher taxonomic richness within all three – α-, β- and γ-proteobacteria classes.
Up until recently, only rhizobia identified as α-proteobacteria, were considered nitrogen-fixing symbiotic bacteria. In this study 65% of all identified strains were identified as α-proteobacteria. Nonetheless, the overall bacterial diversity was greater – strains representing α-, β-, γ-proteobacteria and Bacilli classes were detected (, Table S2). Increasing number of studies, researching rhizobia diversity, observe such genetic diversity, often dividing these bacteria into rhizobia and non-rhizobia endophytes (Rahal and Chekireb Citation2021). The identification of bacterial strains from β-proteobacteria, isolated from legume nodules, is a relatively new discovery (since the early 2000s) compared to about a hundred years of research on α-rhizobia bacteria, and over-turned the long-held belief that bacteria only from the α-proteobacteria class were able to form nodules on legume roots (Chen et al. Citation2003). Moreover, until the year 2000, bacteria from Burkholderia (β-proteobacteria) genus were considered as not only plant, but also animal and human pathogens. Therefore, the discovery of this genus as potential rhizobia, was very surprising (Moulin et al. Citation2001; Chen et al. Citation2003; Bontemps et al. Citation2010). Also, these findings raised new questions as to whether the symbiosis between β-proteobacteria and legumes is an extremely rare phenomenon or has simply gone unnoticed until now (Chen et al. Citation2003). This discovery changes the scientific point of view about the symbiosis between legumes and rhizosphere bacteria, expanding the understanding of these plant growth promoting bacteria, leading to new discoveries to improve nitrogen-fixation efficiency and enhance legume growth (Angus and Hirsch Citation2010).
The ability to convert atmospheric nitrogen (N2) into ammonia (NH3) and to form symbiotic relationships with legumes is not only possessed by bacteria recognised as rhizobia but has also been observed in bacteria belonging to other genera, referred as non-rhizobia endophytes (e.g. Azotobacter, Bacillus, Enterobacter, Pseudomonas, Serratia, Burkholderia and Azospirillum) (Mora et al. Citation2014; Perez-Montano et al. Citation2014).
Bacteria that belong to Pantoea (including Pantoea agglomerans identified in this study; Table S2), Bacillus, Enterobacter, Paenibacillus and Pseudomonas genera have been previously isolated from legume nodules (Martinez-Hidalgo and Hirsch Citation2017; Österman et al. Citation2014; Johnson et al. Citation2019). Serratia sp. together with rhizobia have previously improved nodule formation – enhancing the number of nodules, as well as enhancing the size of nodules, improving legume biomass and root system (Naveed et al. Citation2014). Some bacterial strains from these genera were also identified in our study – Paenibacillus polymyxa, Paenibacillus peoriae, Enterobacter ludwigii, Enterobacter asburiae, Pseudomonas frederiksbergensis, Serratia plymuthica and Azospirillum sp. (Table S2). Many of these bacteria promote plant development by solubilising phosphate in the rhizosphere, making it available for the plant. Rhizobium, Burkholderia, Serratia, Pseudomonas, Rahnella (including R. aquatillis, identified in our study; Table S2), Bacillus, Pantoea, Enterobacter are known for their phosphate solubilisation abilities, with Pseudomonas at the forefront (Kim et al. Citation1997; Shoebitz et al. Citation2009; Saidi et al. Citation2013; Zineb et al. Citation2020). Some Pseudomona species (P. striata, P. fluorescens, P. putida, P. trivialis, P. maltophilia, P. jessenii), when used to supplement rhizobium inoculum, have been shown to increase the nodulation efficiency, resulting in higher legume yield, as well as improved root/shoot length, nitrogenase activity and nitrogen fixation efficiency (Naveed et al. Citation2014). In our study most strains, identified as Pseudomonas sp., were identified only at the genus level, with one exception – P. frederiksbergensis, isolated from Melilotus officinalis (Table S2).
The role of γ-proteobacteria, and in many cases β-proteobacteria, in legume seed inoculation is still under debate. In a study where both γ- and β-proteobacteria were tested for their ability to nodulate black locust (Robina pseudoacacia) roots, Burkholderia sp. (β-proteobacteria), Pseudomonas sp. (γ-proteobacteria) and Paenibacillus sp. (Bacilli) were isolated from the nodules. Inoculation with the Paenibacillus sp. strain, that was previously isolated from surface sterile nodules, did not result in nodulation, indicating that this was a ‘guest’ strain together with the nodule-forming rhizobia bacteria strain (Shiraishi et al. Citation2010). These results suggest that the seven Paenibacillus sp. strains isolated in our study (Table S2) could have also been ‘guest’ strains in nodules, formed by either α-, β- or γ-proteobacteria strains. Apart from the fact that that all of the Paenibacillus sp. strains were identified from the historical Rhizobia Collection (although with no initial genetic identification carried out), these strains have been historically tested for nodulation and nitrogen fixation ability in terms of crop yield quality and quantity (data not published). However, even if these Paenibacillus sp. strains do not form nodules on legume roots, they are known to have plant growth promoting abilities (Goel et al. Citation2002; Vessey Citation2003) including nitrogen fixation (Perez-Montano et al. Citation2014). Therefore, the interaction between rhizobia and Paenibacillus sp. strains should be further studied, for instance, as a potential rhizobia co-inoculant. Due to the bacterial diversity (α-, β-, γ-proteobacteria, Bacilli), legume nodules have been suggested to act as a unique ecological niche that is capable of harbouring, as well as promoting, any compatible soil microorganisms (Saidi et al. Citation2013; De Meyer et al. Citation2015). Meaning, that bacteria from α-proteobacteria should not be the only ones used for legume seed inoculation, but the inoculum should include strains from various bacterial classes (Kawaka et al. Citation2018). However, there is no scientific consensus yet formed on this matter.
Potential phosphate-solubilising bacteria strains
The average total amount of phosphorus in the soil, indicated in literature, is 200–1500 mg kg−1 – most of it in a form that is not observed by plants. Certain soil bacteria are able to dissolve soil phosphates, making phosphorus available for the plant. These bacteria are defined as phosphate solubilising bacteria (PSB) and they increase the bioavailability of the insoluble soil phosphorus for plants (Alori et al. Citation2017). This could be one of the reasons why the distribution of phosphorous results was so high – bacteria (e.g. R. gallicum, Sinorhizobium sp., as well as some of the R. leguminosarum strains) could have successfully dissolved phosphorus compounds, making phosphorus available for the plants, resulting in a lower amount of soil phosphorus; compared with other soil samples, where bacteria were not able to dissolve phosphate, resulting in higher phosphorus level results. Analysing the results according to the sample collection sites, one can ascertain the phosphate solubilising potential of individual strains. Samples isolated in the field trials in Grobina Municipality in 2019 from V. faba (Table S1), show differences in the amount of phosphate between samples. Samples RV19/P5, RV19/P4 and RV19/P6 (Burkholderia sp., Paraburkholderia caledonica and Rhizobium leguminosarum; Table S2) show relatively low soil phosphorus (34.50–49.83 mg kg−1). However, the remaining identified samples in this field trial – RV19/K5, RV19/K12, RV19.3/5 and RV19/K10 (Burkholderia sp., Achromobacter sp., Rahnella aquatillis and Pantoea agglomerans; Table S2), were isolated from soil samples containing relatively higher amount of phosphorus (192.50–419.00 mg kg−1; Table S3). Both identified Burkholderia sp. strains belong to the same genotype, indicating that the phosphate-solubilising capacity of bacterial strains may differ within one genotype.
In literature, potential PSB, belonging to the α-proteobacteria class, are considered R. leguminosarum, Bradyrhizobium japonicum, Sinorhizobium/Ensifer; β-proteobacteria class – Burkholderia sp.; while γ-proteobacteria – Serratia, Rahnella, Pantoae sp., as well as Pseudomonas sp. (Saidi et al. Citation2013; Naveed et al. Citation2014; Zineb et al. Citation2020; Ibrahim et al. Citation2022; Martinez-Hidalgo et al. Citation2022). In addition, Pseudomonas sp. have shown the highest phosphate-solubilising potential (Zineb et al. Citation2020; Timofeeva and Sedkykh Citation2022). In this study, a proportion of Pseudomonas sp. strains have been isolated from plants grown in soil with a relatively low (below 200 mg kg−1; Table S3) phosphorus content. The specific bacterial strains could be used for further testing to research their phosphate-solubilising potential. Enterobacter ludwigii (γ-proteobacteria class), identified also in our study (Table S2), isolated from Glycine max, have previously shown the ability to dissolve calcium triphosphate, increasing the amount of phosphorous in the rhizosphere available for host plants, improving the above-ground mass and height of Lolium perenne, as well as increasing the root system by 50% (Shoebitz et al. Citation2009). Enterobacter in combination with rhizobia have shown to improve the formation of legumes, as well as increasing the yield (Naveed et al. Citation2014). In addition, also Paenibacillus sp. strain, isolated from Medicago sp., has shown phosphate-solubilising capacity (Martinez-Hidalgo et al. Citation2022).
Conclusions
The study confirmed that the full-length 16S rRNA gene is an appropriate marker for the genetic identification of bacteria isolated from legume nodules, and especially for phylogenetic analyses. This marker is particularly useful in studies where unknown rhizosphere bacteria are identified to detect the diversity of bacteria harbouring legume nodules.
The results of this study demonstrate that Latvian soil, and more precisely – legume nodules, contain a wide taxonomic diversity of bacterial genera. Bacteria strains from α-, β-and γ-proteobacteria, as well as Bacilli classes were identified. These nodules contain not only rhizobia bacteria that are necessary for nodulation initiation, but also other plant growth-promoting bacteria, known as non-rhizobia endophytes. Until now, there have been no non-rhizobia bacterial strains isolated and identified from legume nodules in Latvia, nor there are similar legume nodule harbouring bacteria diversity studies available in the neighbouring counties with similar climatic conditions (Estonia or Lithuania). In addition, the results indicate a phosphate-solubilising potential of several identified strains, that needs to be examined in future studies.
Author contribution
The funders had no role in the design of the study; in the collection, analyses, or interpretation of data; in the writing of the manuscript; or in the decision to publish the results.
Acknowledgements
We thank the personnel at the LBTU, Institute of Soil and Plant Sciences for their assistance in sample collection. We thank LBTU Research Laboratory of Biotechnology, The Division of Molecular Biology and Microbiology for the provided laboratory space and molecular biology equipment.
Disclosure statement
No potential conflict of interest was reported by the author(s).
Data Availability Statement
Sequencing data presented in this study are openly available in GenBank database referring to the accession numbers provided in Table S2.
Additional information
Funding
Notes on contributors
A. Klūga
A. Klūga is a PhD degree candidate at the Latvia University of Life Sciences and Technologies (LBTU), Faculty of Agriculture. She received a master's degree in biology from the University of Amsterdam in Amsterdam, Netherlands. She is currently working in LBTU Faculty of Agriculture as a lecturer and as a researcher in various research projects concerning legumes. She is interested in legume-rhizobia interactions as well as rhizobia and other soil microorganism role in sustainable agriculture.
L. Dubova
Dr. L. Dubova is a docent and lead scientist at the Latvia University of Life Sciences and Technologies (LBTU), Institute of Soil and Plant Sciences. Dr. Dubova has received her Ph.D. degree in agriculture from LBTU, Faculty of Agriculture, Jelgava, Latvia. Laila Dubova is currently working at LBTU Institute of Soil and Plant Sciences as a lecturer and as a researcher in various projects. She is interested in soil microbiology and the beneficial effects of mycorrhiza and rhizobia bacteria in sustainable agriculture.
I. Alsiņa
Dr. I. Alsiņa has received her Ph.D. degree in biology from University of Latvia, Faculty of Biology, Riga, Latvia. She is currently a professor and lead scientist at the Latvia University of Life Sciences and Technologies, Institute of Soil and Plant Sciences (Jelgava, Latvia), working as a lecturer, as well as a researcher in various projects. Her scientific interests include plant physiological and horticultural topics, such as non-destructive methods; greenhouse light quality; legume-rhizobia interactions; sustainable agriculture.
N. Rostoks
Dr. N. Rostoks is professor and program director at the Faculty of Biology, University of Latvia, Riga, Latvia. Dr. Rostoks has over 20 years of experience in plant genetics, genomics, genetic engineering, and biotechnology. He is currently the director of Latvian Biomedical Research and Study Centre (Riga, Latvia). He also has 8 years of experience in the European Food Safety Authority GMO Panel that authorizes GMOs for food and feed uses, and cultivation in the EU.
Notes
1 EU Common Agricultural Policy: https://bit.ly/2t0GM1E.
2 European Green Deal: https://bit.ly/418wRpY.
References
- Alori ET, Glick BR, Babalola OO. 2017. Microbial phosphorus solubilization and its potential for use in sustainable agriculture. Front Microbiol. 8:971. doi:10.3389/fmicb.2017.00971.
- Angus AA, Hirsch AM. 2010. Insights into the history of the legume-betaproteobacterial symbiosis. Mol Ecol. 19(1):28–30. doi:10.1111/j.1365-294X.2009.04459.x.
- Bontemps C, Elliott GN, Simon MF, Dos Reis Junior FB, Gross E, Lawton RC, Neto NE, de Fatima Loureiro M, de Faria SM, Sprent JI, James EK, Young JPW. 2010. Burkholderia species are ancient symbionts of legumes. Mol. Ecol. 19(1):44-52. doi:10.1111/j.1365-294X.2009.04458.x.
- Browman B, Kim B, Cho YJ, Korlach J. 2015. Long-Read, single-molecule, real-time (SMRT) DNA rivera sequencing for metagenomic applications. In: Izard J, Rivera MC, editor. Metagenomics for microbiology. London: Elsevier; p. 25–38. doi:10.1016/B978-0-12-410472-3.00002-6.
- Chen WM, Moulin L, Bontemps C, Vandamme P, Béna G, Boivin-Masson C. 2003. Legume symbiotic nitrogen fixation by β-proteobacteria is widespread in Nature. J Bacteriol. 185(24):7266–7272. doi:10.1128/JB.185.24.7266-7272.2003.
- De Meyer S, De Beuf K, Vekeman B, Willems A. 2015. A large diversity of non-rhizobial endophytes found in legume root nodules in Flanders (Belgium). Soil Biol Biochem. 83:1–11. doi:10.1016/j.soilbio.2015.01.002.
- Dubova L, Alsina I, Sergejeva D, Šenberga A. 2015a. Rhizobium leguminosarum un Glomus sp. effect on faba bean growth (in Latvian: Rhizobium leguminosarum un Glomus sp. ietekme uz cūku pupu augšanu). In Latvijas Lauksaimniecības universitātes Lauksaimniecības fakultātes, Latvijas Agronomu biedrības un Latvijas Lauksaimniecības un meža zinātņu akadēmijas zinātniski praktiskās konferences “Līdzsvarota Lauksaimniecība” raksti. p. 121–126.
- Dubova L, Šenberga A, Alsiņa I, Sergejeva D. 2016. Evaluation of the efficiency of the symbiotic system in bean (Vicia faba L.) crops (in Latvian: Simbiotiskās sistēmas efektivitātes izvērtējums pupu (Vicia faba L.) sējumos). Ražas svētki “Vecauce – 2016: Lauksaimniecības zinātne nozares attīstībai”, rakstu krājums. p. 20–23. ISBN: 9789984482408.
- Dubova L, Šenberga A, Alsiņa I. 2015b. The effect of double inoculation on the broad beans (Vicia faba L.) yield quality. Res Rural Dev. 1:34–39.
- Dubova L. 2020. Effects of symbiotic associations on bean (Vicia faba L.) productivity (in Latvian: Simbiotisko asociāciju ietekme uz pupu (Vicia faba L.) produktivitāti) [PhD thesis]. Latvia University of Agriculture. p. 126
- Earl JP, Adappa ND, Krol J, Bhat AS, Balashov S, Ehrlich RL, Palmer JN, Workman AD, Blasetti M, Sen B, et al. 2018. Species-level bacterial community profiling of the healthy sinonasal microbiome using Pacific Biosciences sequencing of full-length 16S rRNA genes. Microbiome. 6. doi:10.1186/s40168-018-0569-2.
- Goel AK, Sindhu SS, Dadarwal KR. 2002. Stimulation of nodulation and plant growth of chickpea (Cicer arietinum L.) by Pseudomonas spp. antagonistic to fungal pathogens. Biol Fertil Soils. 36:391–396. doi:10.1007/s00374-002-0554-5.
- Hassler HB, Probert B, Moore C, Lawson E, Jackson RW, Russell BT, Richards VP. 2022. Phylogenies of the 16S rRNA gene and its hypervariable regions lack concordance with core genome phylogenies. Microbiome. 10:104. doi:10.1186/s40168-022-01295-y.
- Ibrahim M, Iqbal M, Tang YT, Khan S, Guan DX, Li G. 2022. Phosphorus mobilization in plant–soil environments and inspired strategies for managing phosphorus: a review. Agronomy. 12:2539–2022. doi:10.3390/agronomy12102539.
- Janda JM, Abbott SL. 2007. 16S rRNA gene sequencing for bacterial identification in the diagnostic laboratory: pluses, perils, and pitfalls. J Clin Microbiol. 45(9):2761–2764. doi:10.1128/JCM.01228-07.
- Johnson JS, Spakowicz DJ, Hong BY, Petersen LM, Demkowicz P, Chen L, Leopold SR, Hanson BM, Agresta HO, Gerstein M, et al. 2019. Evaluation of 16S rRNA gene sequencing for species and strain-level microbiome analysis. Nat Commun. 10:5029. doi:10.1038/s41467-019-13036-1.
- Kawaka F, Makonde H, Dida M, Opala P, Ombori O, Maingi J, Muoma J. 2018. Genetic diversity of symbiotic bacteria nod-ulating common bean (Phaseolus vulgaris) in western Kenya. PLoS One. 13(11):e0207403. doi:10.1371/journal.pone.0207403.
- Kim KY, Jordan D, Krishnan HB. 1997. Rahnella aquatilis, a bacterium isolated from soybean rhizosphere, can solubilize hydroxyapatite. FEMS Microbiol Lett. 153:273–277. doi:10.1016/S0378-1097(97)00246-2.
- Leite J, Fischer D, Rouws LFM, Fernandes-Júnior PI, Hofmann A, Kublik S, Schloter M, Xavier GR, Radl V. 2017. Cowpea nodules harbor non-rhizobial bacterial communities that are shaped by soil type rather than plant genotype. Front Plant Sci. 7:2064. doi:10.3389/fpls.2016.02064.
- Martinez-Hidalgo P, Hirsch AM. 2017. The nodule microbiome: N2-fixing rhizobia do not live alone. Phytobiomes J. 1:70–82. doi:10.1094/PBIOMES-12-16-0019-RVW.
- Martinez-Hidalgo P, Humm EA, Still DW, Shi B, Pellegrini M, de la Roca G, Veliz E, Maymon M, Bru P, Huntemann M, et al. 2022. Medicago root nodule microbiomes: insights into a complex ecosystem with potential candidates for plant growth promotion. Plant Soil. 471:507–526. doi:10.1007/s11104-021-05247-7.
- Mora Y, Díaz R, Vargas-Lagunas C, Peralta H, Guerrero G, Aguilar A, Encarnación S, Girard L, Mora J. 2014. Nitrogen-fixing rhizobial strains isolated from common bean seeds: phylogeny, physiology, and genome analysis. Appl Environ Microbiol. 80:5644–5654. doi:10.1128/AEM.01491-14.
- Moulin L, Munive A, Dreyfus B, Boivin-Masson C. 2001. Nodulation of legumes by members of the β-subclass of prote-obacteria. Nature. 411:948–950. doi:10.1038/35082070.
- Naveed M, Mehboob I, Hussain MB, Zahir ZA. 2014. Perspectives of rhizobial inoculation for sustainable crop production. In: Arora NK, editor. Plant microbes symbiosis: Applied facts. New Dehli: Springer India; p. 209–239. doi:10.1007/978-81-322-2068-8_11.
- Österman J, Marsh J, Laine PK, Zeng Z, Alatalo E, Sullivan JT, Young JPW, Thomas-Oates J, Paulin L, Lindström K. 2014. Genome sequencing of two Neorhizobium galegae strains reveals a noeT gene responsible for the unusual acetylation of the nodulation factors. BMC Genom. 15:500. doi:10.1186/1471-2164-15-500.
- Perez-Montano F, Alias-Villegas C, Bellogin RA, del Cerro P, Espuny MR, Jiménez-Guerrero L, López-Baena FJ, Ollero FJ, Cubo T. 2014. Plant growth promotion in cereal and leguminous agricultural important plants: From microorganism capacities to crop production. Microbiol Res. 169:325–336. doi:10.1016/j.micres.2013.09.011.
- Petrēvics P. 2021. The effect of symbiosis on soybean yield and its formation in the farm SIA “Ezermaļi-3” (in Latvian: Simbiozes ietekme uz sojas ražu un tās formēšanos zemnieku saimniecībā SIA „Ezermaļi-3”). Studentu un maģistrantu zinātniskās konferences Daudzveidīga lauksaimniecība, Jelgava.
- Pongsilp N. 2012. Phenotypic and genotypic diversity of rhizobia. Nakhon Pathom: Bentham eBooks. p. 194
- Pootakham W, Mhuantong W, Yoocha T, Putchim L, Chutima S, Chaiwat N, Thongtham N, Tangphatsornruang S. 2017. High resolution profiling of coral-associated bacterial communities using full-length 16S rRNA sequence data from PacBio SMRT sequencing system. Sci Rep. 7:2774. doi:10.1038/s41598-017-03139-4.
- Rahal S, Chekireb D. 2021. Diversity of rhizobia and non-rhizobia endophytes isolated from root nodules of Trifolium sp. growing in lead and zinc mine site Guelma, Algeria. Arch Microbiol. 203(7):3839–3849. doi:10.1007/s00203-021-02362-y.
- Ramirez MDA, Espana M, Aguirre C, Kojima K, Ohkama-Ohtsu N, Sekimoto H, Yokoyama T. 2019. Burkholderia and paraburkholderia are predominant soybean rhizobial genera in Venezuelan soils in different climatic and topographical regions. Microbes Environ. 34(1):43–58. doi:10.1264/jsme2.ME18076.
- Rosselli R, Romoli O, Vitulo N, Vezzi A, Campanaro S, de Pascale F, Schiavon R, Tiarca M, Poletto F, Concheri G, et al. 2016. Direct 16S rRNA-seq from bacterial communities: a PCR-independent approach to simultaneously assess microbial diversity and functional activity potential of each taxon. Sci Rep. 6:32165. doi:10.1038/srep32165.
- Saidi S, Chebil S, Gtari M, Mhamdi R. 2013. Characterization of root-nodule bacteria isolated from Vicia faba and selection of plant growth promoting isolates. World J Microbiol Biotechnol. 29(6):1099–1106. doi:10.1007/s11274-013-1278-4.
- Saleh MA, Zaman S, Kabir G. 2013. Yield response of black gram to inoculation by different Rhizobium strains using various types of adhesives. Asian J Biol Sci. 6(3):181–186. doi:10.3923/ajbs.2013.181.186.
- Šenberga A, Dubova L, Alsiņa I, Strauta L. 2017. Rhizobium sp. – a potential tool for improving protein content in peas and faba beans. Rural Sustain Res. 37(332):2–9. doi:10.1515/plua-2017-0001.
- Shiraishi A, Matsushita N, Hougetsu T. 2010. Nodulation in black locust by the Gammaproteobacteria Pseudomonas sp. and the Betaproteobacteria Burkholderia sp. Syst Appl Microbiol. 33:269–274. doi:10.1016/j.syapm.2010.04.005.
- Shoebitz M, Ribaudo CM, Pardo MA, Cantorec ML, Ciampia L, Curáb JA. 2009. Plant growth promoting properties of a strain of Enterobacter ludwigii isolated from Lolium perenne rhizosphere. Soil Biol Biochem. 41:1768–1774. doi:10.1016/j.soilbio.2007.12.031.
- Singer E, Buchnell B, Coleman-Derr D, Browman B, Bowers RM, Levy A, Gies EA, Chen JF, Copeland A, Klenk HP, Hallam SJ, Hugenholtz P, Tringe SG, Woyke T. 2016. High resolution phylogenetic microbial community profiling. ISME J. 10(8):2020–2032. doi:10.1038/ismej.2015.249.
- Sleator RD. 2011. Phylogenetics. Arch. Microbiol. 193:235-293. doi:10.1007/s00203-011-0677-x.
- Tampakaki AP, Alsina I, Ntatsi G. 2014. Working with rhizobia. In: Marques G, Tampakaki AP, Alsina I, editor. Working with microbial symbioses of legumes: Handbook of protocols. p. 10–34. Deliverable D3.1, FP7 Research Project no. 613781, Portugal (Vila Real), Greece (Athens), Latvia (Jelgava). https://www.lf.llu.lv/sites/lf/files/2017-01/Eurolegume%20D3%201%20-%20Handbook%20of%20protocols.pdf.
- Timofeeva A, Sedkykh S. 2022. Prospects for using phosphate-solubilizing microorganisms as natural fertilizers in agriculture. Plants. 11(16):2119. doi:10.3390/plants11162119.
- Vessey JK. 2003. Plant growth promoting rhizobacteria as biofertilizers. Plant Soil. 255:571–586. doi:10.1023/A:1026037216893.
- Wagner J, Coupland P, Browne HP, Lawley TD, Francis SC, Parkhill J. 2016. Evaluation of PacBio sequencing for full-length bacterial 16S rRNA gene classification. BMC Microbiol. 16:274. doi:10.1186/s12866-016-0891-4.
- Wagner SC. 2011. Biological nitrogen fixation. Nat Educ Knowl. 3(10):15. http://www.nature.com/scitable/knowledge/library/biological-nitrogen-fixation-23570419.
- Wang ET, Chen WT, Tian CF, Young JPW, Chen WX. 2019. Symbiosis between rhizobia and legumes. In: Wang ET, Tian CF, Chen WF, Young JPW, Chen WX, editor. Ecology and evolution of rhizobia. Singapore: Springer; p. 3–19.
- Zineb AB, Trabelsi D, Ayachi I, Barhoumi F, Aroca R, Mhamdi R. 2020. Inoculation with elite strains of phosphate-solubilizing bacteria enhances the effectiveness of fertilisation with rock phosphates. Geomicrobiol J. 37:22–30. doi:10.1080/01490451.2019.1658826.