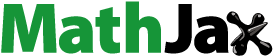
ABSTRACT
Laser-based additive manufacturing (LBAM) has shown great potential in the development of new metallic materials, especially in the design and fabrication of metal matrix composites (MMCs). Steel matrix composites (SMCs) as one MMC-type, have been successfully additively manufactured with full density and good performance. This article reviews emerging studies of LBAM-fabricated SMCs, starting from the methods of feedstock preparation including respective merits and challenges. The mechanisms of phase transformation, grain growth and texture development of the steel matrix, as well as the precipitation of reinforcements during rapid solidification inherent to LBAM are demonstrated. Microstructural features of SMCs with different matrix (austenitic, martensitic, duplex and ferritic) and reinforcement types are discussed. The interrelationship between the composition and physical properties of the composite powder, microstructures and mechanical properties of SMCs are disclosed and the involved strengthening mechanisms are discussed. Lastly, conclusions and outlook focusing on emerging trends of LBAM-fabricated SMCs are presented.
Introduction
Fe-based materials, particularly steels, play an irreplaceable role in today’s global economy, due to the availability of ample resources, relatively low cost and favourable mechanical properties [Citation1,Citation2]. As a response to the industrial demand for even stronger and, particularly, more wear-resistant Fe-based materials, steel matrix composites (SMCs) have been developed [Citation3]. Owing to the introduction of reinforcements, such as intermetallic compounds, carbides, nitrides, oxides, and borides into the steel matrix, SMCs demonstrate the required mechanical properties needed in service as structural materials for example, in aeronautics, defense and automobile industries [Citation4–9]. SMCs profit from the advantageous properties of the hard reinforcing phase and the ductile steel matrix [Citation10]. The fabrication of SMCs is a very effective measure to further enhance the strength, hardness, wear resistance, and fatigue life of the steel components [Citation11–13].
Synthesis of SMCs having homogeneous microstructures with uniformly distributed reinforcing particles is vital to fully exploit their strengthening potential [Citation14]. This task is challenging and cannot be realized easily, because large attractive van der Waals forces, which act between the small-sized reinforcing particles, prevent their uniform distribution within the metal matrix [Citation15]. Traditional preparation methods of SMCs, mainly encompassing casting and powder metallurgy, cannot ensure the uniform distribution of reinforcements in the steel matrix and are generally also time-consuming [Citation16]. Furthermore, there are also investigations reporting on the rapid growth of reinforcing phases during conventional preparation of SMCs adversely affecting the desired strengthening effect [Citation17]. Expensive casting moulds and the coarse-grained microstructure of the resulting SMCs as well as limited interfacial bonding between the reinforcing particulates and the matrix have additionally restricted the application of SMCs [Citation18].
Those deficiencies highlight the necessity for a more suitable processing route of high-performance SMCs with controllable microstructure and uniformly distributed reinforcements. Laser-based additive manufacturing (LBAM) is a popular processing technology enabling the fabrication of complex-shaped components from powder materials in a layer-by-layer manner [Citation19]. So far, LBAM has been utilized for the successful fabrication of high-performance iron-based alloys, such as 316L stainless steel [Citation20,Citation21] and maraging steel [Citation22,Citation23]. LBAM permits to fabricate not only components with complicated shape, but also with designed microstructures and functions. Thus, LBAM also bears great potential for the preparation of novel functional materials, such as shape memory materials [Citation24–31]. LBAM enables the manufacturing of multi-functional and high-performance metallic components, significantly expanding its applicability. In recent years, LBAM has been successfully employed to the fabrication of metal matrix composites (MMCs) with superior mechanical properties, including Al- and Ti-based lightweight MMCs [Citation32–34] as well as high-strength Fe-based MMCs [Citation17]. The fabrication process of MMCs via LBAM consists of feedstock deposition, melting, fusion and binding of successive material layers [Citation35] and the feedstock materials can be powder blends consisting of pre-alloyed powder and reinforcements. This approach entails the synthesis of multicomponent materials with performance-adapted microstructures [Citation19]. By proper selection of the material type, shape and size, as well as volume fraction of the reinforcements, the microstructures of MMCs could be designed according to required property profiles [Citation36]. Among these MMCs, the microstructure of Al-based MMCs always consists of mostly ceramic reinforcing particles and the face-centred cubic (FCC) Al-matrix, regardless of the type, volume fraction and particle size of reinforcements as well as the applied LBAM processing conditions [Citation37–44]. In contrast to Al-based MMCs, the Ti matrix in Ti-based MMCs can undergo an allotropic transformation from α to β-Ti and can also form martensite [Citation45–47], which is influenced by the involved alloying elements, like α- or β-Ti stabilizer, as well as the LBAM processing conditions [Citation47]. However, the reinforcements do not generally affect the phase formation of the Ti-based matrix, and instead, they only change the thermodynamic behaviour of the molten pool, such as the temperature gradient, solidification rate or cooling rate, and influence the grain size and texture features of the Ti-based matrix [Citation48–51]. This is in stark contrast to SMCs. As we show in this article, the phase composition of the steel matrix strongly depends on the addition of reinforcements in the SMCs fabricated by LBAM. In addition to the LBAM processing conditions, the selection of the matrix/reinforcement material as well as the morphology and content of reinforcement dictates the type of the matrix, which can be austenitic, martensitic, ferritic or a mixture of them [Citation52]. Furthermore, the addition of reinforcements greatly influences the rapid solidification process and melt flow behaviour of the molten pool during LBAM processing of SMCs, thus affecting the grain growth and texture development of the steel matrix [Citation53]. Thus, not only the high hardness and high strength of the reinforcement, but also its effect on the phase transformation and microstructural evolution of the steel matrix can be utilized to design high-performance SMC via LBAM. So far, there are no works summarizing on the influencing mechanisms of different kinds of reinforcements on the phase transformation, microstructural evolution and mechanical properties of LBAM-prepared SMCs.
The mostly used LBAM technologies for processing steel composite powders are laser powder bed fusion (LPBF), laser directed energy deposition (LDED), and powder bed laser sintering (PBLS). Due to their unique processing, which is characterized by repetitive cycles of ultrafast heating immediately followed by rapid cooling, the resulting SMCs show distinctly different microstructure and mechanical properties than their counterparts produced by conventional methods. The technology roadmap for the development of high-performance SMC components via the above-mentioned LBAM techniques is similar and visualized in . Obtaining feedstock with high processability is the foundation for the successful fabrication of high-quality SMC parts via LBAM. Currently, no feedstock of steel matrix composite powder is commercially available for LBAM. Suitable feedstock preparation methods, which depend on the matrix material and kind, shape, size and content of reinforcement(s), with high efficiency and acceptable cost should be proposed. The composition and physical properties of the steel composite powder should be well designed, since it determines its LBAM-processability. For different composite powder classes, the LBAM-processing parameters (e.g. laser power, laser scanning speed, powder feed rate, hatching styles and layer thickness) should be optimized to fabricate defect-free SMCs [Citation54–59]. Afterwards, the microstructure analysis and performance test should be conducted for the LBAM-fabricated SMCs. By manipulating the microstructure evolution of the steel matrix (e.g. phase transformation, grain growth, texture development) and precipitation behaviour of the reinforcements (e.g. morphology, size, and distribution of precipitates and matrix/precipitate interface), multiple strengthening and toughening mechanisms can be triggered in the fabricated SMCs. The ultimate objective is the fabrication of high-performance SMCs via LBAM. By evaluating the integrated effect between material, processing, microstructure and performance, feedback of the interaction between the material- and process-related parameters on the microstructure and performance of SMCs can be generated. This feedback allows researchers to further optimize the composition and physical properties of the composite powder as well as the identification of the optimum LBAM-processing parameters. In this way, the mechanisms of tailoring the microstructure and properties of LBAM-fabricated SMCs can be proposed. A review elaborating on LPBF of SMCs with focus on process optimization for the fabrication of defect-free and high-performance SMC components, was very recently published [Citation60]. In order to complete the technology roadmap for the development of high-performance LBAM-ed SMCs, a holistic description of the influencing mechanisms of different kinds of reinforcements on the microstructural evolution and mechanical properties of SMCs is provided in this review. For currently existing review articles addressing LBAM of lightweight MMCs or Fe-based alloys, the readers are referred to literature [Citation61–63].
Figure 1. The common technology roadmap for the development of high-performance SMC components for LBAM-processing.

The purpose of the present review article is to comprehensively review existing studies related to LBAM of SMCs with the aim to (1) demonstrate the design principle of SMC powder for LBAM and give an overview on the preparation methods of the SMC feedstock, (2) illuminate the influencing mechanisms of different kinds of reinforcements on the microstructure of SMCs fabricated via LBAM, (3) provide a comprehensive description about the microstructural characteristics and mechanical properties of typical LBAM-prepared SMCs and (4) indicate upcoming trends. Compared to the existing reviews, in this work, we focus on the physical mechanisms involved during the feedstock preparation process of SMC powder and the rapid melting/solidification process of the SMC melt as well as during the deformation of the LBAM-fabricated SMCs. At first, the selection of the reinforcement for different kinds of steel matrix and the preparation methods of SMCs feedstock for LBAM is described. Then, the effect of processing conditions and reinforcements on the phase transformation and microstructural evolution of the steel matrix during LBAM is illuminated. Subsequently, the microstructures and mechanical properties of LBAM-fabricated SMCs consisting of different matrix microstructure and reinforcements are elaborated and compared to each other. The involved strengthening mechanisms are elucidated to provide a deeper understanding of the mechanical performance of LBAM-fabricated SMCs and how it can be adjusted. Lastly, a summary and concluding remarks on the findings of this review are presented followed by addressing upcoming trends in LBAM of performance-adapted SMCs.
Feedstock preparation
Preparation of matrix powder
Powder mixtures, which consist of the pre-alloyed matrix powder and reinforcing particles, serve as feedstock for LBAM of SMCs. In order to ensure a high processing quality of LBAM-fabricated SMCs parts, the sphericity, size distribution, apparent and tapped density of the matrix powder are very important factors, since they can significantly affect the flowability and spreading property of the SMCs powder mixture. Currently, main preparation approaches applied to produce steel powder for LBAM include water, gas, plasma, and centrifugal atomization techniques [Citation64]. Amongst them, the plasma atomization technique can produce spherical powders with high purity, but it however faces challenges, such as the high production cost and the existence of residual pores within the powder particles which limits its attractiveness for potential suppliers [Citation65]. The centrifugal atomization technique can produce powders with an almost perfect sphericity, an excellent flowability and the absence of agglomeration or contamination [Citation66]. However, the problems of high cost and high difficulty to produce powders with a particle size below 100 μm should be considered [Citation66]. Gas atomization and water atomization are the most used techniques to produce steel powder showing proper flowability and particle size distribution for processing via LBAM at a reasonable cost. By comparison, the gas-atomized powder demonstrates higher sphericity and is thus more suitable for serving as the matrix powder for the LBAM-fabrication of SMCs [Citation64].
a illustrates the fundamental working principle of the gas atomization powder preparation technique. When the gas stream impinges the metal liquid at high velocity, the flow energy of the gas is transformed into melt surface energy which divides it into small droplets. Next, those droplets gradually obtain a spherical shape due to the surface tension, and ultimately finely spherical metal powder results by fast solidification under convection heat dissipation [Citation67]. Various inert gases such as nitrogen, helium or argon are widely utilized to produce high-grade metal powder via gas atomization [Citation66]. As shown in b, 316L SS powder produced by this approach exhibited spherical shape [Citation68]. In addition, powder possesses homogeneous microstructure and good packing ability. The preparation of powder by water-atomization is more cost-efficient than by gas atomization [Citation66], but the respective particles display more irregular shape, as shown in c [Citation68]. Furthermore, their surface is easily oxidized during water-atomization.
Figure 2. (a) Working principle of gas atomization for powder preparation; (b) morphologies of 316L stainless steel powder particles fabricated by gas atomization and (c) water atomization. Reproduced with permission from [Citation67,Citation68].
![Figure 2. (a) Working principle of gas atomization for powder preparation; (b) morphologies of 316L stainless steel powder particles fabricated by gas atomization and (c) water atomization. Reproduced with permission from [Citation67,Citation68].](/cms/asset/8eb8e9b7-f4dc-49c9-870a-a115fdab72eb/yimr_a_2258664_f0002_oc.jpg)
The metal powder quality must be high and consistent, since it dictates the powder delivery into the building chamber and the packing of the powder vital for powder bed fusion and powder feeding of the direct energy deposition process. Thus, the powder quality determines the so-called in-process printing dynamics [Citation69]. In spite of recent research efforts and advances in the powder preparation technology, the formation of powder particle defects, which are mainly internal porosity and satellite features, cannot be circumvented at the moment. visualizes both defect types which adversely affect the quality and reliability of the resulting components fabricated by LBAM. The evolution of both defect types can be described by the liquid break-up mechanism effective during gas atomization. schematically illustrates the mechanisms for the formation of (a) internal pores and (b) satellites during gas atomization. At the very early stage of gas-atomization, the metallic liquid is easily broken-up into droplets which become mushy at high gas velocities in the early stage of solidification [Citation70]. In this stage, a melt fragment (or large droplet) spreads along the direction perpendicular to the gas flow and subsequently bulges parallel to the gas flow, so that the melt fragment takes the shape of bag-type sheet (a). Afterwards, this sheet shatters into finer droplets due to surface tension. If unsuitable temperature and/ or gas flow conditions are met, the bag-type melt fragment can alternatively collapse and will form a hollow drop (or sphere) in which atomized gas will be entrapped [Citation70] (a). The second main defect type of gas-atomized powder are satellites and their formation mechanism can be classified into three types [Citation71] (b): Firstly, larger solidified particles can collide with the smaller semi-solid droplets in the atomization cone mainly at the upper and middle parts of the atomization chamber. Once the smaller droplets impinge on the already solidified larger particles, they wet, then spread and finally solidify on the surface of these larger particles. Secondly, the larger droplets are semi-solid, collide with the smaller solidified particles and they penetrate the larger semi-solid droplets at varying angles. This process can be mainly observed in the atomization cone at the top of the chamber. In the third case, semi-solid droplets with varying diameters are formed in the atomization cone. Smaller droplets advance in the atomization chamber at higher velocities than the larger ones. Owing to their differing velocities, the smaller and larger droplets collide and subsequently solidify. In order to minimize the occurrence of those defects, the gas atomization parameters, such as gas atomizing manifold pressure, gas-to-mass flow, and pouring temperatures, must be optimized to provide powders with high quality suited to LBAM-processing. For more information concerning the effect of gas atomization parameters on the powder quality, the reader is referred to Mandal et al. [Citation64].
Figure 3. Different types of defects including (a) satellites and (b) internal pores related to the gas-atomization-produced powder. Reproduced with permission from [Citation71].
![Figure 3. Different types of defects including (a) satellites and (b) internal pores related to the gas-atomization-produced powder. Reproduced with permission from [Citation71].](/cms/asset/21303fa0-a552-406f-8b01-e23095c22f35/yimr_a_2258664_f0003_ob.jpg)
Figure 4. Schematic showing the formation mechanisms of (a) internal pores and (b) three different kinds of satellites during the process of gas atomization. Reprinted with permission from [Citation71,Citation72].
![Figure 4. Schematic showing the formation mechanisms of (a) internal pores and (b) three different kinds of satellites during the process of gas atomization. Reprinted with permission from [Citation71,Citation72].](/cms/asset/015b1ffc-81a7-4361-a694-e50ab801afee/yimr_a_2258664_f0004_oc.jpg)
Selection of reinforcements for LBAM of SMCs
The selection of reinforcements is a key factor for the design of SMCs with desired microstructure and performance. The addition of reinforcements strongly impacts the solidification conditions of the molten pool during LBAM processing and thus gives access for deliberate manipulation of the solidified microstructure and resulting mechanical properties of SMCs. In general, four factors need to be considered when reinforcements are selected: (1) laser absorptivity, (2) thermal conductivity and (3) coefficient of thermal expansion of the reinforcement(s) as well as (4) wettability between the reinforcing particles and the steel matrix.
The laser absorptivity strongly influences the heat absorption and Marangoni flow effective in the molten pool during LBAM-processing [Citation73]. Most ceramic reinforcements show higher laser absorptivity, such as TiC (0.43–0.94) [Citation74] and SiC (0.66–0.85) [Citation75], than the steel matrix particles (~0.47) [Citation76]. Additionally, the incorporation of small reinforcing particles increases the surface roughness of the powder mixture, leading to multiple reflections and increased absorption of the laser beam. Therefore, the addition of reinforcements to pre-alloyed steel powder generally results in SMC powder mixtures with increased laser absorptivity. Thus, higher temperatures can be achieved within the molten pool accompanied by intensified Marangoni flow during LBAM-processing facilitating the uniform distribution of reinforcements in the solidified microstructure [Citation77]. Too large laser absorptivity of the powder mixture can however cause overheating of the molten pool. If the steel is composed of e.g. elements with low melting point, they might evaporate ultimately increasing the difficulty of fabricating the desired microstructure [Citation60]. Therefore, the laser absorptivity of the reinforcing particles and the chemical composition of the steel powder should be considered when both are selected for the powder mixture which shall be processed by LBAM to SMCs.
The thermal conductivity of reinforcements is another highly influential factor which affects the solidified microstructure of the LBAM-fabricated SMCs. It influences the thermal conduction between the powder mixture and substrate, so that the solidification behaviour of the molten pool, such as temperature gradient, degree of supercooling and the Marangoni flow behaviour within the molten pool, is affected during LBAM-processing, which involves the evolution of the thermal stress, grain growth and texture development of the SMCs during the LBAM processing. When a reinforcement with high thermal conductivity, such as SiC (83.9 W m−1 K−1 at room temperature) [Citation78] in comparison to 316L stainless steel (14.7 W m−1 K−1 at room temperature) [Citation79], is selected, rapid heat conduction and dissipation will result and it diminishes the thermal stresses in the SMCs during LBAM [Citation80]. More importantly, the rapid thermal transfer stemming from the high thermal conductivity can lead to a larger temperature gradient in the molten pool. Thus, it is easier to show a cellular-growth pattern rather than equiaxed-dendrites-growth pattern in the solidified microstructure of LBAM-fabricated SiC reinforced SMCs. The detailed influencing mechanism of reinforcements on the grain growth of SMCs during the rapid solidification of LBAM will be discussed in ‘Grain growth’ section.
The third factor, which needs to be considered for the selection of reinforcements, is their coefficient of thermal expansion (CTE) which determines the thermal stress evolution in SMCs during LBAM. The larger the difference in CTE between the reinforcement and the steel matrix, the larger thermal stress will be induced in the SMC which increases the likelihood of cracking during the LBAM. Besides, the difference in the CTE between the reinforcement and steel matrix can also affect the austenite-martensite phase transformation within the steel matrix during the rapid solidification process of LBAM-processing. For example, duplex austenitic/martensitic matrices are easily formed in LBAM-fabricated 316L-based SMCs reinforced with TiC or SiC particles, due to the large difference in CTE between TiC(∼7 × 10−6/K at 1273 K [Citation81])/SiC(∼5 × 10−6/K at 1273 K [Citation82]) and 316L stainless steel (∼20 × 10−6/K at 1273 K [Citation83]). Strong tensile stresses formed in the SMCs caused by the large difference in CTE between TiC/SiC and the steel matrix triggers the stress-induced martensitic transformation and, thus, lead to the evolution of a duplex austenitic/martensitic matrix in LBAM-fabricated SMCs. Therefore, the CTE difference between reinforcements and steel matrix can be considered for designing the phase constitution of the steel matrix in the LBAM-fabricated SMCs. The influencing mechanisms of reinforcements on the phase transformation of the steel matrix during LBAM will be addressed more in detail in ‘Phase transformation’ section.
At last, the wettability between the steel matrix and reinforcing particles needs to be considered for their selection, since a good wettability between them is desired for obtaining a strong interface and hence strong SMCs. For example, there is a good wettability between WC particles and steel matrix and thus a strong reactive interface can form between them, due to a chemical reaction between the matrix and reinforcement during LBAM-processing [Citation53]. The strong bonding between reinforcements and matrix caused by the formation of the reaction interface contributes a significant load transfer effect to the SMCs, which greatly strengthens the mechanical properties of LBAM-fabricated SMCs [Citation84]. Therefore, it is necessary to choose a reinforcement that has good wetting behaviour with the matrix. A detailed description about the formation of the reaction interface between WC and steel matrix will be given in ‘Microstructural characteristics of LBAM-fabricated SMCs with different phase composition’ section.
Preparation methods of SMC feedstock powder
Currently, feedstock of steel matrix composite powder for processing via LBAM-technologies is commercially not available. Therefore, different preparation methods have been used to blend the steel powder with reinforcements for producing the composite powder. The preparation methods for the composite powder significantly affect its quality readily characterized by the powder morphology, flowability and dispersion degree of reinforcements. The feedstock preparation methods for steel matrix composite powder have been intensely studied. lists and compares the feedstock preparation methods for SMC powder which will be elaborated in this section.
Table 1. Methods for preparing steel matrix composite feedstock.
Direct mixing
The direct mixing method has been used to prepare SMC powders, as demonstrated by a high number of research groups, due to its characteristics of low cost, high efficiency and wide applicability [Citation85–87,Citation102,Citation103]. Reinforcements can be distributed around the steel matrix powder particles with unchanged morphology. Since the interaction force between raw materials is limited during direct mixing, this preparation method can ensure good flowability of the blended powder. One representative example is the directly mixed Al2O3/316L composite powder with a flowability of about 37.3 g s−1 which is similar with the one of 316L powder (∼42.8 g s−1) [Citation104]. However, it is challenging to disperse nanoscale reinforcing particles around the steel particles in a uniform manner. Agglomeration of nanoparticles during direct mixing would occur due to their high surface energy [Citation105]. Van der Walls’ and Coulomb’s forces are the driving force for agglomeration. Furthermore, the difference in density of the nanoparticles and metal matrix powder also poses a processing challenge. Compared to other methods, such as ball milling, the agglomeration effect during direct mixing usually results in inferior densification behaviour and mechanical properties of SMC parts, especially when the volume fraction of the reinforcements is large.
Ball milling
Another cost-effective processing method for the preparation of powder blends is ball milling which can avoid agglomeration of nanoparticles. This process involves repetitive deformation, fracture and cold-welding of the powder particles [Citation34,Citation106]. Thus, it is a non-equilibrium process and can be classified into several stages. Since the reinforcing particles are brittle in nature, they initially fracture prior to sticking onto the matrix powder. Meanwhile, the steel matrix powder particles plastically deform and mix with the fractured reinforcing particles. Subsequent cold welding of the matrix powder leads to finely dispersed reinforcement particles inside the cold-welded matrix powders. shows the procedure of ball milling of the TiC/316L powder mixture.
Figure 5. Schematic showing the procedure of ball milling of the TiC/316L powder mixture. Reprinted with permission from [Citation107].
![Figure 5. Schematic showing the procedure of ball milling of the TiC/316L powder mixture. Reprinted with permission from [Citation107].](/cms/asset/1bda85ce-08d0-4af8-a7c6-c26f58828588/yimr_a_2258664_f0005_oc.jpg)
Based on the degree of cold welding and subsequent deformation during ball milling, this process can be classified into low-energy ball milling and high-energy ball milling [Citation108]. illustrates the mechanism of powder mixing using low-energy ball milling (a) and high-energy ball milling (b). In powder metallurgy, the high-energy ball milling dominates due to the (i) high ball-to-powder ratio (ranging from 5:1 to 30:1), (ii) high mixing speed (ranging from 300 to 600 rpm) and (iii) long mixing time (ranging from 20 to 150 h) [Citation105,Citation109]. During the high-energy ball milling procedure, powders are repeatedly heavily deformed, fractured, and rewelded. As a result, the powders are mechanically alloyed accompanied by significant change in particle shape. Usually, the resulting powder shape is irregular which adversely affects the corresponding flowability. By contrast, nanoparticles can be uniformly distributed on the surface of the steel powder via low-energy ball milling. The steel particle shape remains spherical and, thus, still provides a sufficiently high flowability. Therefore, the low-energy ball milling method is able to prepare powder blends with uniform particle size distribution and good flowability, but without powder agglomerates. We point out that the reinforcing nanoparticles strongly adhere to the surface of the matrix particles with a uniform distribution via the low-energy ball milling process, as the work of Chen et al. [Citation17] demonstrated. They used the low-energy ball milling method to prepare WC/1.2767L (German DIN standard, 0.45 wt.% C, 1.35 wt.% Cr, 4.00 wt.% Ni, 0.25 wt.% Mo, 0.25 wt.% Si, 0.40 wt.% Mn, balance Fe) steel composite powder for LPBF-processing and the morphologies typical for the as-prepared powder blend are shown in . A Pulverisette 6 planetary mono-mill device (Fritsch GmbH, Germany) with a ball-to-powder weight ratio of 2:1 was used for milling which was performed for 4 h at a rotation speed of 200 rpm, in Ar-atmosphere. One can observe steel powder particles with surface being uniformly covered with submicron-sized WC particles. No fractured particles were visible (b). Uniformly distributed reinforcement particles inside the steel matrix are of high importance to the relative density and mechanical properties of the LPBF-fabricated SMCs. The difference in the distribution of reinforcements alters the sequence of the laser interaction between the laser and powder, thus influencing the molten pool and further physical processing [Citation34].
Figure 6. Mechanism of powder mixing by ball milling: (a) low-energy ball milling; (b) high-energy ball milling. Reprinted with permission from [Citation105].
![Figure 6. Mechanism of powder mixing by ball milling: (a) low-energy ball milling; (b) high-energy ball milling. Reprinted with permission from [Citation105].](/cms/asset/4510235c-45cd-4658-bc85-2fb5a1e09aa9/yimr_a_2258664_f0006_oc.jpg)
Figure 7. (a) SEM images illustrate powder particle morphologies typical for the as-prepared composite powder. (b) The magnified SEM image highlights the uniform distribution of WC-particles on the surface of the matrix powder. (c, d) EDS analysis confirms the submicron-sized particle to be WC. Reprinted with permission from [Citation17].
![Figure 7. (a) SEM images illustrate powder particle morphologies typical for the as-prepared composite powder. (b) The magnified SEM image highlights the uniform distribution of WC-particles on the surface of the matrix powder. (c, d) EDS analysis confirms the submicron-sized particle to be WC. Reprinted with permission from [Citation17].](/cms/asset/ee0810ac-2bad-4ce8-a723-9c9c1fc069db/yimr_a_2258664_f0007_oc.jpg)
A successful ball milling procedure is also determined by the densities of the steel powder and reinforcements. It is difficult to achieve a uniform mixing between steel powder and reinforcements if they show a large difference in density, for instance TiC and steel powder. In order to overcome this challenge, Zhang et al. [Citation110] investigated the alignment of the rotation axis on the mixing behaviour of the H13 steel and TiC powder which was subsequently used for processing via LDED. To be more precise, they used vertical as well horizontal ball milling and their results are presented in . It was found that vertical ball milling are not suitable for preparing uniform TiC/H13 powder blends regardless of the milling parameters (a,b). Using this arrangement, TiC particles could be neither distributed in a uniform manner, nor embedded in the H13 particles during ball milling. Because of the difference in the density of H13 and TiC particles, these two kinds of powder are further separated during the powder feeding process; subsequent laser deposition yields a poor deposition effect. By contrast, the reinforcing TiC-particles could be embedded inside the H13 powder, when the horizontal ball milling was used. One can observe an uneven surface of the particles and the presence of TiC on the surface of H13 particles (e), confirmed by the EDS analysis (f). Thus, alignment of the rotary axis of the ball milling device is crucial. The horizontal alignment allows to prepare powder blends from powders with huge difference in density, such as H13 steel and TiC.
Figure 8. Morphology of mixed TiC and H13 powders produced by vertical ball milling. (a) H13 particles were not uniformly covered by the reinforcing TiC particles; (b) magnified section of the SEM image in (a). (c) Mixed powders produced by horizontal ball milling and (d) corresponding magnified section of the SEM image shown in (c). (e) Section of a H13 particle after ball milling shows the presence of TiC as the corresponding EDS spectrum confirms (f). Reproduced with permission from [Citation110].
![Figure 8. Morphology of mixed TiC and H13 powders produced by vertical ball milling. (a) H13 particles were not uniformly covered by the reinforcing TiC particles; (b) magnified section of the SEM image in (a). (c) Mixed powders produced by horizontal ball milling and (d) corresponding magnified section of the SEM image shown in (c). (e) Section of a H13 particle after ball milling shows the presence of TiC as the corresponding EDS spectrum confirms (f). Reproduced with permission from [Citation110].](/cms/asset/22c0533c-f40e-4ea7-a24d-079484f28529/yimr_a_2258664_f0008_oc.jpg)
As discussed above, the major disadvantage of ball milling is the degradation in the flowability of the resulting powder blend. Therefore, optimization of the ball milling parameters, such as ball-to-powder weight ratio, rotation speed, and milling time, is important to the processability of the mixture. illustrates the optimized ball milling parameters for different combinations of steel powder and reinforcements. A ball-to-powder weight ratio of 5:1 and a mixing speed of 200 rpm are suitable for the preparation via ball milling of most SMCs powder mixtures. By contrast, the mixing duration generally varies for the different SMC powder mixtures, as the work of AlMangour et al. [Citation90] reported. They have investigated the microstructure and morphology of TiB2/316L stainless steel powder mixtures, which were prepared by ball-milling, as a function of the milling duration, at a constant ball-to-powder weight ratio of 5:1 as well as constant mixing speed of 200 rpm. depicts the corresponding SEM images visualizing the particle morphology and microstructural evolution of the milled powders dependent on the milling duration. The average particle diameter and corresponding particle size distribution dependent on the milling duration are displayed in . The duration of ball milling being tantamount to the application of mechanical alloying (MA) shows a moderate effect on the characteristics of the milled powders which can be characterized by the particle morphology, shape, and size distribution. Minor fracturing of the particles was observed at the initial stage of the ball-milling process (within the first 2 h). During the next stage (2–4 h), the ductile 316L powder particles underwent severe plastic deformation and cold-welding resulting in an increased particle size of 46.78 ± 20.50 μm. The corresponding particle size distribution could be characterized by: d10 = 33.71 μm, d50 = 42.23 μm, and d90 = 67.85 μm (). The occurrence of cold-welding, which led to overall larger particles, was also perceived on the powder particles surface. Further increase of the milling time to 6 h led to the formation of powder particle pieces originating from the fracturing process which started to prevail over cold-welding. Intense plastic deformation of the 316L particles accompanied by an increasing dislocation density and accumulated strain occurred during ball milling at this stage. Regions in the direct vicinity of the reinforcing particles posed thereby stress concentrators. Once a sufficient strain energy was accumulated, cracks were initiated and propagated until the particles fractured. The interplay between fracturing and cold-welding led to a homogeneous distribution of the reinforcing phase. The fractured particles showed slightly elongated appearance with partially oblong shape in comparison to the initial spherical particles (c). The respective particle size slightly decreased to 45.31 ± 16.8 μm, while there was no significant change in the particle size distribution (d10 = 34.1 μm, d50 = 42.4 μm, and d90 = 64.5 μm; see ) at this stage. After increasing the milling duration to 8 h, the milled powder mixture showed particles with considerably increased diameter, while most particles nearly retained their spherical shape (see inset in d). Distinctly less fractured particles were observed, so that the cold-welding mechanism became dominant again during this final stage of ball-milling. The corresponding particle size distribution (d10 = 34.02 μm, d50 = 43.69 μm, and d90 = 105.4 μm) perceptibly widened (). The rotational speed was kept constant throughout this experimental series at 200 rpm while increasing the ball-milling duration. Centrifugal forces are effective during this powder mixing procedure and they caused the reinforcing particles to uniformly disperse within the powder mixture while only minimal changes were induced in the particle morphology. Thus, the atomic diffusion effect of the reinforcing particles increased. Ball milling for even longer durations (≥8 h) will result in the formation of agglomerated particles due to cold-welding induced by the collisions of the grinding balls (characterized by high kinetic energies) with the powder particles. In order to ensure good flowability properties of SMCs powder and the mixing time should be properly selected with the aim to not only maintain the relative spherical morphology of the powder particles, but also obtain a uniform particle size distribution.
Figure 9. SEM images display characteristic microstructures of the TiB2/316L powders after (a) 2, (b) 4, (c) 6, and (d) 8 h of ball-milling. The inset of d emphasizes the particle morphology after 8 h of ball-milling. Reprinted with permission from [Citation90].
![Figure 9. SEM images display characteristic microstructures of the TiB2/316L powders after (a) 2, (b) 4, (c) 6, and (d) 8 h of ball-milling. The inset of Figure 9d emphasizes the particle morphology after 8 h of ball-milling. Reprinted with permission from [Citation90].](/cms/asset/d89de078-77b9-4141-9776-260aeabcf6de/yimr_a_2258664_f0009_ob.jpg)
Figure 10. Average particle size and particle size distribution of TiB2/316L mixture plotted as a function of the ball milling duration. Reprinted with permission from [Citation90].
![Figure 10. Average particle size and particle size distribution of TiB2/316L mixture plotted as a function of the ball milling duration. Reprinted with permission from [Citation90].](/cms/asset/3769b4a2-4f7c-43e6-b36d-68b247ef4cbd/yimr_a_2258664_f0010_oc.jpg)
Table 2. Ball milling parameters for different steel matrix composite feedstock.
Besides the combination of suitable milling parameters, additional pauses with defined duration are important to obtain a powder blend with high quality. Optimization of the pause-duration during ball-milling requires careful studies and differs for the specific powder blend, as reported in ref. [Citation111]. For CeO2/316L composite powder [Citation95], the milling process was usually paused for 15 min after every 15 min of milling, in order to avoid an excessive temperature rise in the interior of the grinding bowl. For TiC and TiB2/stainless-steel blends, the optimized procedures for milling and pause are generally set as 120+15 min- [Citation88] and 60+15 min-procedure [Citation90], respectively.
Ball milling + in situ reaction
In contrast to ex-situ composite powder, whereby the reinforcing particles were initially added to the steel powder, the reinforcements, which are synthesized through a chemical reaction among the starting elemental materials, show a good interface bonding with the steel matrix [Citation116]. The preparation of such in-situ composite powder requires fewer processing steps, thus resulting in a powder which is finer and, thermodynamically more stable. The microstructure of SMCs fabricated from this in-situ composite powder shows more uniformly distributed reinforcements within the steel matrix [Citation117–120]. Furthermore, the interface between the in-situ formed reinforcement and the steel matrix is generally more compatible, because gas adsorption or oxidation do not occur [Citation121]. Thus, the interfacial bonding is sufficiently strong to transfer the stress from the matrix to the reinforcement phases, ultimately yielding material fabricated by LPBF with improved mechanical properties [Citation122]. As a result, the LPBF-ed SMCs fabricated with in-situ milled powders often show better service properties. AlMangour et al. [Citation123] prepared 316L-based composite reinforced with TiC via an in-situ reaction method. The initial 316L steel powder particles were irregular in shape and about 44 μm in diameter. They were mixed with pure Ti powder particles, which had a polygonal shape with about 10 μm in diameter, and high-purity graphite powder with a particle size of 2–12 μm. Based on the microstructural observations of the LPBF-prepared composite, the authors observed well-bonded nano-sized TiC particles which were uniformly dispersed along the sub-grain boundaries within the grains of the 316L matrix. Song et al. [Citation96] utilized Cr-C-Fe in-situ reaction system to fabricate SMCs via LPBF. (Fe,Cr)23C6 carbides uniformly formed in the LPBF-fabricated SMCs, contributing to a higher hardness and enhanced tensile properties of the SMCs. The ‘ball milling + in situ reaction’ method allows researchers to prepare high-performance SMCs involving desired in-situ reaction between raw materials during LBAM, but necessitates very careful design of the reaction procedure [Citation124].
Agent deposition
Agent deposition is a fitting method to deposit carbon nanotubes (CNTs) or graphene on the surface of the steel matrix powder which allows one to prepare CNT/steel or graphene/steel composite powders. The use of CNTs and graphene as the reinforcement for composite material is very promising and attracts lots of attention [Citation125–127], since both have very high strength and elastic modulus. Furthermore, they also show superior electrical and thermal conductivities [Citation128–130]. However, there are serious challenges in preparing the composite powder. Both CNTs and graphene tend to agglomerate, because of the strong van der Waals attractive forces. One reported solution is the use of an additional agent, such as polyvinyl alcohol (PVA) solution which allows to disperse the CNTs or graphene [Citation131–133]. After subsequent drying and sieving, composite powder with CNTs/graphene distributed in a uniform fashion onto the surface of the steel powder particles can be obtained (see schematic in ). Laser sintering is then applied at lower laser power to process the CNT/graphene reinforced SMC powder, so that the majority of CNTs or graphene can be retained. It was shown that the reaction between carbon and the steel matrix can be significantly mitigated at lower laser powers [Citation98]. Lin et al. [Citation98] mixed micro-sized iron powder with multiwall carbon nanotubes (MWNTs) by using 4 wt.% of polyvinyl alcohol. Single MWNTs can be successfully separated by applying PVA as dispersing agent. The agent deposition method can also be applied to prepare multilayer-graphene/steel [Citation99] and graphene-oxide/steel [Citation100] composite powder.
Laser-aided dielectrophoretic deposition
Laser-aided dielectrophoretic deposition is an innovative method to prepare nano-sized-particles-reinforced steel matrix composite powder for LBAM processing. The corresponding composite powders display good flowability and strong bonding between nanoparticles and the steel powder. Doñate-Buendía et al. [Citation101,Citation134] prepared a nanocomposite powder consisting of Y2O3 and high chromium steel via laser-aided dielectrophoretic deposition. This preparation method is carried out in two steps. At first, the oxide nanoparticles are synthesized via laser fragmentation in liquids (LFL) [Citation135,Citation136], as schematically illustrated in a. Thereby, an initial suspension containing micro- or nanoparticles is irradiated to promote their deagglomeration and size reduction [Citation137,Citation138]. The technological configuration is known as passage reactor with a liquid jet of the initial suspension originating from the reactor and flowing towards a collecting vessel. The jet is exposed to a laser, which is focused through a cylindrical lens (a), to ensure uniform irradiation of the flowing colloid and also to reduce the processing time down to five passage cycles. Doñate-Buendía et al. obtained particles with a bimodal size distribution (258 particles were counted) from TEM investigations (b). In the second step, the nanoparticles are absorbed onto the surface of the steel powder particles by directly adding the steel powder to the dispersed colloidal nanoparticles. Controlling the pH-value during this addition is crucial (), since dielectrophoretic deposition can be achieved only when the pH-value is set between the isoelectric points (IEP) of Y2O3 (IEP = 7.5) [Citation139] and steel [Citation134] (IEP = 6). Stirring this mixture at the appropriate pH-value and subsequent drying in an oven concludes the deposition of the nanoparticles on the steel powder. In the present case, the mixture contained 0.08 wt% Y2O3 and was stirred for 2 h followed by drying in an oven at 50°C. Finally, Y2O3 nanoparticles were finely dispersed on the surface of the steel powder particles, as the shown in b,c.
Figure 12. (a) Schematic illustration of the passage reactor setup used for fragmenting the commercially purchased Y2O3-nanoparticles. (b) Transmission electron microscope (TEM) image of the Y2O3-nanoparticles obtained after five irradiation cycles. Reprinted with permission from [Citation134].
![Figure 12. (a) Schematic illustration of the passage reactor setup used for fragmenting the commercially purchased Y2O3-nanoparticles. (b) Transmission electron microscope (TEM) image of the Y2O3-nanoparticles obtained after five irradiation cycles. Reprinted with permission from [Citation134].](/cms/asset/c13f4cab-f5b5-40e5-9fd1-372832bc23a9/yimr_a_2258664_f0012_oc.jpg)
Figure 13. (a) Schematic representation of the pH-controlled adsorption process of the Y2O3-nanoparticles onto the steel powder. (b, c) SEM images of the obtained nanocomposite powder after the drying process, showing a good dispersion of Y2O3 on the steel particle surface. Reprinted with permission from [Citation134].
![Figure 13. (a) Schematic representation of the pH-controlled adsorption process of the Y2O3-nanoparticles onto the steel powder. (b, c) SEM images of the obtained nanocomposite powder after the drying process, showing a good dispersion of Y2O3 on the steel particle surface. Reprinted with permission from [Citation134].](/cms/asset/3598ab90-d188-4bc6-9513-d20f1cb72728/yimr_a_2258664_f0013_oc.jpg)
Microstructures
A comprehensive description encompassing the microstructural features of LBAM-fabricated SMCs is given in this section. Firstly, we disclose the effect of laser energy input on the microstructure formation of LBAM-fabricated SMCs. Then, the influencing mechanisms of the reinforcements on the phase transformation and microstructural evolution of the steel matrix are revealed. Subsequently, we dwell on the microstructural characteristics of these SMCs which basically consist of different steel matrix and reinforcement phases. In order to give the reader a structured overview, the SMCs are classified according to their main microstructural constituent(s) at room temperature. Different compositions lead to also different microstructural constituents of the steel-based materials. The austenitic and martensitic matrices are most often found in SMCs fabricated by LBAM. The duplex austenitic/martensitic matrix can be obtained provided there is a large difference in CTE between the reinforcement and the steel matrix, such as TiC/316L SMCs. The incorporation of the reinforcement leads to more complicated melting and solidification conditions during LBAM when compared to the steel counterpart. The underlying mechanism is urgently needed to be revealed for exploiting it as a method to deliberately manipulate the microstructure of LBAM-fabricated SMCs. In this section, the metallurgical characteristics and resulting microstructures of typical SMCs fabricated by LBAM are reviewed. It should be noted that the metal-ceramic gradient composites are not included. For the advances in additive manufacturing of metal-based functionally graded materials, the readers are referred to Reichardt et al.’s work [Citation140].
The effect of laser energy input on the microstructure of LBAM-fabricated SMCs
A consensus in literature is that the laser energy density is one of the most important factors for the microstructural evolution of LBAM-fabricated SMCs. A variety of laser energy density measures are used in literature with a volumetric density approach. The volumetric energy density (VED) may have the advantages that it can be normalized with the material enthalpy allowing the comparison of multiple materials and LBAM machines [Citation141]. The VED is more widely used for LPBF and PBLS techniques, which combines the four most influential processing parameters:
(1)
(1) where P is the laser power, v is the laser scanning speed, s is the hatch spacing and l is the layer thickness. Different VEDs cause different temperature gradients and Marangoni flow behaviours within the molten pool during LBAM processing which distinctly influences the solidified microstructure of the steel matrix and reinforcements. A low VED can be achieved by using fast laser scanning velocities which leads to an ultrafine microstructure of the steel matrix because of a high solidification rate in the molten pool. More importantly, the phase transformation of the steel matrix is susceptible to the applied VED for some SMCs such as TiC/316L SMCs. It has been reported that the formation of martensite in TiC/316L SMCs is associated with the cooling rate effective within the molten pool during LPBF-processing [Citation142]. There is a relationship between the cooling rate of the molten pool, ΔT/Δt, and the applied processing parameters including P and v [Citation143]:
(2)
(2) where αλ is the absorptivity at the laser wavelength λ, k is the thermal conductivity, ρ is the density of the metal, c is the specific heat, and d is the diameter of the molten pool. According to Equation (2), the cooling rate of the molten pool is proportional to the square root of the scanning speed at constant laser power. The utilization of a high laser scanning speed imparts large cooling rates in the molten pool during solidification. Large thermal stresses are additionally evoked and they promote the martensite transformation. Besides the steel matrix, the VED also influences the distribution of the reinforcement. The aggregation effect of TiC leads to a higher residual stress within the SMCs which facilitates the phase transformation from austenite to martensite. The exact distribution of the TiC reinforcing particles is determined by the applied VED and illustrates the corresponding mechanism. Various distribution states of the TiC nanoparticles can result and depend on the VED [Citation77]. In the laser-induced molten pool composed of the 316L melt and the partially molten TiC particles, a sharp temperature gradient forms between the edge and centre of the molten pool during LPBF-processing. This temperature gradient generates a surface tension gradient and causes capillary forces which drive the liquid to flow. This phenomenon is known as Marangoni flow [Citation144]. Whenever the capillary forces act on the TiC nanoparticles, a torque is generated around the particles. The torque causes the rotation of the TiC nanoparticles within the pool enabling their rearrangement and, hence, changes their distribution. If the VED is too low (67 J mm−3), both the temperature gradient and the associated capillary force intensity will be attenuated. Then the TiC-nanoparticles cannot be sufficiently rearranged within the molten pool. In this situation, the solidification rate of the liquid front is relatively high, which results in a limited Marangoni flow within the molten pool (a). As a result, the rearrangement of the TiC nanoparticles is impeded, and the distribution of reinforcements is very non-uniform (b). By contrast, a more intense rearrangement of the reinforcing TiC nanoparticles inside the molten pool can be achieved when a higher VED is applied (125 J mm−3), leading to an intensified Marangoni flow. The torque force gradually acts on the TiC nanoparticles, which congregate around the core of the Marangoni flow pattern resulting in the formation of a ring-like structure (c,d). A more intense VED (300 J mm−3) would hinder the uniform distribution of the TiC reinforcements. Although the converging flow pushes the TiC nanoparticles towards the centre, the collective impact of repulsion forces and the significantly intensified Marangoni flow leads to a clustered ring-like structure in the solidified matrix (e,f). Therefore, the proper selection of the VED for the fabrication of SMCs by LBAM is vital for obtaining the desired phase composition of the steel matrix and a uniform distribution of the reinforcement(s) in the SMCs.
Figure 14. Schematic illustrating the movement of TiC nanoparticles within the molten pool during LBAM-processing at various VEDs: (a) VED = 67 J mm−3; (b) VED = 125 J −3; and (c) VED = 300 J mm−3. Reproduced with permission from [Citation77].
![Figure 14. Schematic illustrating the movement of TiC nanoparticles within the molten pool during LBAM-processing at various VEDs: (a) VED = 67 J mm−3; (b) VED = 125 J −3; and (c) VED = 300 J mm−3. Reproduced with permission from [Citation77].](/cms/asset/161558f5-b946-4427-9422-2a787eb613a7/yimr_a_2258664_f0014_oc.jpg)
Influencing mechanisms of reinforcements on the phase transformation and microstructural evolution of the steel matrix during LBAM
Phase transformation
The fast-moving laser during LBAM heats the powder feedstock at very high heating rates resulting in the formation of a spatially very limited molten pool which subsequently solidifies also at high cooling rates. The high cooling rates result from the major heat extraction of this small pool through the previously solidified material and substrate favouring the prevailing martensitic transformation in steels during LBAM [Citation56]. Certain alloying elements in steels, such as C and Ni, stabilize the undercooled austenite and, hence, lower critical cooling rates are sufficient for the martensitic transformation [Citation145]. One suited example is the LPBF of WC/1.2767L SMCs [Citation17], whereby the W and C elements dissolve within the iron-based matrix during melting and significantly affect the phase transformation of the steel matrix between austenite and martensite during subsequent solidification. The solubility of WC particles within the Fe-based melt can be described by the Gibbs-Thomson capillarity effect [Citation146]:
(3)
(3) where M is the molecular weight of WC particle in the Fe-based solvent, T is the absolute temperature, ρ is the density of WC, σ is the interfacial tension between WC particle and Fe-based liquid, S1 and S2 are the solubilities of WC particles with radius r1 and r2 (r1 > r2) and R is the gas constant. Equation (3) indicates that the WC particles will be completely dissolved within the iron-based matrix, if their size is extremely small (submicron-sized or nano-sized [Citation147–149]). Both W and C elements reduce the martensite start temperature (Ms) and, hence, increase the volume fraction of the retained austenite within the iron-based matrix [Citation150]. With more WC incorporated into the steel matrix, more W and C elements dissolve within the iron-based matrix and further decrease the Ms even below the room temperature, thus resulting in more retained austenite, as shown in .
Figure 15. EBSD analysis shows the phase map and volume fraction of austenite and martensite within LPBF-fabricated (a) 1.2767 L tool steel and respective composites reinforced with (b) 2 wt% WC, (c) 4 wt% WC and (d) 6 wt% WC. Reprinted with permission from [Citation149].
![Figure 15. EBSD analysis shows the phase map and volume fraction of austenite and martensite within LPBF-fabricated (a) 1.2767 L tool steel and respective composites reinforced with (b) 2 wt% WC, (c) 4 wt% WC and (d) 6 wt% WC. Reprinted with permission from [Citation149].](/cms/asset/c6b0ebcc-0dd0-48ed-be83-dfdf470e6817/yimr_a_2258664_f0015_oc.jpg)
Another factor influencing the phase transformation between austenite and martensite is the difference in the CTE between the reinforcement and the steel matrix. If there is a large difference in CTE between the reinforcement and the steel matrix, such as for TiC/316L and SiC/316L SMCs, a strong tensile stress is generally induced within the γ-Fe matrix during LBAM-processing. This tensile stress then triggers the martensitic transformation and, thus, leads to the evolution of a duplex austenitic/martensitic matrix. The effect of TiC reinforcement on the phase formation of the steel matrix during laser processing has been elucidated by Axén and Zum Gahr [Citation151]. The TiC particles introduce tensile stresses into the matrix during the cooling stage of LPBF-fabrication, since they show a distinctly lower CTE than the iron-based matrix. Because of that, the martensitic transformation is promoted. Based on the latest publications [Citation88,Citation97,Citation123,Citation142,Citation152–154] on this topic, one can summarize that the transformation of γ-Fe to α-Fe in SMCs is strongly related to the TiC content. shows typical XRD patterns of the LPBF-fabricated TiC/316L SMCs samples reinforced with different volume fractions of nano-sized TiC particles [Citation88]. With the addition of TiC ranging between 2.5 and 5 vol.%, the composite samples consist of γ-Fe as the dominating matrix phase. Reflections for the TiC and α-Fe phases are only visible in the XRD patterns for SMCs with a reinforcing volume fraction of more than 10%. The reflections for α-Fe are intensified with increasing reinforcement content. Thus, the martensitic transformation can be induced with an increase in TiC content which enhances the thermal stresses within the steel matrix and triggers the stress-induced phase transformation.
Figure 16. XRD patterns of the 316L-based SMCs reinforced with different volume fractions of nano-sized TiC particles: (a) 2.5%, (b) 5%, (c) 10%, (d) 15%. Reprinted with permission from [Citation88].
![Figure 16. XRD patterns of the 316L-based SMCs reinforced with different volume fractions of nano-sized TiC particles: (a) 2.5%, (b) 5%, (c) 10%, (d) 15%. Reprinted with permission from [Citation88].](/cms/asset/1e95e3fe-9988-4547-ab64-cae408505060/yimr_a_2258664_f0016_ob.jpg)
Revealing the influencing mechanisms of reinforcements on phase transformation of the steel matrix during LBAM is of high interest, since one can exploit these effects to literally design SMCs with desired phase constitution and, hence deliberately modulate the mechanical properties of the LBAM-fabricated SMCs.
Grain growth
During the solidification process, the temperature gradient (G) and solidification rate (R) are the key parameters for controlling the grain growth pattern and resulting solidification structures. As illustrated in a, the solidification structures are related to the constitutional supercooling. In order to avoid constitutional supercooling, the solid–liquid (S/L) interface needs to satisfy the following equation [Citation155]:
(4)
(4) where ΔT is the solidification range, and DL is the diffusion coefficient of the liquid. A high G combined with a low R leads to a stable planar S/L interface. If G decreases and/or R increases, then the planar S/L interface will be replaced by the so-called cellular or dendritic solidification modes. As clearly illustrated in b,c, the solidification mode changes from planar to cellular, to columnar dendritic, and finally to equiaxed dendritic with increasing constitutional supercooling. During LPBF, the G and R can be varied within the solidified molten pool by incorporating reinforcements. One fitting example is SiC which has a much higher thermal conductivity (83.9 W m−1 K−1 at room temperature [Citation78]) than the steel matrix (14.7 W m−1 K−1 at room temperature for 316L steel [Citation79]); hence, R of the molten pool increases with an increasing SiC fraction. Therefore, the G/R value decreases when more SiC is added, which then gradually alters the solidification structures from cellular to dendritic [Citation156]. By contrast, the introduction of TiB2, which imparts a lower thermal conductivity to the steel matrix, can increase the G/R value, and thus leads to the evolution of a cellular substructure within the TiB2/H13 composite [Citation91].
Figure 17. (a) Schematic of the solidification front with velocity R, and (b) the effect of constitutional supercooling on the solidification modes; (c) The solidification map showing the effect of G and R on the morphology and size of solidification microstructure. Reprinted with permission from [Citation156,Citation157].
![Figure 17. (a) Schematic of the solidification front with velocity R, and (b) the effect of constitutional supercooling on the solidification modes; (c) The solidification map showing the effect of G and R on the morphology and size of solidification microstructure. Reprinted with permission from [Citation156,Citation157].](/cms/asset/4fdcf91b-c082-423c-a75b-8171f8d6dd96/yimr_a_2258664_f0017_oc.jpg)
As shown in the solidification map in c, the scale of the solidification structure can be predicted in terms of the cooling rate GR. For example, the secondary dendrite arm spacing of the columnar and equiaxed dendrites can be correlated with the cooling rate or solidification time, which can be expressed as [Citation157]:
(5)
(5) where tf is local solidification time, ϵC is cooling rate, and a, b and n are material specific constants. Equation (5) indicates that finer dendritic structures can be produced at higher cooling rate and shorter solidification time. By incorporating reinforcements with high thermal conductivity, like SiC, the molten pool of the SMCs undergoes a faster cooling process compared to the monolithic steel counterpart during LBAM. The faster the cooling rate during solidification is, the shorter the duration available for growth of dendrite arms and the smaller the dendrite arm spacing will be. As a consequence, LBAM-fabricated SMCs with finer microstructure result.
Next to the effect on the solidification rate of the molten pool, reinforcing particles can also provide a high number of low-energy-barrier heterogeneous nucleation sites for the matrix grains. This heterogeneous nucleation could reduce the critical undercooling needed to induce the growth of equiaxed grains. visualizes the solidification mechanism for the cellular substructure of LPBF-fabricated V8C7/316L SMCs [Citation92]. At first, a high-energy laser beam locally melts the powder mixture. Thereby, the laser beam exerts an effective pressure Peff on the sub-micron sized vanadium carbides, so that the flow inside the resulting molten pool facilitates their decomposition. Afterwards, a molten pool consisting of completely dissolved V8C7/316L powder blend results. Owing to the ultrafine particle size, high particle-surface activity and elevated heat-input provided by the laser source, the V8C7 constituent was easy to decompose entailing the diffusion V and C atoms into the 316L melt. The applied Peff not only exerted pressure on the vanadium carbide particles to accelerate their decomposition, but also enhanced their migration and more uniform dispersion within the molten pool. When the laser beam moved away from the molten pool, it solidified at very high cooling rates [Citation19]. Owing to the higher melting point of vanadium carbides (VCx), they firstly crystallized during cooling and acted as heterogeneous nucleation site for the austenite grains. Finally, after completion of crystallization, the microstructure showed larger-sized VCx reinforcements distributed along the subgrain boundaries and smaller VCx were retained within the subgrains (b,c). AlMangour et al. [Citation158] also reported similar ring-like-shaped substructures in LPBF-fabricated TiB2/316L SMCs. They observed the TiB2 nanoparticles formed along the subgrain boundaries of the 316L matrix. The degree of bonding between the TiB2 nanoparticles was enhanced with increasing volume fraction of the TiB2-reinforcements. The combined effects of the strong Marangoni convection and the repulsive forces between reinforcing particles account for the evolution of the homogenously distributed ring-like reinforcements [Citation159].
Figure 18. (a) Schematic illustrating the formation mechanism of LPBF-fabricated V8C7/316L SMCs and (b-c) the grain morphology of LPBF-fabricated V8C7/316L SMCs, and some tiny particles were formed inside the austenite grains (yellow arrows). Reprinted with permission from [Citation60].
![Figure 18. (a) Schematic illustrating the formation mechanism of LPBF-fabricated V8C7/316L SMCs and (b-c) the grain morphology of LPBF-fabricated V8C7/316L SMCs, and some tiny particles were formed inside the austenite grains (yellow arrows). Reprinted with permission from [Citation60].](/cms/asset/1f7413b7-c92f-4b5c-a25d-99bf6bdd0b25/yimr_a_2258664_f0018_oc.jpg)
Texture feature
The growth direction of grains is always along the building direction (BD) during the LBAM-process, since the maximum heat flux from the molten pool is extracted through the material towards the building plate [Citation160,Citation161]. In particular in the case of austenitic steel, e.g. 316L, the typical epitaxial columnar microstructure generally shows an extremely high degree of anisotropy featuring a strong <001> texture along the BD [Citation162–164]. This texture also results in a strong anisotropy in mechanical properties of the fabricated components [Citation165]. A concept with the aim to reduce the anisotropy of LBAM-fabricated steel parts is the incorporation of an appropriate amount of reinforcing particulates into the steel matrix. Zhai et al. [Citation107] have compared the texture features of 316L steel and 316L-based SMCs reinforced with different TiC contents. Due to the epitaxial growth mode, the grains of the LPBF-fabricated 316L steel grow along the directions of <001> and <101> resulting in an elongated grain morphology. With the addition of 1 wt.% TiC particles, elongated epitaxial grains were still present in the 316L steel matrix. The grain growth of the LPBF-fabricated microstructure reinforced with 1 wt.% TiC also preferentially follows the directions of <001> and <101>. When, however, 3 wt.% TiC particles were added, the grains of the LPBF-ed SMC showed a very different morphology in comparison to the LBAM-fabricated 316L and 316L-based SMCs reinforced with 1 wt.% TiC. The near disappearance of elongated grains in the 316L-based SMCs with 3 wt.% TiC indicates that the epitaxial growth mode was strongly restricted, if not even surpressed. Random crystal orientations of the matrix grains was obtained in the microstructure of these SMCs. The grains were also significantly refined due to the addition of TiC particles, since they act as heterogeneous nucleation sites leading to grain refinement [Citation166].
The reinforcements have a similar effect on the texture of SMCs which mainly consist of martensite. Chen et al. [Citation149] have compared the texture features of LPBF-fabricated WC/1.2767L SMCs with different amount of WC particles. Differing from the typical columnar grains or equiaxed grains observed in 316L-based SMCs, the microstructure of WC/1.2767L SMCs show lath-like grain morphology and their grain size distinctly scales with the weight fraction of WC. The microstructure of the unreinforced 1.2767L steel consists of relatively large martensitic laths with an average size of 2.8 μm (a). When 2 wt.% WC was added, these martensitic laths became more refined (average size = 0.6 μm) (b). The martensite laths became coarser again (average size = 1.1 μm) when 4 wt.% WC was added (c). Further addition of WC up to 6 wt.% causes no remarkable change of the martensite lath size. Instead, the grain morphology changes from lath-shaped to equiaxed grains with refined substructures formed inside the austenite grains (d). As demonstrated by Li et al. [Citation92], the dispersed distribution of the ultrafine carbides along the subgrain boundaries can substantially inhibit the growth of the austenite crystals evoked by the deposition of further layers. This is the reason for the much more refined martensite present in the composite with 2 wt.% WC addition in comparison to the unreinforced 1.2767L steel. With more WC incorporated into the SMCs, more C atoms can segregate at the sub-grain boundaries of the parent austenite, eventually decreasing the self-diffusion activation energy of the Fe-atoms in the austenite [Citation167]. Typically, the growth and transformation kinetics of austenite, which involve recrystallization, are determined to a considerable extent by the diffusion rate of the Fe atoms through the grain or interphase boundaries [Citation168]. The reduced activation energy for self-diffusion of the Fe atoms promotes the formation and migration of vacancies and finally leads to the formation of coarser parent austenitic grains [Citation149]. Thus, coarser martensite laths will form along different habit planes of the austenite during the following rapid solidification process. Owing to the increase of WC content within the steel matrix, the fraction of retained austenite also increases characterized by equiaxed grain morphology. Interestingly, it is easier for the martensitic matrix composites to obtain an isotropic structure compared to the 316L-based SMCs with austenitic matrix, due to the pronounced martensite transformation during the rapid solidification process of LBAM.
Figure 19. Inverse Pole Figure (IPF) maps of 1.2767L tool steel fabricated by LPBF. (a) unreinforced iron-based matrix serving as reference. BD and TD indicate the building and transverse direction, respectively. SMCs reinforced with (b) 2 wt.% WC, (c) 4 wt.% WC and (d) 6 wt.% WC. Reprinted with permission from [Citation149].
![Figure 19. Inverse Pole Figure (IPF) maps of 1.2767L tool steel fabricated by LPBF. (a) unreinforced iron-based matrix serving as reference. BD and TD indicate the building and transverse direction, respectively. SMCs reinforced with (b) 2 wt.% WC, (c) 4 wt.% WC and (d) 6 wt.% WC. Reprinted with permission from [Citation149].](/cms/asset/d6480d20-12d9-451f-9367-583a43a1534e/yimr_a_2258664_f0019_oc.jpg)
Microstructural characteristics of LBAM-fabricated SMCs with different phase composition
Austenitic matrix composites
For the vast majority of published works on LBAM of austenitic steel matrix composites, 316L stainless steel was utilized as the matrix [Citation90,Citation92,Citation94,Citation99,Citation112,Citation158,Citation169–171]. Since 316L is a fully austenitic steel, the matrix of the resulting SMCs also generally shows mainly an austenitic microstructure. There are also works reporting that maraging and 1.2767L tool steels reinforced with high-weight fractions of micron-sized WC possess a fully austenitic microstructure [Citation53,Citation102,Citation172]. These SMCs should have undergone a complete martensitic transformation during LBAM, whereas the austenite stabilizing elements stemming from the reinforcing WC particles inhibit the martensitic transformation, thus causing an austenitic matrix [Citation53].
The microstructure of LPBF-fabricated austenitic stainless steels has been reported to consist of columnar grains together with fine solidification cellular substructures of 1 μm or less in diameter [Citation53,Citation88–90,Citation123]. The solidification cells show very similar crystallographic orientations, so that up to hundreds of them form one austenite grain. The regulation effect of solidification cellular structure facilitates retention of dislocations, leading to a strong trapping effect of dislocations at the cell walls [Citation173]. Thus, the larger-sized columnar grains and solidification cellular substructures as well as dislocation walls collectively create a hierarchical microstructure in the LPBF-fabricated austenitic stainless steels [Citation62]. LBAM-fabricated 316L-based SMCs with an austenitic matrix inherits this hierarchical microstructure. It is also found that the grain morphology of austenite in the LBAM-fabricated SMCs exhibits a large difference compared to the 316L austenitic stainless steels counterpart. One suited example is that, compared to the SMCs, whose microstructure is composed of the 316L matrix and vanadium carbides, the microstructure of unreinforced 316L steel shows distinctly larger grains (see b,e). The extent of the austenite grain colonies is larger than one melt track or rephrased the width of the molten pool (a,b). A significant number of finely cellular substructures could be observed within these grain colonies (c). By contrast, coarse austenitic grains are not found in the microstructure of the LPBF-fabricated SMCs (d,e) which show much more refined cellular substructures with reinforcing nanoscale vanadium carbide particles decorated along the cellular walls (c,f). It was claimed that the generation of ultrafine cellular substructures in the SMCs originated from the (i) ultra-high rapid cooling rates effective during LPBF, (ii) nanoscale vanadium carbides (V8C7, V2C and V4C2.67) serving as nucleation sites for the austenite grains, and (iii) carbides pinning the austenite grains inhibiting their growth [Citation92]. It is interesting to observe that the vanadium carbides are distributed at the subgrain boundaries and within the subgrains (c,f). For the distribution behaviour of reinforced particles within the steel matrix, Fang et al. [Citation60] have proposed five different distribution types of reinforcements and they depend on the critical interface velocity (Rcr) of the particles. During LBAM, the Rcr can be predicted as [Citation93,Citation174]:
(6)
(6)
(7)
(7) where Δσ0 is the repulsive force between reinforced particles and the solid–liquid interface and it depends on the difference in surface tension among them, d is the distance of the interface between reinforced particles and solid phase, n is a constant (2 to 7), μ is liquid viscosity, μ* is the effective viscosity of liquid, r is the particle radius (if there is an agglomeration of reinforced particles, r will be the average radius of agglomerates), λP and λL are the thermal conductivity of reinforcing particles and liquid phase, respectively, and vp is the volume fraction of the particles. By calculating the Rcr effective during LBAM-processing and comparing it with the solidification rate R of molten pool, one can predict the distribution behaviour of reinforcements during the solidification, as shown in . Rafi et al. [Citation175] reported the cooling rate effective during the LPBF process to be on the order of 106 K s−1. The solidification rate can be calculated according to the melting point and molten pool size to be roughly about 2 × 104 μm s−1. According to equations (6) and (7), one can infer that using nano-particles as the reinforcement leads to a large Rcr. Combined with the strong Marangoni flow [Citation176] effective within the molten pool during LPBF, the nano-particles are prone to be pushed to the grain boundaries and agglomerate with each other (type II in ). One typical example is nano-TiC/316L SMCs which AlMangour et al. [Citation88] studied. The reinforcing TiC phase bonded with each other and formed a continuous ring-like structure with a relatively uniform dispersion throughout the steel matrix. By contrast, in the case of LPBF-fabricated V8C7/316L SMCs [Citation92], the V8C7 particles showed a size of approximately 0.8 μm in diameter. In this case, Rcr appears to be smaller than the solidification rate R. Under the influence of Marangoni convection, certain agglomerated vanadium carbides were also entrapped within the grains, whilst others were pushed towards the grain boundaries (type IV in ), as shown in f.
Figure 20. Comparison of the microstructures of 316L steel (top row) and 316L-based SMC (bottom row) with an addition of 3 wt.% vanadium carbide fabricated by LPBF at a laser scan speed of 300 mm s−1, laser power of 140 W, layer thickness of 35 μm and hatch space of 85 μm: (a) Optical micrograph (OM) of 316L, (b) Scanning electron microscopy (SEM) image in SE mode of 316L, (c) high-magnification FE-SEM image of 316L, (d,e) OM images of the SMC, (f) high-magnification FE-SEM image of the SMC. All images were taken from the cross-section which is perpendicular to the building direction (BD) of the LPBF-fabricated parts. Reprinted with permission from [Citation92].
![Figure 20. Comparison of the microstructures of 316L steel (top row) and 316L-based SMC (bottom row) with an addition of 3 wt.% vanadium carbide fabricated by LPBF at a laser scan speed of 300 mm s−1, laser power of 140 W, layer thickness of 35 μm and hatch space of 85 μm: (a) Optical micrograph (OM) of 316L, (b) Scanning electron microscopy (SEM) image in SE mode of 316L, (c) high-magnification FE-SEM image of 316L, (d,e) OM images of the SMC, (f) high-magnification FE-SEM image of the SMC. All images were taken from the cross-section which is perpendicular to the building direction (BD) of the LPBF-fabricated parts. Reprinted with permission from [Citation92].](/cms/asset/6260c5c1-a0e5-47e7-8c62-2a0d787f51b4/yimr_a_2258664_f0020_oc.jpg)
Figure 21. Possible structures in a multidirectional solidification as a function of the solidification rate, convection level, and particles size. Reprinted with permission from [Citation60].
![Figure 21. Possible structures in a multidirectional solidification as a function of the solidification rate, convection level, and particles size. Reprinted with permission from [Citation60].](/cms/asset/d5551d12-356d-4885-919c-d71b148f8223/yimr_a_2258664_f0021_oc.jpg)
The incorporation of reinforcements significantly affects the grain size of the 316L matrix. displays EBSD orientation maps for LPBF-manufactured pure 316L, 5 wt.% TiB2/316L SMC (named SMC-5) and 10 wt.% TiB2/316L SMC (named SMC-10) [Citation112]. With the addition of TiB2, the columnar grains become shorter, narrower and do not extend into adjacent layers anymore (b,c). The average grain size for SMC-5 and SMC-10 is 5.7 and 1.7 μm. These grains are smaller than those in the unreinforced 316L steel. The nano-sized TiB2 particles can serve as sites for heterogeneous nucleation which hinder grain growth, due to a higher density of nucleated grains [Citation177,Citation178]. Salman et al. [Citation95] reported that submicron-sized CeO2 particles can also serve as heterogeneous nucleation sites for 316L matrix and, thus, effectively refine the microstructure of the matrix. These reinforcing phases can exert a strong Zener pinning effect [Citation179] to the steel matrix which hindered the growth of the austenitic grains during the LPBF process of 316L-based SMCs, which promotes the fabrication of finer-grained microstructures.
Figure 22. EBSD analysis showing the grain orientation maps of (a) LPBF-processed pure 316L, (b) 5 wt% TiB2/316L SMC, and (c) 10 wt% TiB2/316L SMC. Reprinted with permission from [Citation112].
![Figure 22. EBSD analysis showing the grain orientation maps of (a) LPBF-processed pure 316L, (b) 5 wt% TiB2/316L SMC, and (c) 10 wt% TiB2/316L SMC. Reprinted with permission from [Citation112].](/cms/asset/e418f574-84d7-4987-bce2-0c4162ff6f8d/yimr_a_2258664_f0022_oc.jpg)
LDED-processed austenitic stainless steel also possesses hierarchical solidification features, however, with more pronounced microsegregations located along the cell walls [Citation62]. Compared to LPBF, a stronger cyclic reheating effect and a lower solidification rate are inherent to LDED [Citation180–182]. The significant cyclic reheating treatment effective during LDED leads to an enrichment of the ferrite stabilizing elements Cr and Mo along the subgrain boundaries [Citation62]. Furthermore, the relatively low solidification rate, which the molten pool experiences during LDED, caused the released atoms from partially or completely melted reinforcements to segregate along the cell walls. The austenite grains then also underwent a columnar to equiaxed transition (CET) [Citation183] which resulted in a greatly refined microstructure. The microstructure of LDED-fabricated 316L steel is characterized by large columnar grains extending across several layers (a). By contrast, equiaxed grains can be obtained in the microstructure of 316L-based SMCs (see the microstructure of TiN/316L SMCs in b–d), which show a typical CET effect. The average grain size decreased gradually from ∼422 μm to ∼9 μm when the TiN content was increased from 0 wt.% to 6 wt.%, respectively. Ouyang et al. [Citation99] also found a similar CET effect in multilayer-graphene reinforced 316L matrix composites via LDED. The graphene causes an apparent refinement effect in the 316L matrix by pinning the grain boundaries. As a consequence, the grains nucleated with the graphene particles as core. Additionally, the graphene offers great heat-conducting property [Citation184] accelerating the solidification of the melt once the laser beam moved away from the molten pool. The resulting higher cooling rate inhibited the growth of columnar grains and promoted further refinement of the grain structure. In this situation, the growth of columnar grains was inhibited and the grain structure was refined. It is worth noting that the equiaxed grains produced by the incorporation of graphene differ in the morphology of grains formed during LDED-fabrication of unreinforced steel parts. The reason behind is the high thermal conductivity of graphene [Citation185].
Figure 23. EBSD orientation maps of unreinforced and reinforced microstructures fabricated by LDED: (a) 316L, (b) 316L-2TiN, (c) 316L-4TiN, and (d) 316L-6TiN. (e) The definition of the colour orientation in maps are given (a–d), and (f) displays the average grain size of the samples shown in (a-d). Reprinted with permission from [Citation171].
![Figure 23. EBSD orientation maps of unreinforced and reinforced microstructures fabricated by LDED: (a) 316L, (b) 316L-2TiN, (c) 316L-4TiN, and (d) 316L-6TiN. (e) The definition of the colour orientation in maps are given (a–d), and (f) displays the average grain size of the samples shown in (a-d). Reprinted with permission from [Citation171].](/cms/asset/77a8681d-0390-4464-89eb-c4e8fef139ff/yimr_a_2258664_f0023_oc.jpg)
Maraging steel- or 1.2767L steel-based SMCs reinforced with high weight fractions (≥15 wt.%) of micron-sized WC also exhibit mainly austenitic microstructures, albeit the fact that, a large proportion of solidified austenite would transform into martensite in these steel materials during LPBF due to the self-quenching effect [Citation186]. Owing to the presence of WC particles, the austenite phase is stabilized during LPBF. The WC transforms into the carbide-type WC1-x after the LPBF process (see ), because of the significant diffusion of carbon from WC particles into the iron-based matrix. Since carbon is a strong austenite forming element, the diffused carbon dissolves in the iron-based matrix as interstitial atoms and inhibits the martensitic transformation of the iron-based matrix during rapid solidification. The diffused carbon atoms also participate in the formation of the carbide-type M6C with the carbide forming elements, such as Fe and W ().
Figure 24. XRD pattern of the LPBF-fabricated SMCs reinforced with 20 wt.% WC. Austenite and martensite as well as carbides of the WC1-x and M6C types were indexed, where the red and blue dashed lines represent the standard 2θ of austenite and martensite, respectively. Reprinted with permission from [Citation53].
![Figure 24. XRD pattern of the LPBF-fabricated SMCs reinforced with 20 wt.% WC. Austenite and martensite as well as carbides of the WC1-x and M6C types were indexed, where the red and blue dashed lines represent the standard 2θ of austenite and martensite, respectively. Reprinted with permission from [Citation53].](/cms/asset/a2cbe63d-7f81-4fd0-92af-35a128fab441/yimr_a_2258664_f0024_oc.jpg)
Another consequence of the addition of high-weight fractions of micron-sized WC is the prevailing formation of a gradient interface (also known as reaction layer) between the WC particles and steel matrix. The interfacial region demarcated by the matrix and reinforcements is of great importance for SMCs, since it can greatly affect their mechanical and physical properties, such as tensile as well as compressive behaviour, thermal conductivity and coefficient of thermal expansion [Citation187]. Based on the compatibility between the matrix and reinforcement, the interface in metal-based composites can be categorized into the following types [Citation188]: (i) reactive interface between ex-situ unmelted reinforcement and matrix, (ii) semi-coherent, and (iii) coherent interface between in-situ formed reinforcement and steel matrix. As the name already suggests, a reactive interface can form, due to a chemical reaction between the matrix and reinforcement during LBAM-processing. This reaction leads to the evolution of compounds at the so-called reactive region which connects the matrix and reinforcement. Chen et al. [Citation53] investigated the interface between the carbide precipitation and iron-based matrix in LPBF-ed SMCs reinforced with micron-sized WC. The WC-particles are depleted in C resulting in the formation of WC1-x particles () and a reactive layer which is well bonded to the matrix (a). This interface is very thin (below 0.5 μm) (b) and the reaction layer is composed of numerous nano-sized particles (f), which were identified as a (Fe, W)3C type carbide. Additionally, the microstructure of SMCs reinforced with WC also consisted of in-situ formed carbides of M6C-type forming a network. Owing to their different lattice parameters, the interface between these M6C and the steel matrix is generally semi-coherent. Besides M6C carbides, spherical and ultrafine precipitates with about 70 nm in diameter can also form in the microstructures of these SMCs. These nanoprecipitates could be identified as the Fe2W2C-type carbide. The nano-sized precipitates are highly coherent with the Fe-based matrix described by the following crystallographic relation: [1 1̅ 1]Fe2W2C//[1 1̅ 0]Fe. The development of such nanoparticles mainly depends on short-range reshuffling of the constituent atoms. This reshuffling is not based on long-range diffusion which is obstructed during the rapid non-equilibrium solidification via LPBF-processing. Kang et al. [Citation102] also found the typical reaction layer in WC-reinforced maraging steel composite fabricated through LPBF. The interfacial region shows a dense microstructure without clear surface separation. Besides, the microstructural features (such as grain size and morphology of the steel matrix) differ in the direct vicinity of the WC-particles and farther away from it. The interface plays an important role in the diffusion behaviour between the WC-particles and the steel matrix. The high thermal conductivity of WC increases the cooling rate effective within the molten pool during solidification and, ultimately, leads to a more refined microstructure.
Figure 25. SEM and TEM images depict the microstructure (a–c) in the direct vicinity of the WC1-x phase and (d) within the iron-based matrix. (e) and (f) show the selected area electron diffraction (SAED) of the regions indicated in (b) and (c), respectively. Reprinted with permission from [Citation53].
![Figure 25. SEM and TEM images depict the microstructure (a–c) in the direct vicinity of the WC1-x phase and (d) within the iron-based matrix. (e) and (f) show the selected area electron diffraction (SAED) of the regions indicated in (b) and (c), respectively. Reprinted with permission from [Citation53].](/cms/asset/879f4d93-03f4-4597-a5cb-6d3e5b350411/yimr_a_2258664_f0025_oc.jpg)
The gradient interface can also be found in LDED-fabricated SMCs reinforced with micron-sized WC particles [Citation169]. The steel matrix showed a dendritic microstructure together with interdendritic eutectic phases (a). The thickness of the interface ranged from 0.9 to 1.8 µm (b), which is larger than that found in LPBF-fabricated WC reinforced SMCs. Li et al. [Citation189] reported that the thickness of such interfaces synthesized by laser processing depends on the cooling rate effective in the molten pool during laser processing. They compared the WC/Ti-interfacial zones prepared by vacuum arc furnace melting and laser melting. Under the condition of slower cooling rates as in vacuum arc furnace melting, the thickness of the interface was nearly 10 µm, which was largely thicker than that produced by laser processing. Both LDED and LPBF utilize the laser as heat source, while the cooling rate of the molten pool during these two processes is largely different [Citation182]. LPBF utilizes a laser with a smaller spot size, which leads to a smaller molten pool entailing faster cooling. Thus, the interface of SMCs produced by LPBF was thinner than those produced by LDED. The thin interface was beneficial in transferring the stress from the matrix to the reinforcing particle leading to an enhanced mechanical performance of SMCs [Citation189].
Figure 26. Microstructure of a WC/Fe-based SMC produced by LDED. The interfacial region is emphasized: (a) a WC-particle embedded in the steel matrix; (b) magnified section of the thin interface. Reprinted with permission from [Citation169].
![Figure 26. Microstructure of a WC/Fe-based SMC produced by LDED. The interfacial region is emphasized: (a) a WC-particle embedded in the steel matrix; (b) magnified section of the thin interface. Reprinted with permission from [Citation169].](/cms/asset/b834b3db-6145-43a9-9f20-408795577691/yimr_a_2258664_f0026_ob.jpg)
Rong et al. [Citation190,Citation191] proposed the formation mechanisms of the reaction interface in LBAM-fabricated WC/Inconel 718 composite parts, which, we believe, can be also applicable to LBAM-fabricated WC-reinforced SMCs. During LBAM-processing, the mixed powder is exposed to the laser beam, which causes the metallic powder blend to completely melt, so that the Fe, Ni, Cr atoms diffuse into the molten pool. Due to the relatively high melting point, the WC particles only underwent surface melting. Thereby, the W and C atoms were partially released into the surrounding melt. The C-concentration is high in the direct vicinity of the WC1-x particles. Extremely high cooling rates are effective during LPBF and the resulting kinetic constraints force the C-atoms to diffuse a short-, instead of long-distance. Thus, the non-uniform C-distribution is preserved and resulted in the formation of a reactive interface-layer. Both the atoms, which released on a short-distance, and the laser energy provided the material and energy conditions for the formation of the gradient interface and diffusion layer (a). Thus, the W and C atoms diffused from the WC1-x particles easily react with the Fe, Ni, Cr atoms, resulting in the formation of the (W,M)C3 (M = Fe, Ni, Cr) carbide which in-situ consists of graded interfacial layer (b). When a higher laser energy input is used as is common in LDED, more C and W atoms are released into the molten pool, leading to the formation of the second category of diffusion layer consisting of (W,M)C2 (M = Ni, Cr, Fe) carbides. Compared to the (W,M)C3 carbide present in the graded interfacial layer, the diffusion layer (W,M)C2 carbides usually form as interdendritic eutectic phases (). These interdendritic phases are slightly enriched in Fe, Ni, Cr and depleted in W and C. Owing to the formation of the (W,M)C2 carbide diffusion layer, chemical diffusion of the W and C atoms from the reinforcing particles into the matrix can be hindered. The diffusion of the Fe, Ni, Cr atoms from the matrix to the surface of the reinforcing particles is also restricted. Therefore, the thickness of the diffusion interface in WC-reinforced composites fabricated by LDED can be controlled at a smaller value compared to other laser processing technique (such as laser melt injection [Citation192]) when a high laser energy source is used.
Figure 27. Schematic illustrating the mechanism for the formation of the gradient interface between the WC particles and Inconel 718 matrix: (a) Released atoms and the laser energy provided material and energy conditions sufficient for the formation of the gradient interface and the diffusion layer; (b) Formation mechanisms of (W,M)C3 and (W,M)C2 carbides within the gradient interface and diffusion layer. Reprinted with permission from [Citation190,Citation191].
![Figure 27. Schematic illustrating the mechanism for the formation of the gradient interface between the WC particles and Inconel 718 matrix: (a) Released atoms and the laser energy provided material and energy conditions sufficient for the formation of the gradient interface and the diffusion layer; (b) Formation mechanisms of (W,M)C3 and (W,M)C2 carbides within the gradient interface and diffusion layer. Reprinted with permission from [Citation190,Citation191].](/cms/asset/4e7ec391-03ed-468d-858a-fc7e81a8d267/yimr_a_2258664_f0027_oc.jpg)
Martensitic matrix composites
For the LBAM-fabrication of SMCs with mainly martensitic matrix, AISI 420 stainless steel [Citation93], H13 [Citation89] and 1.2767L tool steel [Citation17], as well as 18Ni-300 maraging steel [Citation193], have been used as the steel matrix. During the laser-induced rapid cooling process, austenite firstly crystallized from the melt and subsequently transformed into martensite, due to the self-quenching effect [Citation186] during LBAM. Chen et al. [Citation17] investigated the microstructural evolution of submicron-sized WC reinforced 1.2767L-based SMCs produced via LPBF (see ). Besides ring-like cellular substructures, the SMCs also present typical lath-like martensitic structures. This microstructural feature can also be found in LPBF-processed TiC/AISI420 [Citation114], TiN/AISI420 [Citation93], TiC/H13 [Citation89], and TiB2/H13 [Citation91] SMCs. Chen et al. [Citation17] also proposed the mechanism for the SMCs with martensitic matrix during the solidification process of LPBF. With the instantaneous laser exposing on the WC/1.2767L composite powder, the high-energy laser beam melts the composite powder, so that the WC particles will be most likely fully dissolved and diffuse into the iron-based matrix. The W- and C-atoms should be then distributed within the molten pool in a uniform manner (b). As the laser scanning line advances, the molten Fe-W-C alloy rapidly solidifies. Then the Fe2W4C type carbide should precipitate along the cell boundaries during the subsequent solidification (c). Due to the ultrahigh cooling rates effective during LPBF, firstly the austenitic steel-matrix precipitates and subsequently transforms into martensite (d), when the temperature is lowered below the martensite start temperature, Ms. Once the martensite finish temperature, Mf, is reached, the martensitic transformation is completed.
Figure 28. Schematic illustration depicting the mechanism of the solidification process during LPBF of the composite powder. (a) The composite powder is exposed to the laser beam (red circle). (b) The laser beam fully melts the Fe-based matrix and WC particles which are uniformly dissolved within the resulting liquid. (c) Fe2W4C carbides precipitate into a network. The residual melt solidifies into the austenitic Fe-based matrix (d) The austenite matrix transforms into martensite, when cooled below the martensite start temperature, Ms. Transformation continues until the martensite finish temperature, Mf, is reached. Secondary electron micrographs show the microstructure of etched submicron-sized WC/1.2767L SMCs samples fabricated by LPBF at different laser energy densities of (e) 60 J mm−3 and (f) 150 J mm−3. Reproduced with permission from [Citation17].
![Figure 28. Schematic illustration depicting the mechanism of the solidification process during LPBF of the composite powder. (a) The composite powder is exposed to the laser beam (red circle). (b) The laser beam fully melts the Fe-based matrix and WC particles which are uniformly dissolved within the resulting liquid. (c) Fe2W4C carbides precipitate into a network. The residual melt solidifies into the austenitic Fe-based matrix (d) The austenite matrix transforms into martensite, when cooled below the martensite start temperature, Ms. Transformation continues until the martensite finish temperature, Mf, is reached. Secondary electron micrographs show the microstructure of etched submicron-sized WC/1.2767L SMCs samples fabricated by LPBF at different laser energy densities of (e) 60 J mm−3 and (f) 150 J mm−3. Reproduced with permission from [Citation17].](/cms/asset/7a211f48-db42-4a22-a1d3-048780f0cb5c/yimr_a_2258664_f0028_oc.jpg)
The incorporation of reinforcements can also promote precipitation of nanoparticles in the martensitic matrix. Tan et al. [Citation156] have compared the precipitation behaviour of 18Ni-300 maraging steel (named as MS) with its composites reinforced with different amount of SiC. The solidified microstructure of the MS samples is free of precipitates (a). By contrast, a large number of precipitate particles was observed in the as-built 3 vol.% micron-sized SiC/MS SMCs (named as M3) without post heat-treatment (b,c). The incorporation of SiC promoted in-situ precipitation during laser processing and additionally larger SiC particles of about 50–100 nm in diameter were observed (marked with yellow arrows in c). The SAED pattern from the selected SiC particle (d) consists of two sets of diffraction patterns (i.e. martensite and α-SiC). The corresponding high-resolution TEM (HRTEM) analysis of both phases further confirms the presence of the α-SiC and martensite phases (e–g). Based on the results from the TEM analysis, we note that in contrast to the as-fabricated MS, a large number of precipitates formed in the as-fabricated SMCs. Consequently, the addition of SiC affects the aging behaviour of MS. According to literature, the precipitation behaviour in maraging steel is strongly related to the activation energy for the growth of precipitates [Citation194]. In order to calculate this activation energy, E, the so-called Augis-Bennett modified Kissinger equation was deduced as follows [Citation195]:
(8)
(8) where T0 is the initial temperature, TP is the exothermic peak temperature of the precipitates, α is the heating rate, R is the gas constant, and k is the reaction constant, whereby T0 = 303 K and C = 8.31 J mol−1 K−1. One can plot ln[(Tp-T0)/α] vs. 1/TP. A subsequent linear fit allows to determine the slope of k which follows from E = kC. Tan et al. [Citation156] have determined the activation energy values of MS-based SMCs with 6 and 12 vol.% SiC as 58.2 and 62.0 kJ mol−1, respectively. Increasing additions of SiC also increases the activation energy and, hence, quantitatively proves the influence of SiC-additions on the aging behaviour of MS via influencing the growth activation energy of precipitates.
Figure 29. TEM microstructure analyses of the LPBF-processed MS and M3 samples. (a) BF-TEM of MS, (b) and (c) BF-TEM of M3 at different magnifications, (d) SAED of the encircled region in (c), a close-view of the SiC-matrix interface taken from region e in (c), and FFTs of (f) SiC and (g) matrix corresponding to zones f and g indicated in (e). Reprinted with permission from [Citation156].
![Figure 29. TEM microstructure analyses of the LPBF-processed MS and M3 samples. (a) BF-TEM of MS, (b) and (c) BF-TEM of M3 at different magnifications, (d) SAED of the encircled region in (c), a close-view of the SiC-matrix interface taken from region e in (c), and FFTs of (f) SiC and (g) matrix corresponding to zones f and g indicated in (e). Reprinted with permission from [Citation156].](/cms/asset/f1d5fd51-d990-4320-8693-d8e4eb03135e/yimr_a_2258664_f0029_oc.jpg)
The variation of the activation energy in the SMCs reinforced with different contents of SiC can be explained as follows. (i) laser processing refined the SiC particles down to the nanoscale, because of the partial melting of the outer regions of the SiC particles. This phenomenon was also reported in SiC-reinforced pure iron [Citation196] and SiC/316L SMCs [Citation113] fabricated by LPBF. The nanosized SiC particles provided a high number of favourable heterogeneous nucleation sites for the precipitates promoting their formation via heterogeneous nucleation. (ii) The physical property mismatch between the SiC particles and the MS matrix could generate a large number of dislocations stemming from the high strain imparted to the matrix. Dislocations could also act as preferential nucleation sites for the precipitates, as they can reduce the free energy required for nucleation. This leads to an increased nucleation rate of the precipitates. (iii) The formation of martensitic laths is generally accompanied by a high dislocation density evolving within them [Citation197]. The high density of dislocations in martensite provides channels for the diffusion of solute atoms which then accelerate the diffusion rate [Citation198]. Therefore, the precipitation behaviour is intensified and the aging strengthening process leads to the formation of a high number of precipitates in the SMCs without post heat-treatment. We note that such precipitates were only observed in unreinforced MS after heat-treatment [Citation199]. (iv) Higher additions of SiC reinforcement could lead to even higher nucleation rates effective during solidification of the steel matrix. The precipitates then compete for growth when nucleated in the same matrix and solute concentration. Thus, the higher nucleation rate of the precipitates limits the growth of subsequent precipitates which requires more energy.
Even though the cooling rate of the molten pool effective during LDED is reported to be lower than during LPBF [Citation62], it is still sufficiently high to induce the martensitic transformation during the rapid solidification process, as shown by Zhu et al. [Citation200]. Hu et al. [Citation193] have demonstrated that incorporation of TiC also reduces the size of martensite in the 18Ni-300 steel matrix fabricated by LDED (a,c). Majority of the micron-sized TiC particles with an average size of ∼1.25 μm along with a small number of nano-sized Ti2N and TiC particles with a size of ∼90 nm were distributed within the martensite matrix. Moreover, the TiC/18Ni-300 SMCs showed higher dislocation density than the unreinforced 18Ni-300 counterparts (a,c). This observation can be explained by the mismatch in CTE between TiC and the 18Ni300 steel matrix, which is similar to the increased dislocation density after the introduction of TiC into the SKD 11 tool steel [Citation201] and 316L stainless steel [Citation123]. After subsequent heat treatment (solution treatment at 850°C for 1 h and a subsequent aging treatment at 500°C for 3 h), the microstructure of the TiC/18Ni-300 SMC additionally showed nano-sized Ni3(Ti,Mo) particles (5–10 nm) and a few patches of austenite which were dispersed in the martensite matrix.
Figure 30. TEM analysis showing the bright field (BF) image, SAED pattern and dark field (DF) image of 18Ni-300 steel samples (a, b), 5 vol.% TiC/18Ni-300 SMCs (c, d) and heat-treated SMCs (e, f). (g) Schematic diagram of the formation mechanism of TiC particles with different morphologies: granular-shape; strip-shape; and flower-shape. Reprinted with permission from [Citation193].
![Figure 30. TEM analysis showing the bright field (BF) image, SAED pattern and dark field (DF) image of 18Ni-300 steel samples (a, b), 5 vol.% TiC/18Ni-300 SMCs (c, d) and heat-treated SMCs (e, f). (g) Schematic diagram of the formation mechanism of TiC particles with different morphologies: granular-shape; strip-shape; and flower-shape. Reprinted with permission from [Citation193].](/cms/asset/99c5899d-db13-41e9-b93b-a3fc9e3c6d18/yimr_a_2258664_f0030_oc.jpg)
Furthermore, the TiC particles were shown to form with various morphologies including granular-shape, strip-shape and flower-shape when the SMCs were fabricated at different processing conditions. g visualizes the formation mechanism of these TiC particles with varying morphologies during LDED-fabrication of the TiC/18Ni300 SMCs. When the powder mixture is melted by the laser beam, the TiC particles partially melt/decompose and the elements diffuse into the metal matrix. Thereby, they are encapsulated by the molten liquid [Citation202]. Since the density of 18Ni-300 steel (7.85 g/cm3) is higher than that of the TiC (4.25 g/cm3) [Citation193], the TiC particles will float upward within the molten pool, because of the Marangoni convection effect. The particles then collide and sinter during floating which results in different growth morphologies of the TiC particles [Citation203]. A possible formation mechanism for the TiC particles with different morphologies is suggested as follows:
Granular-shaped TiC: The granular-shaped TiC particles can be classified into two types, such as the micron-sized unmelted/partial-melted TiC and nano-sized precipitated TiC. The latter was formed by the in-situ reaction between supersaturated Ti and C atoms from the liquid matrix alloy as the temperature of the molten pool drastically decreased due to the advanced laser beam [Citation204].
Strip-shaped TiC: The growth mechanism of the strip-shaped TiC particles can be attributed to the collision of several un-melted or partial-melted TiC particles and the subsequent sintering of these TiC particles under the influence of the Marangoni convection effective in the molten pool [Citation205].
Flower-shaped TiC: The flower-shape morphology of TiC can be subclassified into two types: (a) TiC-shape with bonded petals are generated via collision and sintering of unmelted/ partial-melted TiC particles at close distances; and (b) TiC-morphology with separated petals follows from movement of some unmelted/partial-melted TiC particles at far distances towards each other during fast cooling.
Duplex austenitic/martensitic matrix composites
Duplex austenitic/martensitic matrices can be mainly found in LBAM-fabricated TiC or SiC reinforced SMCs. The duplex microstructure usually results from the largely differing CTE between the reinforcement and steel matrix, and reduced Ms-temperature caused by the increased fraction of austenite stabilizers stemming from reinforcements. The matrix of both LPBF- and LDED-fabricated TiC or SiC reinforced SMCs, partially undergo the phase transformation from austenite to martensite during processing [Citation206]. With augmenting TiC or SiC content in the SMCs, more austenite can be retained in the steel matrix, thus a duplex austenitic/martensitic matrix can form in the solidified microstructure.
Chen et al. [Citation207] have investigated the effect of TiC content on the microstructure evolution of the TiC/15-5PH SMCs prepared via LDED. In the TiC-free steel specimen, martensite lath structures with a high-density of dislocations and an average size of 16 μm dominated the microstructure. It was accompanied by retained austenite (RA, 2.6 vol.%) which was located as a film along the lath boundaries (). The RA and martensite showed the Kurdjumov-Sachs (K-S) orientation relationship . During LDED-processing of martensitic steel, a fully martensitic microstructure can be achieved owing to the fast cooling rates effective during LDED (102–104 K s−1 [Citation208]). The addition of TiC particles induced an incomplete martensitic transformation by decreasing the Ms-temperature [Citation107] and thus resulted in the formation a martensite/austenite duplex microstructure. The addition of TiC particles substantially refined the block size of martensite and induced a great change in the microstructure characterized by a larger fraction of RA. Compared to the TiC-free steel specimen, the volume fraction of RA in the 1 wt.% TiC/15-5PH SMCs showed a significant increase (20.7%), and the presence of block-type RA within the martensite matrix could be observed, which was indexed with a Nishiyama-Wassermann (N-W) orientation relationship of
. Further increase in the content of TiC to 2 wt.% resulted in an austenite-dominated microstructure (d) with a volume fraction of 61.2 vol.% RA, as inferred from EBSD analysis. The RA exhibits a blocky grain morphology with a dimension of ~18.7 μm. Contrary to expectation, a slightly decreased proportion of RA (41.5 vol.% by EBSD) is observed in the SMC specimen with 4 wt.% TiC, which can be ascribed to the decreased TiC particles dissolution. Further additions of TiC up to 8 wt.% substantially decreased the volume fraction of RA (32.5 vol.% by EBSD) due to the more sluggish dissolution of the reinforcing TiC particles. The RA also showed a ring-like structure (f–g) which can be ascribed to the micro-segregation of C and Ti elements resulting from the evolution of (Nb,Ti)C particles within the ring-structure regions. The partial non-dissolution of TiC particles can account for the formation of submicron-sized (Nb,Ti)C particles. The dissolution of TiC particles depends on both the holding temperature and duration of laser irradiation. However, the substantially increased energy absorption caused by the mass addition of high-melting TiC particles leads to overall insufficient laser energy input, and eventually slows down the dissolution of TiC. According to Refs. [Citation209–211], undissolved TiC particles could act as nucleation sites for (Nb,Ti)C carbide when subjected to (1) the high heat flux during laser irradiation and (2) the following intrinsic heat treatment induced by the deposition of subsequent layers. These undissolved TiC particles can be continuously driven by the torque force and gather around the centre of the Marangoni flow pattern [Citation212] resulting in the formation of continuous ring-like structures, as illustrated in . The TiC/316L [Citation88], SiC/316L [Citation113], SiC/18Ni300 [Citation156] SMCs fabricated by LPBF also exhibit similar ring-like structure. Interestingly, it was found that in the LDED-fabricated SiC/316L SMCs [Citation213], besides the ring-like arranged SiC, ultrafine iron silicide phases, such as Fe3Si and FeSi, could also be detected. Their formation indicates that Si, which was released from SiC, diffused into the Fe-based melt and, subsequently, reacted with Fe during LDED-processing. The formation of iron silicides has also been reported by Majumdar et al. [Citation214] and Lo et al. [Citation215].
Figure 31. Inverse pole figures (IPFs), and phase maps of the as-built TiC/15-5PH SMCs reinforced with different TiC contents: (a) 0 wt.%, (b) 0.5 wt.%, (c) 1 wt.%, (d) 2 wt.%, (e) 4 wt.%, and (f) 8 wt.%; (g). Phase map of the SMC specimen with 8 wt.% TiC and the corresponding EDS mapping of Nb, Ti and C elements at higher magnification than in (f). Reprinted with permission from [Citation207].
![Figure 31. Inverse pole figures (IPFs), and phase maps of the as-built TiC/15-5PH SMCs reinforced with different TiC contents: (a) 0 wt.%, (b) 0.5 wt.%, (c) 1 wt.%, (d) 2 wt.%, (e) 4 wt.%, and (f) 8 wt.%; (g). Phase map of the SMC specimen with 8 wt.% TiC and the corresponding EDS mapping of Nb, Ti and C elements at higher magnification than in (f). Reprinted with permission from [Citation207].](/cms/asset/afe55d3f-c2f3-4522-a540-25ddecf9d7b0/yimr_a_2258664_f0031_oc.jpg)
Figure 32. Schematics illustrate the evolution of (Nb,Ti)C particles with the increase of TiC content. Reprinted with permission from [Citation207].
![Figure 32. Schematics illustrate the evolution of (Nb,Ti)C particles with the increase of TiC content. Reprinted with permission from [Citation207].](/cms/asset/541264e7-ba0e-4971-9672-56abf7c8fc3f/yimr_a_2258664_f0032_oc.jpg)
Ferritic matrix composites
SMCs with a ferritic matrix are generally fabricated using pure iron or high chromium steels as the matrix constituent. Nano-sized carbides, oxides, and carbon nanotubes are often selected as the reinforcing constituent to tailor the mechanical properties of the ferritic matrix. In order to ensure a good dispersion of the nanoparticles on the surface of the steel powder, preparation methods like agent deposition and laser-aided dielectrophoretic deposition are generally used in preparing the composite powder. These methods result in a more homogeneous microstructure with uniformly dispersed nano-sized reinforcements within the SMCs and, hence, with optimized mechanical performance.
Doñate-Buendia et al. [Citation134] prepared nano-sized Y2O3 oxide dispersion strengthened (ODS) iron–chromium ferritic steel (PM2000) via LPBF and LDED. They compared the microstructural features and mechanical properties of the SMCs fabricated by different LBAM methods. A direct comparison of the resulting microstructures () demonstrates a pronounced effect of the added nanoparticles on the grain orientation. In the case of LDED, the ODS SMC (c,d) displays an increased fraction of subgrain structures than the LDED-ed steel without reinforcement (a,b). This microstructural effect is less pronounced for the LPBF sample (e, f, g and h). The fabrication technique as well as the addition of nanoparticles determine the crystallographic texture of the resulting microstructure. The most distinct texture for LBAM-fabricated ODS SMCs is with (001) parallel to the building direction, which is typical for microstructures fabricated by LBAM-techniques, particularly when polycrystalline substrates are used, due to directional solidification [Citation216]. On the other hand, the LDED samples show other orientations also (a–d) and, hence, have lower texture intensities. In contrast, both the LPBF-fabricated unreinforced steel and ODS SMCs show a sharper fibre texture (f,h). Doñate-Buendia et al. [Citation134] then used atom probe tomography (APT) to conduct a more detailed investigation of the nanoscopic features of the ODS SMCs samples (see ). For the present SMC synthesized by LPBF, the presence of Y-O nanoparticles with a size similar to the initially added Y2O3 nanoparticles can be proven via APT. By contrast, no Y-O nanoparticles are observed in the LDED sample. The nanoparticles within the LPBF-ed microstructure consist of equal fractions of Y- and O as well as approximately 10–15 at% Ti (d). Thus, the nanoparticle will be referred to as Y-Ti-O phase, that contributes to the enhanced mechanical response of the LPBF sample when compared to the LDED sample, in which no Y-Ti-O nanoparticles were detected [Citation217]. LPBF-fabricated samples also exhibit Ti-C structures surrounding these Y-enriched nanoparticles which play a role in pinning low-angle grain boundaries (c). The presence of Ti-C-based nanostructure with its large fraction of grain boundaries has been proven to contribute to the strengthening of the ODS-steel due to grain refinement [Citation95].
Figure 33. Inverse pole figure (IPF) maps of steel-based samples produced by two LBAM-techniques (LDED and LPBF) along the cross-section (viewing direction perpendicular to the building direction) for: (a) LDED pure steel, (c) LDED ODS steel, (e) LPBF raw steel, and (g) LPBF ODS steel; and along the longitudinal section (viewing direction parallel to the building direction) for: (b) LDED pure steel, (d) LDED ODS steel, (f) LPBF pure steel, and (h) LPBF ODS steel. The colour-coding refers to the building direction of the sample (see inset). Reprinted with permission from [Citation134].
![Figure 33. Inverse pole figure (IPF) maps of steel-based samples produced by two LBAM-techniques (LDED and LPBF) along the cross-section (viewing direction perpendicular to the building direction) for: (a) LDED pure steel, (c) LDED ODS steel, (e) LPBF raw steel, and (g) LPBF ODS steel; and along the longitudinal section (viewing direction parallel to the building direction) for: (b) LDED pure steel, (d) LDED ODS steel, (f) LPBF pure steel, and (h) LPBF ODS steel. The colour-coding refers to the building direction of the sample (see inset). Reprinted with permission from [Citation134].](/cms/asset/13b7862a-f32f-4afb-a0af-de1e530aa154/yimr_a_2258664_f0033_oc.jpg)
Figure 34. Atom probe tomography (APT)-results from ODS steel matrix composites fabricated by (a-b) LDED and (c-d) LPBF. Reprinted with permission from [Citation134].
![Figure 34. Atom probe tomography (APT)-results from ODS steel matrix composites fabricated by (a-b) LDED and (c-d) LPBF. Reprinted with permission from [Citation134].](/cms/asset/19571d47-5d31-4dc8-aa99-08998793e995/yimr_a_2258664_f0034_oc.jpg)
The presence of Y-enriched nanoparticles is also confirmed by Boegelein et al. [Citation218] who observed multi-phase precipitates containing various elements, i.e. O, Ti, Al, Fe, Cr, and Y, in an ODS steel fabricated via LPBF. They carried out an APT-analysis similar to the one presented in and also demonstrated the complex phase formation during LBAM-processing. Further investigations will be focused on completely elucidating the phase evolution during LBAM-processing of steel powders blended with nano-sized oxide additions.
LBAM fabricating SMCs consisting of ferritic microstructure decorated with nano-sized carbides, CNTs and graphene oxide also attracted numerous attentions in recent years [Citation85,Citation96,Citation98,Citation100]. It has been reported that the addition of, for instance, nano-sized SiC particles, is effective in fabricating more isotropic and refined microstructure of SMCs (LPBF-fabricated SiC/Fe SMCs). Additionally, transformation products such as pearlite, which consists of α-Fe and Fe3C, were also generated. The evolution of pearlite is associated with C released from the nano-sized SiC. Nano-sized SiC retained in the steel matrix could also serve as heterogeneous nucleants during the solidification process and promote grain refinement [Citation219]. In another work, nano-sized Cr3C2 was incorporated into a steel-matrix leading to the evolution of refined columnar dendrites and equiaxed grains coexisting in the matrix [Citation96]. Furthermore, this work demonstrated that one can control the columnar to equiaxed transition process of the SMC-microstructure to some extent by modulating the LBAM processing conditions. One can find several works in literature about preparation of carbon nanotubes or graphene oxide reinforced ferrite-based composites via powder bed laser sintering [Citation98,Citation100]. The feedstock is prepared via the agent deposition method by using PVA as dispersing agent, so that the CNTs or graphene oxide can be deposited on the surface of the steel matrix powder in a uniform fashion, as we introduced in 'Preparation methods of SMC feedstock powder' section. In powder bed laser sintering generally a low laser power is used when compared to LPBF, so that majority of the CNTs or graphene oxide can be retained without losing their nanostructure characteristic. The CNTs have much larger thermal conductivity than the iron-based matrix. Thus, the operative temperature during laser sintering process is reduced for composite powder blends with increasing volume fractions of CNTs [Citation98], leading to a lower thermal stress in the laser-processed layer. Because of that, preservation of the CNTs within the matrix is promoted during laser sintering. These CNTs can be separated and uniformly distributed within the iron-based matrix (). The graphene oxide is easier to be preserved during laser sinter processing compared to CNTs, due to its high melting temperature and thermal stability [Citation100]. σ-bonding is the bonding-type between the in-plane carbon atoms of the graphene resulting in high mechanical strength [Citation220]. Since the basal plane of graphene is stable, formation of carbides is not favoured at the side contact. Therefore, carbides form at the interface through the end contact interaction with the matrix (σ-bond formation) [Citation221]. The carbide formation decreases the contact angle of the liquid and graphene oxide, which will improve their wetting behaviour [Citation220]. Thus, a strongly bonded interface can be obtained between the carbides within the Fe-based matrix and the graphene oxide.
Figure 35. Morphology of multiwalled carbon nanotube (MWNT)/Fe nanocomposites prepared by laser sintering. (a) and (b) Morphology of MWNTs at the surface; (c) MWNTs distributed in the cross section of the iron matrix. Reprinted with permission from [Citation98].
![Figure 35. Morphology of multiwalled carbon nanotube (MWNT)/Fe nanocomposites prepared by laser sintering. (a) and (b) Morphology of MWNTs at the surface; (c) MWNTs distributed in the cross section of the iron matrix. Reprinted with permission from [Citation98].](/cms/asset/1daad7e1-6277-4f06-bd58-244e68cb2f95/yimr_a_2258664_f0035_ob.jpg)
Mechanical properties and strengthening mechanisms
In this section, the mechanical properties of SMCs with different kinds of matrix are summarized and the interrelationship between microstructure and mechanical properties is demonstrated. The underlying strengthening mechanisms in LBAM-fabricated SMCs are also discussed.
Austenitic matrix composites
Recent works have focused on the tensile and compressive properties as well as hardness of LBAM-fabricated SMCs composed of mainly austenitic matrix. presents an overview of the mechanical properties of the austenitic matrix composites fabricated via LBAM. One can observe that the tensile strength of LBAM-processed SMCs can be effectively increased by incorporating appropriate amounts of reinforcements, whereas the ductility of these SMCs decreases. Thus, the improvement in strength of SMCs was usually accomplished by scarifying ductility. As one typical example LPBF-fabricated V8C7/316L SMCs can be given. They show much higher strength than the 316L steel counterpart, while their fracture elongation is reduced to 11.3–18.5% [Citation92]. Similarly, 9 vol.% SiC-reinforced SMCs fabricated by LPBF demonstrated an excellent tensile strength of ∼1301 MPa at the expense of reduced elongation to ∼5.1%. However, the work of Zhai et al. [Citation107] depicts an exception to this trend. They have incorporated micron-sized TiC particles into 316L steel. The strength of the resulting TiC/316L SMCs increased while high plastic deformability with a large elongation of ∼55% was achieved.
Table 3. Overview of published mechanical properties of mainly austenitic matrix composites produced via LBAM.
The LDED-produced SMCs with austenitic matrix generally show lower strength and stronger anisotropy in comparison to the LPBF-fabricated SMCs. The reason behind this difference is the formation of coarser, epitaxial-grown columnar grains characteristic for microstructures synthesized by LDED. As one representative example the LDED-fabricated TiN/316L SMC with an addition of 2 wt.% TiN can be considered. This SMC showed an almost isotropic mechanical performance with good combination of tensile strength of ∼640 MPa and ductility of ∼30%, which can be attributed to the prevailing formation of equiaxed grains [Citation171]. It is worth noting that another promising reinforcement for the preparation of high-performance SMCs via LDED is graphene [Citation99]. The fabricated 316L-based SMC reinforced with 2 wt.% graphene showed an ultrahigh microhardness of 765 HV, due to the significantly refined equiaxed grains and strong pinning effect of graphene. However, the investigation of strength and toughness of these LDED-fabricated SMCs reinforced with graphene is still lacking and further investigation are needed.
summarizes the present results demonstrating the superior mechanical properties of LBAM-produced SMCs (mainly LPBF) with austenitic matrix, 316L counterparts fabricated via different processing methods, and further MMCs. One can observe that the strength and particularly the combination of strength and ductility of LBAM-fabricated austenitic matrix composites is better than TiC or SiC reinforced Al–Si alloys and pure 316L counterparts fabricated by other processing methods, such as spark plasma sintering [Citation50].
Figure 36. Mechanical properties of LBAM-produced (mainly LPBF) SMCs with austenitic matrix, 316L counterparts fabricated via different processing method, and other MMCs. Reproduced with permission from [Citation113].
![Figure 36. Mechanical properties of LBAM-produced (mainly LPBF) SMCs with austenitic matrix, 316L counterparts fabricated via different processing method, and other MMCs. Reproduced with permission from [Citation113].](/cms/asset/db289a92-5ad5-4e36-97e8-aa646ef7d02f/yimr_a_2258664_f0036_oc.jpg)
The significantly increased strength of LBAM-fabricated austenite matrix composites can be explained by grain refinement according to the Hall-Petch effect, dislocation strengthening caused by the different CTEs between matrix and reinforcements, grain boundary strengthening (load transform strength) and Orowan strengthening via forming uniformly distributed reinforced particles in the steel matrix [Citation51,Citation52]. AlMangour et al. [Citation112] also reported a segregation strengthening effect [Citation224] in TiB2/316L SMCs fabricated via LPBF. Below are detailed descriptions about the strengthening mechanisms in LPBF-fabricated austenitic matrix composites, using TiB2/316L SMCs as an example.
The total yield strength, σs, of TiB2/316L SMCs can be predicted from the root square of the above-mentioned effects:
(9)
(9) where σA is the strength of the 316L alloy matrix (290 MPa, [Citation112]), σse is the contribution of segregation strengthening, which can be expressed as [Citation225]:
(10)
(10) where dcell is the length of the cellular structure boundary to which the elements are segregated, δ is a material constant and emulates the effects of the segregated elements such as Cr and Mo (742 MPa d −1/2 [Citation112], d is the grain size of the austenitic matrix), vp is the volume faction of the reinforcements.
σor is the contribution of Orowan strengthening via dispersed nanoparticles in the matrix and can be expressed as [Citation84]:
(11)
(11)
(12)
(12) where M is the Taylor factor for 316L (3.06), v is the Poisson’s ratio (0.25), G is the shear modulus (78 GPa), L is the interparticle spacing, b is the Burgers vector (0.254 nm), and dp is the average reinforcement diameter.
σHP is the contribution of grain-boundary strengthening, which enhances the overall strength can be described by the Hall-Petch relation [Citation226]:
(13)
(13) where σ0 is the friction stress in the absence of grain boundaries, ky represents the strength coefficient.
σdis contributes to the increased strength, due to the increased density of geometrically necessary dislocations (GNDs). GNDs increase to accommodate the mismatch between the CTEs and the elastic moduli of the matrix and reinforcement particles. The increase in strength, due to GND density can be calculated as [Citation227]:
(14)
(14) where β is a geometric constant and ρGND is the GND density.
The last common strengthening mechanism in LPBF-fabricated SMCs with austenite matrix is the load transfer effect. The strong interfacial bonding between the 316L matrix and reinforcements promotes the transfer of the load applied to the material from the matrix to the reinforcement. Ye et al. [Citation228] proposed a model describing the relationship between the elastic strain ϵφ and uniaxial loading stress σLT defined by the effective elastic response of Fe-TiB2 SMCs as follows:
(15)
(15) where ω is the angle related to the diffraction crystal planes (hkl),
,
are the effective Poisson’s ratio and effective elastic modulus of the complete composites. The effective elastic constants (EECs) of the composites were described according to the Mori-Tanaka scheme [Citation229]:
(16)
(16)
(17)
(17) where
,
, vp is the volume faction of the TiB2 reinforcement, Km and Gm are the effective bulk modulus and effective shear modulus of the constituents (m = 0 for matrix, m = 1 for reinforcements), respectively. One can find that, with increasing elastic strain ϵφ, the load transfer effect becomes more pronounced. As ϵφ reaches the yield point, for the equiaxed reinforcements, the uniaxial loading stress σLT can be simplified as follows [Citation84]:
(18)
(18) where σm is the strength of the steel matrix (σm = 290 MPa for 316L alloy [Citation112]).
According to above proposed strengthening model, AlMangour et al. [Citation112] estimated the room-temperature yield strength of the 316L matrix composites reinforced with 5 and 10 vol.% TiB2 to be 826 and 984 MPa, respectively. The contributions of each strengthening mechanism to the room-temperature yield strength are displayed in . The predicated yield strength shows a good agreement with the experimentally determined strengths of 827 and 980 MPa for SMCs with 5 and 10 vol.% TiB2, respectively. This result validates the above-mentioned strengthening mechanisms to be effective during loading of LPBF-fabricated SMCs with austenitic matrix.
Figure 37. Comparison of the contributions of each strengthening mechanism to the room-temperature strengths of SMC reinforced with 5 and 10 vol.% TiB2. Reprinted with permission from [Citation112].
![Figure 37. Comparison of the contributions of each strengthening mechanism to the room-temperature strengths of SMC reinforced with 5 and 10 vol.% TiB2. Reprinted with permission from [Citation112].](/cms/asset/96293dfb-eb08-4b5d-b3cc-df1799f04913/yimr_a_2258664_f0037_oc.jpg)
With the working environment of metallic parts becoming harsher, it is necessary for them to show not only required mechanical properties, but also an excellent corrosion resistance to the harsh environment. With the incorporation of reinforcements, the corrosion resistance of 316L-based SMCs prepared by LBAM can be further improved, due to the formation of certain phases and passive films. As one example, the corrosion behaviours of the LPBF-fabricated 316L stainless steel and the 316L-based SMCs strengthened with the amorphous Fe43.7Co7.3Cr14.7Mo12.6C15.5B4.3Y1.9 alloy were compared by Zheng et al. [Citation230]. It was found that the SMCs showed a higher pitting potential value, a wider passive region, a longer pitting incubation time, and the faster formation of the passive film compared to the 316L stainless steel. The better corrosion behaviour was attributed to the formation of yttrium oxides, the solid solution of additional elements, and smaller grains in composites. The grain refinement effect also plays an important role in the corrosion resistance of SMCs. For example, the LPBF-fabricated W/316L SMCs exhibited a better corrosion resistance compared to the 316L stainless steel, because the grain refinement could effectively increase the activity of electrons at grain boundaries leading to the fast production of stable passive films [Citation60]. Thus, the formation of the passive film is an important mechanism for the enhancement of the corrosion resistance of SMCs, and the addition of reinforcements can promote the formation of these passive films. Another example is the addition of Co-Cr-Mo-W alloy powder into the 316L stainless steel which could increase the thickness and Cr2O3 content of the passive film, so that a Co-Cr-Mo-W/316L SMCs with good corrosion resistance could be acquired [Citation231]. Additionally, the content of reinforcements can also have a strong influence on the corrosion resistance of 316L-based SMCs. Zhao et al. [Citation232] reported that an increased TiC content caused the inhomogeneous distribution of TiC particles in the steel matrix which led to the formation of pores inside the LPBF-fabricated SMCs. The existence of pores distinctly attenuated the corrosion resistance of the SMCs, because pores serve as initiation sites for pitting corrosion [Citation233]. In summary, the grain refinement effect, the formation of certain phases as well as passive films, and the content of reinforcements synergistically affect the corrosion resistance of LBAM-fabricated 316L-based SMCs. Thus, the combination of the steel matrix and reinforcements, the reinforcement content and LBAM processing parameters should be appropriately selected to achieve an excellent corrosion resistance for 316L-based SMCs.
Martensitic matrix composites
Owing to the strengthening effect stemming from the high-strength martensite and high-hardness reinforcements, the LBAM-fabricated SMCs with mainly martensitic matrix generally show ultrahigh strength, hardness, and wear resistance. gives a summary of the mechanical properties of mainly martensitic steel matrix composites produced by LBAM, and reported in literature, so far.
Table 4. Summary of the mechanical properties of mainly martensitic matrix composites produced via LBAM reported in literature, to date.
The LPBF-fabricated martensitic matrix composites generally achieve a high tensile strength at the expense of ductility, as the SiC/18Ni300 and TiC/AISI420 SMCs demonstrate. With incorporation of appropriate content of reinforcements, the tensile strength of martensitic matrix composites can be enhanced up to 1600 MPa. The common strengthening mechanisms in martensitic matrix composites include: (i) grain boundary strengthening following the Hall-Petch relation (ΔσHP). (ii) Orowan mechanism (Δσor). (iii) dislocation strengthening (Δσdis), and (iv) load-bearing strengthening (ΔσLT). Tan et al. [Citation156] demonstrated that solidified martensitic structures were not refined by additions of reinforcements, which is unlike the 316L-based SMCs where SiC [Citation113], TiB2 [Citation112] or TiC [Citation234] additions significantly refined the grain size of the austenite matrix. The underlying reasons could be the following: (i) The LBAM-fabricated austenitic 316L microstructure is almost fully composed of FCC phase and the respective grains are tens of microns in size [Citation113,Citation234]. This is much larger than grains constituting the microstructure of LBAM-fabricated martensitic matrix steel (only a couple of microns). As a consequence, further grain refinement of the martensitic matrix is challenging to achieve when compared to 316L. (ii) The addition of reinforcements, such as TiC and SiC, promoted formation of the FCC phase, which has a much larger grain size than the BCC phase. In this particular case, the average grain size of the microstructure is increased, due to the presence of a large fraction of FCC phase in the microstructure of the respective SMCs which also consists of BCC phase further refined by reinforcements. Hence, grain boundary strengthening is not the main contributor to the strength of these martensitic matrix composites. In a study on LPBF-fabricated SiC/18Ni300 SMCs, the increase in yield strength Δσy was theoretically calculated from the sum of each strengthening contribution (Δσy = Δσor+Δσdis+ΔσLT) to be 334 MPa, which is slightly above the experimentally determined result of 262 MPa. The possible reason for this difference could be the high austenite content in the SiC/18Ni300 SMCs leading to a reduced YS of the matrix. A higher fraction of austenite can also be beneficial for the plasticity of SMC, as studies on WC/1.2767L [Citation149] and TiC/15-5PH SMCs [Citation207] report. Owing to the formation of FCC-structured austenite, a strong transformation-induced plasticity (TRIP) effect can occur [Citation235] during deformation. The austenite retained in the martensitic matrix transforms then to martensite, entails an increasing work-hardening rate and the strain resulting from the deformation-induced transformation contributes to a larger total elongation. Thus, a simultaneous improvement in the strength and ductility of SMCs can be achieved.
LBAM-fabricated SMCs with a martensite matrix also exhibit an ultrahigh hardness and wear resistance in comparison to their steel counterparts. For example, the hardness of LPBF-fabricated TiN/AISI420 SMCs (56.7 HRC) is about 11.8% higher than that of the AISI420 stainless steel counterparts (HRC 50.7) [Citation236]. Another fitting example is LDED-fabricated TiC/18Ni300 SMCs showing a higher hardness of ∼444 HV when compared to the 18Ni300 steel counterparts with a hardness of ∼368 HV [Citation193]. TiC-reinforced H13 composites fabricated by LPBF showed a much higher Vickers hardness of 811-858 HV [Citation89] when compared to H13 steel counterparts with hardness of ∼670 HV [Citation237]. An ultrahigh hardness is usually accompanied by excellent wear resistance. The wear rate can be effectively lowered by ∼26% after incorporating 15 vol.% TiC into the H13 matrix (4.9 × 10−6 mm3 Nm−1 for H13 steel and 3.6 × 10−6 mm3 Nm−1 for SMCs [Citation89]). The improvement in wear property by incorporation of reinforcements can be ascribed to altered wear mechanisms. Almangour et al. [Citation89] revealed different wear mechanisms effective in H13 steel and the H13-based SMC reinforced with 15 vol.% TiC, both fabricated by LPBF (see ). Elemental maps from the worn surface of H13 steel samples displayed the presence of iron, carbon, and chromium (a). The EDS map of the worn surface of the corresponding SMC sample indicated the presence of more iron, but less carbon (b), suggesting a small fraction of TiC particles to be worn off. The TiC particles appeared to protect the matrix during testing from wear. The worn surface of the SMC only displays pits stemming from spalled carbides which generated mini-grooves. No presence of oxides was detected after sliding suggesting that there was no significant temperature increase at the sample surface during testing. Thus, the improved wear performance was mainly attributed to the presence of uniformly dispersed nanoparticles reinforcing the steel matrix. Their additional low interfacial stress with the matrix also contributed to less breakage of the SMCs during sliding. Hot isostatic pressing (HIP) was shown to further increase the hardness of LPBF-fabricated SMCs with martensite matrix (see ) [Citation91]. However, these HIP-treated SMCs were characterized to largely fluctuate in hardness which can be attributed to the two inverse effects of the HIP treatment [Citation90]: For the positive effect, the bonding between the particles is improved which resulted in higher density and thus enhanced mechanical properties. The negative effect is the coarsening of the microstructure caused by the high temperature of the annealing treatment which deteriorates the mechanical properties of the SMCs.
Figure 38. SEM micrographs of the worn surface and the corresponding elemental maps of LPBF-fabricated (a) pure H13 steel and (b) 15% TiC/H13 steel matrix composite. Reprinted with permission from [Citation89].
![Figure 38. SEM micrographs of the worn surface and the corresponding elemental maps of LPBF-fabricated (a) pure H13 steel and (b) 15% TiC/H13 steel matrix composite. Reprinted with permission from [Citation89].](/cms/asset/2ef7464d-8973-4c4e-8a64-657ec67f09ce/yimr_a_2258664_f0038_oc.jpg)
Duplex austenitic/martensitic matrix composites
Due to the diphasic microstructure feature, duplex austenitic/martensitic matrix composites show multiple strengthening mechanisms, thus exhibiting an excellent combination of strength and ductility. With 3 wt.% micron-sized TiC (0.5–21 μm) addition, the LPBF-fabricated 316L-based SMCs exhibit a high tensile strength of ∼1032 MPa, combined with an excellent elongation of ∼29% [Citation107]. The nano-sized (∼60 nm) TiC/316L SMCs prepared via LPBF also show good combination of tensile strength (∼988 MPa) and elongation (∼32%) [Citation238]. Similarly, the LDED-fabricated TiC/15-5PH SMCs show appealing tensile properties with a high tensile strength of ~1398 MPa and a large fracture strain of ∼16.9% at room temperature [Citation207].
Compared to other types of SMCs fabricated via LBAM, the duplex austenitic/martensitic matrix composites exhibit a prominent TRIP effect during deformation, due to their higher volume fraction of austenite. Besides the common strengthening mechanism such as grain boundary strengthening, dislocation strengthening, load-bearing strengthening and Orowan strengthening, TRIP effect is an important strengthening mechanism contributing to both strength and ductility. Chen et al. [Citation207] have demonstrated that the progressive TRIP effect in LDED-fabricated TiC/15-5PH SMCs provided by the retained austeniote (RA) is an important toughening behaviour, and obvious enhancement of work-hardening induced by TRIP before necking can be achieved. Most of the RA grains are directly transformed to martensite after tensile deformation at room temperature, which demonstrates a pronounced TRIP effect. The phase transformation behaviour of the TiC/15-5PH composites subjected to different strain levels are provided in The RA grains have various sizes before deformation (a). The fraction of RA remains almost constant over the entire elastic and plastic yield regimes (b,c). After straining to ϵ = 5% and 7% (d,e), most of the blocky RA grains show partial transformation into martensite to promote the TRIP effect and a considerable reduction in grain size, and the resulting hardening prevents local damage, thereby allowing higher elongation before necking [Citation239,Citation240]. When SMC specimens are deformed to fracture, only a few of the larger RA grains can be detected. These results suggest that larger RA grains, at first experience plastic deformation (0–3% strain) and only after higher deformation (above 3% strain) gradual martensitic transformation occurs. It is worth noting that the RA in 15-5PH/TiC composites will only transform during plastic deformation, but will not transform spontaneously due to sufficient C and Ti element content in RA [Citation239].
Figure 39. Evolution of RA phase in LDED-ed2 wt.% TiC/15-5PH SMC specimen during tensile tests when the specimens are globally strained to (a) 0%, (b) 1%, (c) 3%, (d) 5%, (e) 7%, and (f) ultimate fracture. Reprinted with permission from [Citation207].
![Figure 39. Evolution of RA phase in LDED-ed2 wt.% TiC/15-5PH SMC specimen during tensile tests when the specimens are globally strained to (a) 0%, (b) 1%, (c) 3%, (d) 5%, (e) 7%, and (f) ultimate fracture. Reprinted with permission from [Citation207].](/cms/asset/8652d5f2-98e4-4912-bc9a-bafb445beb4b/yimr_a_2258664_f0039_oc.jpg)
Besides good strength and toughness, the duplex austenitic/martensitic matrix composites also exhibit high hardness and wear resistance. Recent literature [Citation88] reported that the 15 vol.% nano-sized TiC/316L SMCs show a high microhardness of ∼403 HV and low coefficient of friction (COF) of 0.69 as well as a low wear rate of 3.1 × 10−4 mm3 Nm−1, which can be attributed to the refined microstructure, uniformly distributed TiC reinforcements and good compromise in deformation in duplex microstructure.
Ferritic matrix composites
Since ferritic steel is rare to be applied as matrix for SMCs, studies about the mechanical properties of ferritic matrix composites fabricated via LBAM are very limited. Compared to single ferritic steel, LBAM-fabricated ferritic matrix composites show improved mechanical properties, as substantiated by the following selected works. Both LPBF- and LDED-fabricated ferritic matrix composites reinforced with nano-sized Y2O3 display higher compressive strength compared to their counterparts [Citation101,Citation134]; the LPBF-fabricated nano-SiC/pure-Fe SMCs show a simultaneous improvement of tensile strength (∼764 MPa) and elongation (∼10.4%) compared to the as-fabricated pure iron (~357 MPa, ~9%) [Citation13]; Incorporation of nano-Cr3C2 into pure Fe achieves a drastically enhanced tensile strength of ∼1158 MPa, albeit at the expense of ductility with a low elongation of ∼2.1% [Citation96]; The hardness of the PBLS-fabricated ferritic matrix composites was increased with the incorporation of 2 wt.% multiwalled carbon nanotubes (MWNTs) or graphene oxide to about 600 HV [Citation98,Citation100], which is caused by the strong end contact interaction (i.e. σ-bonding) between the CNT/graphene-incorporations and the matrix [Citation220]. Further investigation is needed to understand the strengthening mechanisms in these ferritic matrix composites.
Conclusions
Steel matrix composites (SMCs) are in the focus of intense research efforts within recent years, because of their exceptional mechanical properties which are superior to steels. In particular, the combination of high specific modulus, high strength, appealing toughness and excellent wear resistance is advantageous. In comparison to steels, SMCs are therefore more suited as safety-critical key components across various application fields such as automobile, aerospace, moulds and nuclear industry. SMCs are traditionally prepared by casting and powder metallurgy. However, the expensive, geometrically complex casting moulds, limited interfacial bonding between the reinforcing particles and the steel matrix, and, particularly, the high difficulty in achieving the uniform distribution of the reinforcing particles within the matrix restrict the application of SMCs. The innovative laser-based additive manufacturing (LBAM) technologies exactly allow to overcome these limitations. Furthermore, complex-shaped SMC parts are fabricated in a layer-by-layer manner by processing powder mixtures via LBAM enabling the fabrication of SMCs with reinforcing particles uniformly embedded in the steel matrix. The present work provides a comprehensive review on the current research progress in high-performance SMCs prepared by LBAM inclusive of the feedstock preparation, microstructures and mechanical properties for LBAM of SMCs.
The selection of reinforcements in LBAM of SMCs should be conducted considering the laser absorptivity, thermal conductivity and coefficient of thermal expansion (CTE) of the reinforcements as well as the wettability between the reinforcing particles and the matrix. Different combinations of the steel matrix and reinforcements cause also different phase composition and microstructural features in the LBAM-fabricated SMCs along with different mechanical properties. So far, feedstock of steel matrix composite powder for processing via LBAM is commercially not available. Therefore, different preparation methods have been used to produce the SMC powder based on the material, shape and size of reinforcements incorporated into the steel powder. Direct mixing, ball milling also with in-situ reaction, agent deposition and laser-aided dielectrophoretic deposition are the most used preparation methods of SMC powder for LBAM. The best method must be identified for the preparation of each powder blend requiring high processability. Ensuring a good flowability and good dispersion of reinforcements is the main concern in the choice of feedstock preparation. This is the prerequisite for the LBAM-fabrication of dense SMCs with desired microstructure and mechanical performance. While the other requirements such as cost and efficiency should be also considered.
The incorporation of reinforcements strongly affects the microstructure of the SMCs fabricated by LBAM. During LBAM processing of SMCs, the reinforcements dramatically affect the solidification behaviour of the molten pool (e.g. thermal gradient, cooling rate, solidification rate and melt flow behaviour), thus posing great influence on the grain growth mechanisms and texture development of the steel matrix. Solidification and solid-state phase transformation of the steel matrix can be also altered by incorporating specific reinforcements (e.g. the duplex austenitic/martensitic matrix obtained in TiC/316L SMCs with a large difference in CTE between the reinforcement and the steel matrix). Consequently, numerous combinations of the steel matrix and reinforcements lead to the variability of phase composition, grain morphology and texture features for the SMCs fabricated via LBAM. Therefore, optimization of material, shape, size and content of reinforcements is necessary for different kinds of steel matrix, including austenitic, martensitic, duplex and ferritic matrix steel. This also provides a feasible strategy for microstructure control of SMCs parts to achieve tailored performance. It is worth noting that the laser energy density is also a key factor to microstructure formation of LBAM-fabricated SMCs. The synergetic effect of the laser energy density and addition of reinforcements on the microstructure of the SMCs should be considered to produce high-performance SMCs via LBAM.
The strength of the SMCs manufactured by LBAM is significantly higher than the unreinforced steel counterparts, due to multiple strengthening mechanisms including Orowan strengthening, grain-boundary strengthening, dislocation strengthening, load-transfer effect and segregation strengthening. The ductility, however, is often found to be inferior to the steel counterpart. For achieving a simultaneous improvement in strength and ductility, it is crucial to obtain a hierarchical microstructure involving refined microstructure, precipitation of nanosized particles, formation of non-equilibrium grain boundaries, solid-solution strengthening and the transformation-induced plasticity (TRIP) effect, e.g. the LPBF-fabricated WC/1.2767L SMCs. The incorporation of reinforcements also greatly enhances the hardness and wear resistance of LBAM-fabricated SMCs by inducing strong precipitation strengthening as well as grain-boundary strengthening. Thus, it is important to utilize the reinforcements to activate different kinds of strengthening and toughening mechanisms in the SMCs, so that the mechanical properties of the LBAM-fabricated SMCs can be greatly improved. In order to facilitate the strengthening and toughening effect in LBAM-fabricated SMCs, the triggering conditions of the strengthening and toughening mechanisms and their contributions to strength and ductility are recommended to be revealed at first.
Challenges, future trends and perspectives
The reviewed LBAM processes (LPBF, LDED and PBLS) show great potential in producing near-net-shape SMC parts. Various reported examples prove that dense SMC parts with designed microstructure and hence mechanical properties can be fabricated. However, there are still many challenges ahead and new solutions for LBAM of SMCs are required to overcome them. We believe the most significant future trends in the development of SMCs to be the following:
So far, the number of SMCs which can be processed via LBAM is very limited. While over 1000 steels were developed for conventional manufacturing (casting, forging, machining, etc.), there is only a scarce number of steels used as matrix for LBAM of SMCs by researchers and even less by industry, to date. To be more precise, the number of qualified Fe-based alloys is restricted to only pure iron, 316L, AISI420, maraging 300, H13, 1.2767L and PM2000 steels. Further efforts must be made to literally design new steels for the matrix of SMCs. Such steels and the resulting blends must better withstand the harsh processing conditions effective during LBAM, so that highly dense SMCs with even further enhanced mechanical properties can be fabricated.
We believe the low energy ball milling for the preparation methods of powder mixtures to further prevail, because they are cost-effective and easy to realize. It does not require multiple synthesis steps as for instance in the laser-aided dielectrophoretic deposition and also permits the preparation of SMCs with several types of reinforcing particles. Consequently, future attention will be given towards further optimization of the processing conditions enabling the preparation of composite powders with good flowability and uniformly distributed reinforcing particles.
In designing new steel compositions for the matrix of SMCs, the type of reinforcement can be exploited as a tool for designing the microstructure of the steel matrix. In other words, further trends in the preparation of the SMC powder mixtures will be directed towards the selection of the reinforcement(s) which can be based on the crystallographic information, as it is known for Al-based MMCs or just the mechanical or wear properties which the reinforcement imparts on the SMC. One example would be WC with high hardness and the resulting SMCs could be used as cutting tools because of the enhanced abrasive wear resistance. Cr3C2 is known for providing good sliding wear properties which is required for e.g. bearing components. Future research activities will focus on opening up the usage of new reinforcing particle types and their combination to LBAM-fabricate novel SMCs. Another selection criterion is just the chemical composition of the reinforcement. In particular, carbides are an interesting choice, because they give access to the phase components of the steel matrix. Not only the size and volume fraction of the reinforcement, but also its exact composition can be utilized to design high-performance SMCs via LBAM and we term this novel approach as ‘carbide engineering’. Except for the processing conditions, mainly the C content determines whether the steel matrix of the SMCs will be austenitic, martensitic, a mixture of both or ferritic by controlling the martensite start and finish temperatures. The C content of the steel melt can be, however, indirectly modulated by the type and fraction of the reinforcement. Thus, a quantitative interrelationship between carbide reinforcement characteristics and phase components of SMCs should be established in the near feature. We believe that the development of this novel approach ‘carbide engineering’ in LBAM fabricating SMCs will contribute to precisely tailor the microstructure and mechanical properties of SMCs.
There are multiple material-specific parameters such as, steel matrix/reinforcement classes, morphology, content and size of reinforcing particles, and process parameters such as, laser power, scanning speed, powder feed rate, layer thickness, scanning strategy, which must be identified for the successful fabrication of defect-free, high-performance SMCs with near-net shape. The microstructure, evolving thermal and residual stress gradients and mechanical properties of the SMCs depend on the processing parameters and interaction among them. Thus, the selection of the ideal processing parameter set would allow for the design of LBAM components with desired microstructure and hence mechanical performance. Therefore, the optimal values must be identified for a vast number of processing parameters (over 130 parameters [Citation241]). Next to experimentation guided parameter identification and statistical methods, approaches based on machine learning (ML) can be exploited to facilitate their identification [Citation242]. Given a sufficient large training dataset consisting of features (material and processing parameters) and labels (desired property, e.g. hardness and strength), this innovative technology can emulate all interdependent variables between the given features and labels. The developed ML model could suggest an optimum parameter set for the fabrication of SMCs with the envisaged microstructure and performance. Thus, tedious efforts required for identifying this parameter set with numerous experiments could be significantly mitigated. Suitable datasets must be generated for the construction of ML models. Since this a resource-intensive task, ML algorithms such as Gaussian Process regression which are suited to small datasets are desired. This is nowadays required, because datasets reported in literature are, unfortunately, not useful for the construction of ML models with high predictive accuracy. They are created at different testing procedures and methodologies, so that their consolidation is extremely challenging. As solution, we suggest the development of standard procedures which would also facilitate the AM community to interpret the data more efficiently and prevent experimental duplication. It is believed that this innovative ML-based approach has the potential to reduce resource consumption and, hence, increase the sustainability of LBAM-processing.
For the mechanical properties of LBAM-fabricated SMCs, the existing literature only reported their static mechanical properties, such as hardness, wear property, tensile and compression properties. Key dynamic mechanical properties such as fatigue, creep and anti-impact properties are scarcely reported. These properties are also important, since they determine the working life of SMCs. Metallurgical bonding between the layers in LBAM-fabricated parts usually acts as source for fracture when subjected to dynamic loading, so that it is necessary to further study the relationship between processing, interlayer microstructure and the dynamic mechanical properties of the resulting LBAM-fabricated SMCs.
Further investigations are required to clarify the strengthening mechanisms of LBAM-fabricated SMCs. The analysis on the contribution of different strengthening mechanisms in SMCs is still limited. Next to experiments, we believe multi-scale and multi-physics simulations could be proposed, since they can significantly contribute to understand the deformation mechanisms and resulting mechanical properties of LBAM-fabricated SMCs. Simulations can provide a theoretical foundation for predicting and maybe tailoring the mechanical properties of SMCs fabricated via LBAM.
In order for customized SMCs to find their way into commercial use, not only their mechanical performance and underlying deformation mechanisms, but further properties such as e.g. the corrosion resistance also at elevated temperatures are required to be investigated in-depth. In particular, there are only a limited number of publications with focus on the corrosion resistance of SMCs available, so that more research efforts are required along this line.
Disclosure statement
No potential conflict of interest was reported by the author(s).
Additional information
Funding
References
- Lu K. The future of metals. Science. 2010;328:319–320. doi:10.1126/science.1185866
- Raabe D, Tasan CC, Olivetti EA. Strategies for improving the sustainability of structural metals. Nature. 2019;575:64–74. doi:10.1038/s41586-019-1702-5
- Chen R, Li B, Li Y, et al. Influences of particle fraction and characteristics on damage tolerance of TiB2-reinforced steel matrix composites. Mater Sci Eng A. 2021;823:141736. doi:10.1016/j.msea.2021.141736
- Weigelt C, Schmidt G, Aneziris CG, et al. Compressive and tensile deformation behaviour of TRIP steel-matrix composite materials with reinforcing additions of zirconia and/or aluminium titanate. J Alloys Compd. 2017;695:9–20. doi:10.1016/j.jallcom.2016.10.176
- Hlushko K, Keckes J, Ressel G, et al. Dark-field X-ray microscopy reveals mosaicity and strain gradients across sub-surface TiC and TiN particles in steel matrix composites. Scr Mater. 2020;187:402–406. doi:10.1016/j.scriptamat.2020.06.053
- Tu XX, Xiao LR, Cai ZY, et al. Effects of vibration aging on residual stress and performance of instrument-grade TiC reinforced steel matrix composite. Mater Lett. 2022;325:132829. doi:10.1016/j.matlet.2022.132829
- Tu X, Xiao L, Zhao X, et al. Microstructure and nanoindentation creep behavior of TiC reinforced steel matrix composite after stabilizing heat treatments. Ceram Int. 2022;48:24733–24744. doi:10.1016/j.ceramint.2022.05.122
- Aparicio-Fernández R, Springer H, Szczepaniak A, et al. In-situ metal matrix composite steels: effect of alloying and annealing on morphology, structure and mechanical properties of TiB2 particle containing high modulus steels. Acta Mater. 2016;107:38–48. doi:10.1016/j.actamat.2016.01.048
- Yang Y, Wang H, Liang Y, et al. Fabrication of steel matrix composites locally reinforced with different ratios of TiC/TiB2 particulates using SHS reactions of Ni–Ti–B4C and Ni–Ti–B4C–C systems during casting. Mater Sci Eng A. 2007;445–446:398–404. doi:10.1016/j.msea.2006.09.062
- Tjong SC. Recent progress in the development and properties of novel metal matrix nanocomposites reinforced with carbon nanotubes and graphene nanosheets. Mater Sci Eng R Reports. 2013;74:281–350. doi:10.1016/j.mser.2013.08.001
- Radhamani AV, Lau HC, Ramakrishna S. CNT-reinforced metal and steel nanocomposites: A comprehensive assessment of progress and future directions. Compos Part A. 2018;114:170–187. doi:10.1016/j.compositesa.2018.08.010
- Wang BG, Wang GD, Misra RDK, et al. Increased hot-formability and grain-refinement by dynamic recrystallization of ferrite in an in situ TiB2 reinforced steel matrix composite. Mater Sci Eng A. 2021;812:141100. doi:10.1016/j.msea.2021.141100
- Qiu F, Zhang H, Li C-L, et al. Simultaneously enhanced strength and toughness of cast medium carbon steels matrix composites by trace nano-sized TiC particles. Mater Sci Eng A. 2021;819:141485. doi:10.1016/j.msea.2021.141485
- Chawla KK. Metal matrix composites: science and engineering. Cham: Springer International Publishing; 2019. p. 199–249. doi:10.1007/978-3-030-28983-6_6
- Moya JS, Lopez-Esteban S, Pecharromán C. The challenge of ceramic/metal microcomposites and nanocomposites. Prog Mater Sci. 2007;52:1017–1090. doi:10.1016/j.pmatsci.2006.09.003
- Guan D, He X, Zhang R, et al. Microstructure and tensile properties of in situ polymer-derived particles reinforced steel matrix composites produced by powder metallurgy method. Mater Sci Eng A. 2017;705:231–238. doi:10.1016/j.msea.2017.07.084
- Chen H, Gu D, Deng L, et al. Laser additive manufactured high-performance Fe-based composites with unique strengthening structure. J Mater Sci Technol. 2021;89:242–252. doi:10.1016/j.jmst.2020.04.011
- Campbell J. Complete casting handbook: metal casting processes, metallurgy, techniques and design. Oxford: Butterworth-Heinemann; 2015.
- Pauly S, Wang P, Kühn U, et al. Experimental determination of cooling rates in selectively laser-melted eutectic Al-33Cu. Addit Manuf. 2018;22:753–757. doi:10.1016/j.addma.2018.05.034
- Özer G, Kisasöz A. The role of heat treatments on wear behaviour of 316L stainless steel produced by additive manufacturing. Mater Lett. 2022;327:133014. doi:10.1016/j.matlet.2022.133014.
- Riquelme A, Sánchez de Rojas Candela C, Rodrigo P, et al. Influence of process parameters in additive manufacturing of highly reinforced 316L/SiCp composites. J Mater Process Technol. 2022;299:117325. doi:10.1016/j.jmatprotec.2021.117325.
- Özer G, Karaaslan A. A study on the effects of different heat-treatment parameters on microstructure–mechanical properties and corrosion behavior of maraging steel produced by direct metal laser sintering. Steel Res Int. 2020;91:1–8. doi:10.1002/srin.202000195
- Guo L, Zhang L, Andersson J, et al. Additive manufacturing of 18% nickel maraging steels: defect, structure and mechanical properties: a review. J Mater Sci Technol. 2022;120:227–252. doi:10.1016/j.jmst.2021.10.056
- Hou H, Simsek E, Stasak D, et al. Elastocaloric cooling of additive manufactured shape memory alloys with large latent heat. J Phys D Appl Phys. 2017;50:404001. doi:10.1088/1361-6463/aa85bf.
- Dadbakhsh S, Speirs M, Van Humbeeck J, et al. Laser additive manufacturing of bulk and porous shape-memory NiTi alloys: from processes to potential biomedical applications. MRS Bull. 2016;41:765–774. doi:10.1557/mrs.2016.209
- Frazier WE. Metal additive manufacturing: a review. J Mater Eng Perform. 2014;23:1917–1928. doi:10.1007/s11665-014-0958-z
- Van Humbeeck J. Additive manufacturing of shape memory alloys. Shape Mem Superelasticity. 2018;4:309–312. doi:10.1007/s40830-018-0174-z
- Babacan N, Pauly S, Gustmann T. Laser powder bed fusion of a superelastic Cu-Al-Mn shape memory alloy. Mater Des. 2021;203:109625. doi:10.1016/j.matdes.2021.109625
- Ferretto I, Kim D, Della Ventura NM, et al. Laser powder bed fusion of a Fe–Mn–Si shape memory alloy. Addit Manuf. 2021;46:102071. doi:10.1016/j.addma.2021.102071
- Hou H, Simsek E, Ma T, et al. Fatigue-resistant high-performance elastocaloric materials made by additive manufacturing. Science. 2019;366:1116–1121. doi:10.1126/science.aax7616
- Haberland C, Elahinia M, Walker JM, et al. On the development of high quality NiTi shape memory and pseudoelastic parts by additive manufacturing. Smart Mater Struct. 2014;23:104002. doi:10.1088/0964-1726/23/10/104002.
- Tang S, Ummethala R, Suryanarayana C, et al. Additive manufacturing of aluminum-based metal matrix composites—a review. Adv Eng Mater. 2021;23:2100053. doi:10.1002/adem.202100053
- Wang Z, Ummethala R, Singh N, et al. Selective laser melting of aluminum and its alloys. Materials (Basel). 2020;13:4564. doi:10.3390/ma13204564
- Yu WH, Sing SL, Chua CK, et al. Particle-reinforced metal matrix nanocomposites fabricated by selective laser melting: a state of the art review. Prog Mater Sci. 2019;104:330–379. doi:10.1016/j.pmatsci.2019.04.006
- Boes J, Röttger A, Becker L, et al. Processing of gas-nitrided AISI 316L steel powder by laser powder bed fusion – microstructure and properties. Addit Manuf. 2019;30:100836. doi:10.1016/j.addma.2019.100836
- Ogawa F, Masuda C. Fabrication and the mechanical and physical properties of nanocarbon-reinforced light metal matrix composites: a review and future directions. Mater Sci Eng A. 2021;820:141542. doi:10.1016/j.msea.2021.141542
- Gao C, Wang Z, Xiao Z, et al. Selective laser melting of TiN nanoparticle-reinforced AlSi10Mg composite: microstructural, interfacial, and mechanical properties. J Mater Process Technol. 2020;281:116618. doi:10.1016/j.jmatprotec.2020.116618
- Wang M, Song B, Wei Q, et al. Improved mechanical properties of AlSi7Mg/nano-SiCp composites fabricated by selective laser melting. J Alloys Compd. 2019;810:151926. doi:10.1016/j.jallcom.2019.151926
- Xi L, Guo S, Gu D, et al. Microstructure development, tribological property and underlying mechanism of laser additive manufactured submicro-TiB2 reinforced Al-based composites. J Alloys Compd. 2020;819:152980. doi:10.1016/j.jallcom.2019.152980
- Zhang D, Yi D, Wu X, et al. Sic reinforced AlSi10Mg composites fabricated by selective laser melting. J Alloys Compd. 2022;894:162365. doi:10.1016/j.jallcom.2021.162365
- Xi L, Gu D, Guo S, et al. Grain refinement in laser manufactured Al-based composites with TiB2 ceramic. J Mater Res Technol. 2020;9:2611–2622. doi:10.1016/j.jmrt.2020.04.059
- Zhao X, Gu D, Ma C, et al. Microstructure characteristics and its formation mechanism of selective laser melting SiC reinforced Al-based composites. Vacuum. 2019;160:189–196. doi:10.1016/j.vacuum.2018.11.022
- Xie H, Zhang J, Li F, et al. Selective laser melting of SiCp/Al composites: densification, microstructure, and mechanical and tribological properties. Ceram Int. 2021;47:30826–30837. doi:10.1016/j.ceramint.2021.07.263
- Chang F, Gu D, Dai D, et al. Selective laser melting of in-situ Al4SiC4 + SiC hybrid reinforced Al matrix composites: influence of starting SiC particle size. Surf Coatings Technol. 2015;272:15–24. doi:10.1016/j.surfcoat.2015.04.029
- Yan Q, Chen B, Li JS. Super-high-strength graphene/titanium composites fabricated by selective laser melting. Carbon. 2021;174:451–462. doi:10.1016/j.carbon.2020.12.047
- Zhao Y, Wu C, Zhou S, et al. Selective laser melting of Ti-TiN composites: formation mechanism and corrosion behaviour in H2SO4/HCl mixed solution. J Alloys Compd. 2021;863:158721. doi:10.1016/j.jallcom.2021.158721
- Zhou Z, Liu Y, Liu X, et al. Microstructure evolution and mechanical properties of in-situ Ti6Al4V–TiB composites manufactured by selective laser melting. Compos Part B. 2021;207:108567. doi:10.1016/j.compositesb.2020.108567
- Xi L, Ding K, Gu D, et al. Interfacial structure and wear properties of selective laser melted Ti/(TiC + TiN) composites with high content of reinforcements. J Alloys Compd. 2021;870:159436. doi:10.1016/j.jallcom.2021.159436
- Gu D, Chen H, Dai D, et al. Carbon nanotubes enabled laser 3D printing of high-performance titanium with highly concentrated reinforcement. IScience. 2020;23:101498. doi:10.1016/j.isci.2020.101498
- Gu D, Hagedorn YC, Meiners W, et al. Selective laser melting of in-situ TiC/Ti5Si3 composites with novel reinforcement architecture and elevated performance. Surf Coatings Technol. 2011;205:3285–3292. doi:10.1016/j.surfcoat.2010.11.051
- Gu D, Hagedorn YC, Meiners W, et al. Nanocrystalline TiC reinforced Ti matrix bulk-form nanocomposites by selective laser melting (SLM): densification, growth mechanism and wear behavior. Compos Sci Technol. 2011;71:1612–1620. doi:10.1016/j.compscitech.2011.07.010
- Niessen F, Gazder AA, Hald J, et al. Multiscale in-situ studies of strain-induced martensite formation in inter-critically annealed extra-low-carbon martensitic stainless steel. Acta Mater. 2021;220:117339. doi:10.1016/j.actamat.2021.117339
- Chen H, Gu D, Kosiba K, et al. Achieving high strength and high ductility in WC-reinforced iron-based composites by laser additive manufacturing. Addit Manuf. 2020;35:101195. doi:10.1016/j.addma.2020.101195
- Li R, Liu J, Shi Y, et al. Balling behavior of stainless steel and nickel powder during selective laser melting process. Int J Adv Manuf Technol. 2012;59:1025–1035. doi:10.1007/s00170-011-3566-1
- yu Chen H, dong Gu D, Ge Q, et al. Role of laser scan strategies in defect control, microstructural evolution and mechanical properties of steel matrix composites prepared by laser additive manufacturing. Int J Miner Metall Mater. 2021;28:462–474. doi:10.1007/s12613-020-2133-x
- Chen H, Gu D. Effect of metallurgical defect and phase transition on geometric accuracy and wear resistance of iron-based parts fabricated by selective laser melting. J Mater Res. 2016;31:1477–1490. doi:10.1557/jmr.2016.132
- Boegelein T, Dryepondt SN, Pandey A, et al. Mechanical response and deformation mechanisms of ferritic oxide dispersion strengthened steel structures produced by selective laser melting. Acta Mater. 2015;87:201–215. doi:10.1016/j.actamat.2014.12.047
- Garibaldi M, Ashcroft I, Simonelli M, et al. Metallurgy of high-silicon steel parts produced using selective laser melting. Acta Mater. 2016;110:207–216. doi:10.1016/j.actamat.2016.03.037
- Qiu C, Adkins NJE, Attallah MM. Selective laser melting of invar 36: microstructure and properties. Acta Mater. 2016;103:382–395. doi:10.1016/j.actamat.2015.10.020
- Fang Y, Kim MK, Zhang Y, et al. Particulate-reinforced iron-based metal matrix composites fabricated by selective laser melting: a systematic review. J Manuf Process. 2022;74:592–639. doi:10.1016/j.jmapro.2021.12.018
- Fayazfar H, Salarian M, Rogalsky A, et al. A critical review of powder-based additive manufacturing of ferrous alloys: process parameters, microstructure and mechanical properties. Mater Des. 2018;144:98–128. doi:10.1016/j.matdes.2018.02.018
- Bajaj P, Hariharan A, Kini A, et al. Steels in additive manufacturing: a review of their microstructure and properties. Mater Sci Eng A. 2020;772:138633. doi:10.1016/j.msea.2019.138633.
- Herzog D, Seyda V, Wycisk E, et al. Additive manufacturing of metals. Acta Mater. 2016;117:371–392. doi:10.1016/j.actamat.2016.07.019
- Mandal S, Sadeghianjahromi A, Wang CC. Experimental and numerical investigations on molten metal atomization techniques – a critical review. Adv Powder Technol. 2022;33:103809. doi:10.1016/j.apt.2022.103809
- Jang T-S, Kim D, Han G, et al. Powder based additive manufacturing for biomedical application of titanium and its alloys: a review. Biomed Eng Lett. 2020;10:505–516. doi:10.1007/s13534-020-00177-2
- Averardi A, Cola C, Zeltmann SE, et al. Effect of particle size distribution on the packing of powder beds: a critical discussion relevant to additive manufacturing. Mater Today Commun. 2020;24:100964. doi:10.1016/j.mtcomm.2020.100964
- Yang M, Dai Y, Song C, et al. Microstructure evolution of grey cast iron powder by high pressure gas atomization. J Mater Process Technol. 2010;210:351–355. doi:10.1016/j.jmatprotec.2009.09.023
- Li R, Shi Y, Wang Z, et al. Densification behavior of gas and water atomized 316L stainless steel powder during selective laser melting. Appl Surf Sci. 2010;256:4350–4356. doi:10.1016/j.apsusc.2010.02.030
- Snow Z, Nassar AR, Reutzel EW. Invited review article: review of the formation and impact of flaws in powder bed fusion additive manufacturing. Addit Manuf. 2020;36:101457. doi:10.1016/j.addma.2020.101457
- Rabin BH, Smolik GR, Korth GE. Characterization of entrapped gases in rapidly solidified powders. Mater Sci Eng A. 1990;124:1–7. doi:10.1016/0921-5093(90)90328-Z
- Zhang LC, Xu WY, Li Z, et al. Mechanism of rapidly solidified satellites formation in gas atomized powders: simulation and characterization. Powder Technol. 2023;418:118162. doi:10.1016/j.powtec.2022.118162
- Xiong L, Chuang AC, Thomas J, et al. Defect and satellite characteristics of additive manufacturing metal powders. Adv Powder Technol. 2022;33:103486. doi:10.1016/j.apt.2022.103486
- Gu D, Yuan P. Thermal evolution behavior and fluid dynamics during laser additive manufacturing of Al-based nanocomposites: underlying role of reinforcement weight fraction. J Appl Phys. 2015;118:233109. doi:10.1063/1.4937905
- Vasantgadkar NA, Bhandarkar UV, Joshi SS. A finite element model to predict the ablation depth in pulsed laser ablation. Thin Solid Films. 2010;519:1421–1430. doi:10.1016/j.tsf.2010.09.016
- Samant AN, Du B, Dahotre NB. In-situ surface absorptivity prediction during 1.06 μm wavelength laser low aspect ratio machining of structural ceramics. Phys Status Solidi. 2009;206:1433–1439. doi:10.1002/pssa.200925108
- Chen H, Gu D, Dai D, et al. Microstructure and composition homogeneity, tensile property, and underlying thermal physical mechanism of selective laser melting tool steel parts. Mater Sci Eng A. 2017;682:279–289. doi:10.1016/j.msea.2016.11.047
- AlMangour B, Grzesiak D, Cheng J, et al. Thermal behavior of the molten pool, microstructural evolution, and tribological performance during selective laser melting of TiC/316L stainless steel nanocomposites: experimental and simulation methods. J Mater Process Technol. 2018;257:288–301. doi:10.1016/j.jmatprotec.2018.01.028
- Kim KJ, Kim Y-W, Lim K-Y, et al. Electrical and thermal properties of SiC–AlN ceramics without sintering additives. J Eur Ceram Soc. 2015;35:2715–2721. doi:10.1016/j.jeurceramsoc.2015.04.010
- Prass GS, d’Oliveira ASCM. Processing and characterization of AISI 316L coatings modified with Cu and CuO nanoparticles. Surf Coatings Technol. 2023;461:129465. doi:10.1016/j.surfcoat.2023.129465
- Wei C, Chueh Y-H, Zhang X, et al. Easy-to-remove composite support material and procedure in additive manufacturing of metallic components using multiple material laser-based powder bed fusion. J Manuf Sci Eng. 2019;141:071002. doi:10.1115/1.4043536.
- Kim J, Kang S. Elastic and thermo-physical properties of TiC, TiN, and their intermediate composition alloys using ab initio calculations. J Alloys Compd. 2012;528:20–27. doi:10.1016/j.jallcom.2012.02.124
- Taya M, Hayashi S, Kobayashi AS, et al. Toughening of a particulate-reinforced ceramic-matrix composite by thermal residual stress. J Am Ceram Soc. 1990;73:1382–1391. doi:10.1111/j.1151-2916.1990.tb05209.x
- Rong Y, Huang Y, Xu J, et al. Numerical simulation and experiment analysis of angular distortion and residual stress in hybrid laser-magnetic welding. J Mater Process Technol. 2017;245:270–277. doi:10.1016/j.jmatprotec.2017.02.031
- Sanaty-Zadeh A. Comparison between current models for the strength of particulate-reinforced metal matrix nanocomposites with emphasis on consideration of Hall-Petch effect. Mater Sci Eng A. 2012;531:112–118. doi:10.1016/j.msea.2011.10.043
- Song B, Dong S, Coddet P, et al. Microstructure and tensile behavior of hybrid nano-micro SiC reinforced iron matrix composites produced by selective laser melting. J Alloys Compd. 2013;579:415–421. doi:10.1016/j.jallcom.2013.06.087
- Wei Q, Li S, Han C, et al. Selective laser melting of stainless-steel/nano-hydroxyapatite composites for medical applications: microstructure, element distribution, crack and mechanical properties. J Mater Process Technol. 2015;222:444–453. doi:10.1016/j.jmatprotec.2015.02.010
- Yan X, Huang C, Chen C, et al. Additive manufacturing of WC reinforced maraging steel 300 composites by cold spraying and selective laser melting. Surf Coatings Technol. 2019;371:161–171. doi:10.1016/j.surfcoat.2018.03.072
- AlMangour B, Grzesiak D, Yang J-M. Selective laser melting of TiC reinforced 316L stainless steel matrix nanocomposites: influence of starting TiC particle size and volume content. Mater Des. 2016;104:141–151. doi:10.1016/j.matdes.2016.05.018
- AlMangour B, Grzesiak D, Yang JM. Nanocrystalline TiC-reinforced H13 steel matrix nanocomposites fabricated by selective laser melting. Mater Des. 2016;96:150–161. doi:10.1016/j.matdes.2016.02.022
- AlMangour B, Grzesiak D, Yang JM. Selective laser melting of TiB2/316L stainless steel composites: the roles of powder preparation and hot isostatic pressing post-treatment. Powder Technol. 2017;309:37–48. doi:10.1016/j.powtec.2016.12.073
- AlMangour B, Grzesiak D, Yang JM. Selective laser melting of TiB2/H13 steel nanocomposites: influence of hot isostatic pressing post-treatment. J Mater Process Technol. 2017;244:344–353. doi:10.1016/j.jmatprotec.2017.01.019
- Li B, Qian B, Xu Y, et al. Additive manufacturing of ultrafine-grained austenitic stainless steel matrix composite via vanadium carbide reinforcement addition and selective laser melting: formation mechanism and strengthening effect. Mater Sci Eng A. 2019;745:495–508. doi:10.1016/j.msea.2019.01.008
- Zhao X, Wei QS, Gao N, et al. Rapid fabrication of TiN/AISI 420 stainless steel composite by selective laser melting additive manufacturing. J Mater Process Technol. 2019;270:8–19. doi:10.1016/j.jmatprotec.2019.01.028
- Zheng Z, Wang L, Jia M, et al. Microstructure and mechanical properties of stainless steel/calcium silicate composites manufactured by selective laser melting. Mater Sci Eng C. 2017;71:1099–1105. doi:10.1016/j.msec.2016.11.032
- Salman O, Funk A, Waske A, et al. Additive manufacturing of a 316L steel matrix composite reinforced with CeO2 particles: process optimization by adjusting the laser scanning speed. Technologies. 2018;6:25. doi:10.3390/technologies6010025
- Song B, Wang Z, Yan Q, et al. Integral method of preparation and fabrication of metal matrix composite: selective laser melting of in-situ nano/submicro-sized carbides reinforced iron matrix composites. Mater Sci Eng A. 2017;707:478–487. doi:10.1016/j.msea.2017.09.092
- AlMangour B, Grzesiak D, Yang JM. In situ formation of TiC-particle-reinforced stainless steel matrix nanocomposites during ball milling: feedstock powder preparation for selective laser melting at various energy densities. Powder Technol. 2018;326:467–478. doi:10.1016/j.powtec.2017.11.064
- Lin D, Richard Liu C, Cheng GJ. Laser sintering of separated and uniformly distributed multiwall carbon nanotubes integrated iron nanocomposites. J Appl Phys. 2014;115. doi:10.1063/1.4869214
- Ouyang W, Xu Z, Jia S, et al. Multilayer-graphene reinforced 316L matrix composites preparation by laser deposited additive manufacturing: microstructure and mechanical property analysis. Mater Res Express. 2019;6:096557. doi:10.1088/2053-1591/ab2f2e.
- Lin D, Richard Liu C, Cheng GJ. Single-layer graphene oxide reinforced metal matrix composites by laser sintering: microstructure and mechanical property enhancement. Acta Mater. 2014;80:183–193. doi:10.1016/j.actamat.2014.07.038
- Doñate-Buendía C, Frömel F, Wilms MB, et al. Oxide dispersion-strengthened alloys generated by laser metal deposition of laser-generated nanoparticle-metal powder composites. Mater Des. 2018;154:360–369. doi:10.1016/j.matdes.2018.05.044
- Kang N, Ma W, Li F, et al. Microstructure and wear properties of selective laser melted WC reinforced 18Ni-300 steel matrix composite. Vacuum. 2018;154:69–74. doi:10.1016/j.vacuum.2018.04.044
- Behera MP, Dougherty T, Singamneni S. Selective laser melting of stainless steel and silicon nitride fibre metal matrix composites. Proc Inst Mech Eng Part B J Eng Manuf. 2020;234:1513–1525. doi:10.1177/0954405420928684
- Li X, Willy HJ, Chang S, et al. Selective laser melting of stainless steel and alumina composite: experimental and simulation studies on processing parameters, microstructure and mechanical properties. Mater Des. 2018;145:1–10. doi:10.1016/j.matdes.2018.02.050
- Zhai W, Zhou W, Nai SML, et al. Characterization of nanoparticle mixed 316 L powder for additive manufacturing. J Mater Sci Technol. 2020;47:162–168. doi:10.1016/j.jmst.2020.02.019
- Suryanarayana C. Mechanical alloying: a critical review. Mater Res Lett. 2022;10:619–647. doi:10.1080/21663831.2022.2075243
- Zhai W, Zhu Z, Zhou W, et al. Selective laser melting of dispersed TiC particles strengthened 316L stainless steel. Compos Part B. 2020;199:108291. doi:10.1016/j.compositesb.2020.108291
- Suryanarayana C. Mechanical alloying and milling. Prog Mater Sci. 2001;46:1–184. doi:10.1016/S0079-6425(99)00010-9
- Suryanarayana C, Al-Aqeeli N. Mechanically alloyed nanocomposites. Prog Mater Sci. 2013;58:383–502. doi:10.1016/j.pmatsci.2012.10.001
- Zhang M, Li C, Gao Q, et al. The effect of heat treatment on microstructure and properties of laser-deposited TiC reinforced H13 steel matrix composites. Optik. 2020;206:164286. doi:10.1016/j.ijleo.2020.164286
- Han Q, Setchi R, Evans SL. Synthesis and characterisation of advanced ball-milled Al-Al2O3 nanocomposites for selective laser melting. Powder Technol. 2016;297:183–192. doi:10.1016/j.powtec.2016.04.015
- AlMangour B, Kim YK, Grzesiak D, et al. Novel TiB2-reinforced 316L stainless steel nanocomposites with excellent room- and high-temperature yield strength developed by additive manufacturing. Compos Part B. 2019;156:51–63. doi:10.1016/j.compositesb.2018.07.050
- Zou Y, Tan C, Qiu Z, et al. Additively manufactured SiC-reinforced stainless steel with excellent strength and wear resistance. Addit Manuf. 2021;41:101971. doi:10.1016/j.addma.2021.101971
- Liu Y, Tang M, Hu Q, et al. Densification behavior, microstructural evolution, and mechanical properties of TiC/AISI420 stainless steel composites fabricated by selective laser melting. Mater Des. 2020;187:1–13. doi:10.1016/j.matdes.2019.108381
- Ertugrul O, Enrici TM, Paydas H, et al. Laser cladding of TiC reinforced 316L stainless steel composites: feedstock powder preparation and microstructural evaluation. Powder Technol. 2020;375:384–396. doi:10.1016/j.powtec.2020.07.100
- Matin MA, Lu L, Gupta M. Investigation of the reactions between boron and titanium compounds with magnesium. Scr Mater. 2001;45:479–486. doi:10.1016/S1359-6462(01)01059-4
- Wang X, Jha A, Brydson R. In situ fabrication of Al3Ti particle reinforced aluminium alloy metal–matrix composites. Mater Sci Eng A. 2004;364:339–345. doi:10.1016/j.msea.2003.08.049
- Tjong SC. Novel nanoparticle-reinforced metal matrix composites with enhanced mechanical properties. Adv Eng Mater. 2007;9:639–652. doi:10.1002/adem.200700106
- Saheb N, Iqbal Z, Khalil A, et al. Spark plasma sintering of metals and metal matrix nanocomposites: a review. J Nanomater. 2012;2012:983470. doi:10.1155/2012/983470.
- Wang HY, Jiang QC, Li XL, et al. In situ synthesis of TiC/Mg composites in molten magnesium. Scr Mater. 2003;48:1349–1354. doi:10.1016/S1359-6462(03)00014-9
- Krasnowski M, Kulik T. Nanocrystalline FeAl–TiN composites obtained by hot-pressing consolidation of reactively milled powders. Scr Mater. 2007;57:553–556. doi:10.1016/j.scriptamat.2007.04.031
- Tjong SC, Ma ZY. Microstructural and mechanical characteristics of in situ metal matrix composites. Mater Sci Eng R Reports. 2000;29:49–113. doi:10.1016/S0927-796X(00)00024-3
- AlMangour B, Grzesiak D, Yang JM. In-situ formation of novel TiC-particle-reinforced 316L stainless steel bulk-form composites by selective laser melting. J Alloys Compd. 2017;706:409–418. doi:10.1016/j.jallcom.2017.01.149
- Dadbakhsh S, Hao L. Effect of Al alloys on selective laser melting behaviour and microstructure of in situ formed particle reinforced composites. J Alloys Compd. 2012;541:328–334. doi:10.1016/j.jallcom.2012.06.097
- Hwang JY, Neira A, Scharf TW, et al. Laser-deposited carbon nanotube reinforced nickel matrix composites. Scr Mater. 2008;59:487–490. doi:10.1016/j.scriptamat.2008.04.032
- Yasin G, Khan MA, Arif M, et al. Synthesis of spheres-like Ni/graphene nanocomposite as an efficient anti-corrosive coating; effect of graphene content on its morphology and mechanical properties. J Alloys Compd. 2018;755:79–88. doi:10.1016/j.jallcom.2018.04.321
- Jin B, Xiong D-B, Tan Z, et al. Enhanced corrosion resistance in metal matrix composites assembled from graphene encapsulated copper nanoflakes. Carbon. 2019;142:482–490. doi:10.1016/j.carbon.2018.10.088
- Nieto A, Bisht A, Lahiri D, et al. Graphene reinforced metal and ceramic matrix composites: a review. Int Mater Rev. 2017;62:241–302. doi:10.1080/09506608.2016.1219481
- Huang X, Yin Z, Wu S, et al. Graphene-based materials: synthesis, characterization, properties, and applications. Small. 2011;7:1876–1902. doi:10.1002/smll.201002009
- Chu L, Shi J, de Cursi ES. Vibration analysis of vacancy defected graphene sheets by Monte Carlo based finite element method. Nanomaterials. 2018;8:489. doi:10.3390/nano8070489.
- Lin D, Ye C, Liao Y, et al. Mechanism of fatigue performance enhancement in a laser sintered superhard nanoparticles reinforced nanocomposite followed by laser shock peening. J Appl Phys. 2013;113:133509. doi:10.1063/1.4799154
- Lin D, Suslov S, Ye C, et al. Laser assisted embedding of nanoparticles into metallic materials. Appl Surf Sci. 2012;258:2289–2296. doi:10.1016/j.apsusc.2011.09.132
- Fischer P, Romano V, Weber HP, et al. Sintering of commercially pure titanium powder with a Nd:YAG laser source. Acta Mater. 2003;51:1651–1662. doi:10.1016/S1359-6454(02)00567-0
- Doñate-Buendia C, Kürnsteiner P, Stern F, et al. Microstructure formation and mechanical properties of ODS steels built by laser additive manufacturing of nanoparticle coated iron-chromium powders. Acta Mater. 2021;206:116566. doi:10.1016/j.actamat.2020.116566.
- Zhang D, Gökce B, Barcikowski S. Laser synthesis and processing of colloids: fundamentals and applications. Chem Rev. 2017;117:3990–4103. doi:10.1021/acs.chemrev.6b00468
- Schmitz T, Wiedwald U, Dubs C, et al. Ultrasmall yttrium iron garnet nanoparticles with high coercivity at low temperature synthesized by laser ablation and fragmentation of pressed powders. ChemPhysChem. 2017;18:1125–1132. doi:10.1002/cphc.201601183
- Wagener P, Barcikowski S. Laser fragmentation of organic microparticles into colloidal nanoparticles in a free liquid jet. Appl Phys A Mater Sci Process. 2010;101:435–439. doi:10.1007/s00339-010-5814-x
- Tsuji T, Iryo K, Watanabe N, et al. Preparation of silver nanoparticles by laser ablation in solution: influence of laser wavelength on particle size. Appl Surf Sci. 2002;202:80–85. doi:10.1016/S0169-4332(02)00936-4
- Kong CY, Scudamore RJ, Allen J. High-rate laser metal deposition of Inconel 718 component using low heat-input approach. Phys Procedia. 2010;5:379–386. doi:10.1016/j.phpro.2010.08.159
- Reichardt A, Shapiro AA, Otis R, et al. Advances in additive manufacturing of metal-based functionally graded materials. Int Mater Rev. 2021;66:1–29. doi:10.1080/09506608.2019.1709354
- Thomas M, Baxter GJ, Todd I. Normalised model-based processing diagrams for additive layer manufacture of engineering alloys. Acta Mater. 2016;108:26–35. doi:10.1016/j.actamat.2016.02.025
- AlMangour B, Grzesiak D, Borkar T, et al. Densification behavior, microstructural evolution, and mechanical properties of TiC/316L stainless steel nanocomposites fabricated by selective laser melting. Mater Des. 2018;138:119–128. doi:10.1016/j.matdes.2017.10.039
- Hofmeister W, Griffith M. Solidification in direct metal deposition by LENS processing. JOM. 2001;53:30–34. doi:10.1007/s11837-001-0066-z
- Simchi A. Direct laser sintering of metal powders: mechanism, kinetics and microstructural features. Mater Sci Eng A. 2006;428:148–158. doi:10.1016/j.msea.2006.04.117
- Cheng L, DeZun W, YunXu L, et al. Composition design of a new type low-alloy high-strength steel. Mater Des. 1997;18:53–59. doi:10.1016/S0261-3069(97)00029-0
- Borm P, Klaessig FC, Landry TD, et al. Research strategies for safety evaluation of nanomaterials, part V: role of dissolution in biological fate and effects of nanoscale particles. Toxicol Sci. 2006;90:23–32. doi:10.1093/toxsci/kfj084
- Chen L, Sun Y, Li L, et al. Microstructure evolution, mechanical properties, and strengthening mechanism of TiC reinforced Inconel 625 nanocomposites fabricated by selective laser melting. Mater Sci Eng A. 2020;792:139655. doi:10.1016/j.msea.2020.139655
- Chen L, Sun Y, Li L, et al. In situ TiC/inconel 625 nanocomposites fabricated by selective laser melting: densification behavior, microstructure evolution, and wear properties. Appl Surf Sci. 2020;518:145981. doi:10.1016/j.apsusc.2020.145981
- Chen H, Gu D, Zhang H, et al. Novel WC-reinforced iron-based composites with excellent mechanical properties synthesized by laser additive manufacturing: underlying role of reinforcement weight fraction. J Mater Process Technol. 2021;289:116959. doi:10.1016/j.jmatprotec.2020.116959
- van Bohemen SMC. Bainite and martensite start temperature calculated with exponential carbon dependence. Mater Sci Technol. 2012;28:487–495. doi:10.1179/1743284711Y.0000000097
- Axén N, Zum Gahr K-H. Abrasive wear of TiC-steel composite clad layers on tool steel. Wear. 1992;157:189–201. doi:10.1016/0043-1648(92)90197-G
- Sun Z, Xu Y, Chen F, et al. Effects of ion irradiation on microstructure of 316L stainless steel strengthened by disperse nano TiC through selective laser melting. Mater Charact. 2021;180:111420. doi:10.1016/j.matchar.2021.111420
- Zhai W, Zhou W, Nai SML. In-situ formation of TiC nanoparticles in selective laser melting of 316L with addition of micronsized TiC particles. Mater Sci Eng A. 2022;829:142179. doi:10.1016/j.msea.2021.142179
- Zhao S, Shen X, Yang J, et al. Densification behavior and mechanical properties of nanocrystalline TiC reinforced 316L stainless steel composite parts fabricated by selective laser melting. Opt Laser Technol. 2018;103:239–250. doi:10.1016/j.optlastec.2018.01.005
- Kou S. Welding metallurgy, second ed. New York: Wiley; 2003.
- Tan C, Zou J, Wang D, et al. Duplex strengthening via SiC addition and in-situ precipitation in additively manufactured composite materials. Compos Part B. 2022;236:109820. doi:10.1016/j.compositesb.2022.109820
- DebRoy T, Wei HL, Zuback JS, et al. Additive manufacturing of metallic components – process, structure and properties. Prog Mater Sci. 2018;92:112–224. doi:10.1016/j.pmatsci.2017.10.001
- Almangour B, Grzesiak D, Yang JM. Rapid fabrication of bulk-form TiB2/316L stainless steel nanocomposites with novel reinforcement architecture and improved performance by selective laser melting. J Alloys Compd. 2016;680:480–493. doi:10.1016/j.jallcom.2016.04.156
- Niu HJ, Chang ITH. Selective laser sintering of gas atomized M2 high speed steel powder. J Mater Sci. 2000;35:31–38. doi:10.1023/A:1004720011671
- Moyle M, Ledermueller C, Zou Z, et al. Multi-scale characterisation of microstructure and texture of 316L stainless steel manufactured by laser powder bed fusion. Mater Charact. 2022;184:111663. doi:10.1016/j.matchar.2021.111663
- Kunze K, Etter T, Grässlin J, et al. Texture, anisotropy in microstructure and mechanical properties of IN738LC alloy processed by selective laser melting (SLM). Mater Sci Eng A. 2015;620:213–222. doi:10.1016/j.msea.2014.10.003
- Andreau O, Koutiri I, Peyre P, et al. Texture control of 316L parts by modulation of the melt pool morphology in selective laser melting. J Mater Process Technol. 2019;264:21–31. doi:10.1016/j.jmatprotec.2018.08.049
- Kumar D, Shankar G, Prashanth KG, et al. Texture dependent strain hardening in additively manufactured stainless steel 316L. Mater Sci Eng A. 2021;820:141483. doi:10.1016/j.msea.2021.141483
- Kim TH, Baek GY, Jeon JB, et al. Effect of laser rescanning on microstructure and mechanical properties of direct energy deposited AISI 316L stainless steel. Surf Coatings Technol. 2021;405:126540. doi:10.1016/j.surfcoat.2020.126540
- Aboulkhair NT, Simonelli M, Parry L, et al. 3D printing of aluminium alloys: additive manufacturing of aluminium alloys using selective laser melting. Prog Mater Sci. 2019;106:100578. doi:10.1016/j.pmatsci.2019.100578
- Li J, Qu H, Bai J. Grain boundary engineering during the laser powder bed fusion of TiC/316L stainless steel composites: new mechanism for forming TiC-induced special grain boundaries. Acta Mater. 2022;226:117605. doi:10.1016/j.actamat.2021.117605
- Vasilyev AA, Sokolov SF, Kolbasnikov NG, et al. Effect of alloying on the self-diffusion activation energy in γ-iron. Phys Solid State. 2011;53: 2194–2200.
- Bokshteyn SZ. Diffusion and the structure of metals. Met Sci Heat Treat Met. 1961;3:473–480. doi:10.1007/BF00814533
- Wang J, Li L, Tao W. Crack initiation and propagation behavior of WC particles reinforced Fe-based metal matrix composite produced by laser melting deposition. Opt Laser Technol. 2016;82:170–182. doi:10.1016/j.optlastec.2016.03.008
- Zheng B, Haley JC, Yang N, et al. On the evolution of microstructure and defect control in 316L SS components fabricated via directed energy deposition. Mater Sci Eng A. 2019;764:138243. doi:10.1016/j.msea.2019.138243
- Wang Y, Liu Z, Zhou Y, et al. Microstructure and mechanical properties of TiN particles strengthened 316L steel prepared by laser melting deposition process. Mater Sci Eng A. 2021;814:141220. doi:10.1016/j.msea.2021.141220
- Kang N, Ma W, Heraud L, et al. Selective laser melting of tungsten carbide reinforced maraging steel composite. Addit Manuf. 2018;22:104–110. doi:10.1016/j.addma.2018.04.031
- Wang YM, Voisin T, McKeown JT, et al. Additively manufactured hierarchical stainless steels with high strength and ductility. Nat Mater. 2018;17:63–70. doi:10.1038/nmat5021
- Stefanescu DM, Dhindaw BK, Kacar SA, et al. Behavior of ceramic particles at the solid-liquid metal interface in metal matrix composites. Metall Trans A. 1988;19(11):2847–2855. doi:10.1007/BF02645819
- Rafi HK, Karthik NV, Gong H, et al. Microstructures and mechanical properties of Ti6Al4V parts fabricated by selective laser melting and electron beam melting. J Mater Eng Perform. 2013;22:3872–3883. doi:10.1007/s11665-013-0658-0
- Chen H, Gu D, Xiong J, et al. Improving additive manufacturing processability of hard-to-process overhanging structure by selective laser melting. J Mater Process Technol. 2017;250:99–108. doi:10.1016/j.jmatprotec.2017.06.044
- Sulima I, Klimczyk P, Malczewski P. Effect of TiB2 particles on the tribological properties of stainless steel matrix composites. Acta Metall Sin. 2014;27:12–18. doi:10.1007/s40195-013-0002-6
- Pan F, Zhang J, Chen HL, et al. Effects of rare earth metals on steel microstructures. Materials (Basel). 2016;9:1–19. doi:10.3390/ma9060417
- Sahoo SK, Sahoo BN, Panigrahi SK. Effect of in-situ sub-micron sized TiB2 reinforcement on microstructure and mechanical properties in ZE41 magnesium matrix composites. Mater Sci Eng A. 2020;773:138883. doi:10.1016/j.msea.2019.138883
- Sames WJ, List FA, Pannala S, et al. The metallurgy and processing science of metal additive manufacturing. Int Mater Rev. 2016;61:315–360. doi:10.1080/09506608.2015.1116649
- Lehmann T, Rose D, Ranjbar E, et al. Large-scale metal additive manufacturing: a holistic review of the state of the art and challenges. Int Mater Rev. 2022;67:410–459. doi:10.1080/09506608.2021.1971427
- Gu DD, Meiners W, Wissenbach K, et al. Laser additive manufacturing of metallic components: materials, processes and mechanisms. Int Mater Rev. 2012;57:133–164. doi:10.1179/1743280411Y.0000000014
- Zhang J, Zhang Y, Li W, et al. Microstructure and properties of functionally graded materials Ti6Al4V/TiC fabricated by direct laser deposition. Rapid Prototyp J. 2018;24:677–687. doi:10.1108/RPJ-12-2016-0215
- Balandin AA. Thermal properties of graphene and nanostructured carbon materials. Nat Mater. 2011;10:569–581. doi:10.1038/nmat3064
- Geim AK. Graphene: status and prospects. Science. 2009;324:1530–1534. doi:10.1126/science.1158877
- Zeisig J, Schädlich N, Giebeler L, et al. Microstructure and abrasive wear behavior of a novel FeCrMoVC laser cladding alloy for high-performance tool steels. Wear. 2017;382–383:107–112. doi:10.1016/j.wear.2017.04.021
- Ibrahim MF, Ammar HR, Samuel AM, et al. Metallurgical parameters controlling matrix/B4C particulate interaction in aluminium–boron carbide metal matrix composites. Int J Cast Met Res. 2013;26:364–373. doi:10.1179/1743133613Y.0000000074
- Wang X, Wang CC, Jiang DM, et al. Application of a sub-regular solution model in the interface reaction in metal matrix composites. Rare Metal Mat Eng. 2015;44:830–833. doi:10.1016/S1875-5372(15)30057-6
- Li L, Liu D, Chen Y, et al. Electron microscopy study of reaction layers between single-crystal WC particle and Ti–6Al–4 V after laser melt injection. Acta Mater. 2009;57:3606–3614. doi:10.1016/j.actamat.2009.04.021
- Rong T, Gu D, Shi Q, et al. Effects of tailored gradient interface on wear properties of WC/Inconel 718 composites using selective laser melting. Surf Coatings Technol. 2016;307:418–427. doi:10.1016/j.surfcoat.2016.09.011
- Rong T, Gu D. Formation of novel graded interface and its function on mechanical properties of WC1-x reinforced Inconel 718 composites processed by selective laser melting. J Alloys Compd. 2016;680:333–342. doi:10.1016/j.jallcom.2016.04.107
- Liu D, Li L, Li F, et al. WCp/Fe metal matrix composites produced by laser melt injection. Surf Coatings Technol. 2008;202:1771–1777. doi:10.1016/j.surfcoat.2007.07.053
- Hu J, Zhu H, Zhang J, et al. Effects of TiC addition on microstructure, microhardness and wear resistance of 18Ni300 maraging steel by direct laser deposition. J Mater Process Technol. 2021;296:117213. doi:10.1016/j.jmatprotec.2021.117213
- Jeong C, Kong BS, Shin JH, et al. Evaluation of thermal aging activation energies based on multi-scale mechanical property tests for an austenitic stainless steel weld beads. Mater Sci Eng A. 2022;835:142629. doi:10.1016/j.msea.2022.142629
- Augis JA, Bennett JE. Calculation of the Avrami parameters for heterogeneous solid state reactions using a modification of the Kissinger method. J Therm Anal. 1978;13:283–292. doi:10.1007/BF01912301
- Song B, Dong S, Coddet C. Rapid in situ fabrication of Fe/SiC bulk nanocomposites by selective laser melting directly from a mixed powder of microsized Fe and SiC. Scr Mater. 2014;75:90–93. doi:10.1016/j.scriptamat.2013.11.031
- Chen H, Gu D, Dai D, et al. A novel approach to direct preparation of complete lath martensite microstructure in tool steel by selective laser melting. Mater Lett. 2018;227:128–131. doi:10.1016/j.matlet.2018.05.042
- Weiland A, Hultman L, Wahlström U, et al. Internal stress and microstructure of SiC reinforced aluminium alloy 2014. Acta Mater. 1998;46:5271–5281. doi:10.1016/S1359-6454(98)00212-2
- Tan C, Zhou K, Kuang M, et al. Microstructural characterization and properties of selective laser melted maraging steel with different build directions. Sci Technol Adv Mater. 2018;19:746–758. doi:10.1080/14686996.2018.1527645
- Zhu HM, Zhang JW, Hu JP, et al. Effects of aging time on the microstructure and mechanical properties of laser-cladded 18Ni300 maraging steel. J Mater Sci. 2021;56:8835–8847. doi:10.1007/s10853-021-05841-1
- Oh NR, Lee SK, Hwang KC, et al. Characterization of microstructure and tensile fracture behavior in a novel infiltrated TiC-steel composite. Scr Mater. 2016;112:123–127. doi:10.1016/j.scriptamat.2015.09.028
- Muvvala G, Mullick S, Nath AK. Development of process maps based on molten pool thermal history during laser cladding of Inconel 718/TiC metal matrix composite coatings. Surf Coatings Technol. 2020;399:126100. doi:10.1016/j.surfcoat.2020.126100
- Yang S, Chen N, Liu W, et al. Fabrication of nickel composite coatings reinforced with TiC particles by laser cladding. Surf Coatings Technol. 2004;183:254–260. doi:10.1016/j.surfcoat.2003.09.062
- Jiang WH, Kovacevic R. Laser deposited TiC/H13 tool steel composite coatings and their erosion resistance. J Mater Process Technol. 2007;186:331–338. doi:10.1016/j.jmatprotec.2006.12.053
- Dadoo A, Boutorabi SMA, Kheirandish S. Effect of titanium carbide concentration on the morphology of MC carbides in pulsed laser surface alloyed AISI H13 tool steel. Opt Laser Technol. 2019;112:236–244. doi:10.1016/j.optlastec.2018.11.001
- Wu CL, Zhang S, Zhang CH, et al. Formation mechanism and phase evolution of in situ synthesizing TiC-reinforced 316L stainless steel matrix composites by laser melting deposition. Mater Lett. 2018;217:304–307. doi:10.1016/j.matlet.2018.01.097
- Chen W, Xiao B, Xu L, et al. Additive manufacturing of martensitic stainless steel matrix composites with simultaneously enhanced strength-ductility and corrosion resistance. Compos Part B. 2022;234:109745. doi:10.1016/j.compositesb.2022.109745
- DebRoy T, Mukherjee T, Milewski JO, et al. Scientific, technological and economic issues in metal printing and their solutions. Nat Mater. 2019;18:1026–1032. doi:10.1038/s41563-019-0408-2
- Gu D, Shi X, Poprawe R, et al. Material-structure-performance integrated laser-metal additive manufacturing. Science. 2021;372:eabg1487. doi:10.1126/science.abg1487
- Li Q, Lei Y, Fu H. Growth mechanism, distribution characteristics and reinforcing behavior of (Ti, Nb)C particle in laser cladded Fe-based composite coating. Appl Surf Sci. 2014;316:610–616. doi:10.1016/j.apsusc.2014.08.052
- Zhao C, Zhou Y, Xing X, et al. Precipitation stability and micro-property of (Nb, Ti)C carbides in MMC coating. J Alloys Compd. 2018;763:670–678. doi:10.1016/j.jallcom.2018.05.318
- Kürnsteiner P, Wilms MB, Weisheit A, et al. High-strength Damascus steel by additive manufacturing. Nature. 2020;582:515–519. doi:10.1038/s41586-020-2409-3
- Wu CL, Zhang S, Zhang CH, et al. Effects of SiC content on phase evolution and corrosion behavior of SiC-reinforced 316L stainless steel matrix composites by laser melting deposition. Opt Laser Technol. 2019;115:134–139. doi:10.1016/j.optlastec.2019.02.029
- Majumdar JD, Li L. Studies on direct laser cladding of SiC dispersed AISI 316L stainless steel. Metall Mater Trans A. 2009;40:3001. doi:10.1007/s11661-009-0018-8
- Lo KH, Kwok CT, Cheng FT, et al. Corrosion resistance of laser-fabricated metal–matrix composite layer on stainless steel 316L. J Laser Appl. 2003;15:107–114. doi:10.2351/1.1536649
- Wei HL, Mazumder J, DebRoy T. Evolution of solidification texture during additive manufacturing. Sci Rep. 2015;5:1–7. doi:10.1038/srep16446
- Zhang H, Huang Y, Ning H, et al. Processing and microstructure characterisation of oxide dispersion strengthened Fe–14Cr–0.4Ti–0.25Y2O3 ferritic steels fabricated by spark plasma sintering. J Nucl Mater. 2015;464:61–68. doi:10.1016/j.jnucmat.2015.04.029
- Boegelein T, Louvis E, Dawson K, et al. Characterisation of a complex thin walled structure fabricated by selective laser melting using a ferritic oxide dispersion strengthened steel. Mater Charact. 2016;112:30–40. doi:10.1016/j.matchar.2015.11.021
- Zhu Q, Qu S, Wang X, et al. Synthesis of Fe-based amorphous composite coatings with low purity materials by laser cladding. Appl Surf Sci. 2007;253:7060–7064. doi:10.1016/j.apsusc.2007.02.055
- Nieto A, Agarwal A, Lahiri D, et al. Carbon nanotubes reinforced metal matrix composites. Boca Raton, FL: CRC Press; 2011.
- Palacios JJ, Pérez-Jiménez AJ, Louis E, et al. First-Principles phase-coherent transport in metallic nanotubes with realistic contacts. Phys Rev Lett. 2003;90:106801. doi:10.1103/PhysRevLett.90.106801
- Wang D, Song C, Yang Y, et al. Investigation of crystal growth mechanism during selective laser melting and mechanical property characterization of 316L stainless steel parts. Mater Des. 2016;100:291–299. doi:10.1016/j.matdes.2016.03.111
- Gu D, Chen H. Selective laser melting of high strength and toughness stainless steel parts: the roles of laser hatch style and part placement strategy. Mater Sci Eng A. 2018;725:419–427. doi:10.1016/j.msea.2018.04.046
- Li XP, Ji G, Chen Z, et al. Selective laser melting of nano-TiB2decorated AlSi10Mg alloy with high fracture strength and ductility. Acta Mater. 2017;129:183–193. doi:10.1016/j.actamat.2017.02.062
- Raabe D, Herbig M, Sandlöbes S, et al. Grain boundary segregation engineering in metallic alloys: a pathway to the design of interfaces. Curr Opin Solid State Mater Sci. 2014;18:253–261. doi:10.1016/j.cossms.2014.06.002
- Weertman JR. Hall-Petch strengthening in nanocrystalline metals. Mater Sci Eng A. 1993;166:161–167. doi:10.1016/0921-5093(93)90319-A
- Goh CS, Wei J, Lee LC, et al. Properties and deformation behaviour of Mg–Y2O3 nanocomposites. Acta Mater. 2007;55:5115–5121. doi:10.1016/j.actamat.2007.05.032
- Ye Y, Ni Z, Huang C, et al. Constitutive model of elastic response for Fe-TiB2 composites. Mater Today Commun. 2022;33:104620. doi:10.1016/j.mtcomm.2022.104620
- Mori T, Tanaka K. Average stress in matrix and average elastic energy of materials with misfitting inclusions. Acta Metall. 1973;21:571–574. doi:10.1016/0001-6160(73)90064-3
- Zhang Y, Song B, Ming J, et al. Corrosion mechanism of amorphous alloy strengthened stainless steel composite fabricated by selective laser melting. Corros Sci. 2020;163:108241. doi:10.1016/j.corsci.2019.108241
- Li B, Wang T, Li P, et al. Selective laser melting of 316L stainless steel: influence of Co-Cr-Mo-W addition on corrosion resistance. Metals (Basel). 2021;11:597. doi:10.3390/met11040597
- Zhao Z, Li J, Bai P, et al. Microstructure and mechanical properties of TiC-reinforced 316L stainless steel composites fabricated using selective laser melting. Metals (Basel). 2019;9:267. doi:10.3390/met9020267
- Schaller RF, Mishra A, Rodelas JM, et al. The role of microstructure and surface finish on the corrosion of selective laser melted 304L. J Electrochem Soc. 2018;165:C234. doi:10.1149/2.0431805jes
- AlMangour B, Baek MS, Grzesiak D, et al. Strengthening of stainless steel by titanium carbide addition and grain refinement during selective laser melting. Mater Sci Eng A. 2018;712:812–818. doi:10.1016/j.msea.2017.11.126
- Song C, Wang H, Sun Z, et al. Effect of multiphase microstructure on fatigue crack propagation behavior in TRIP-assisted steels. Int J Fatigue. 2020;133:105425. doi:10.1016/j.ijfatigue.2019.105425
- Zhao X, Wei Q, Song B, et al. Fabrication and characterization of AISI 420 stainless steel using selective laser melting. Mater Manuf Process. 2015;30:1283–1289. doi:10.1080/10426914.2015.1026351
- Holzweissig MJ, Taube A, Brenne F, et al. Microstructural characterization and mechanical performance of hot work tool steel processed by selective laser melting. Metall Mater Trans B. 2015;46:545–549. doi:10.1007/s11663-014-0267-9
- Zhai W, Zhou W, Nai SML. Grain refinement and strengthening of 316L stainless steel through addition of TiC nanoparticles and selective laser melting. Mater Sci Eng A. 2022;832:142460. doi:10.1016/j.msea.2021.142460
- Dai Z, Chen H, Ding R, et al. Fundamentals and application of solid-state phase transformations for advanced high strength steels containing metastable retained austenite. Mater Sci Eng R Reports. 2021;143:100590. doi:10.1016/j.mser.2020.100590
- Wang M, Huang MX. Abnormal TRIP effect on the work hardening behavior of a quenching and partitioning steel at high strain rate. Acta Mater. 2020;188:551–559. doi:10.1016/j.actamat.2020.02.035
- King WE, Anderson AT, Ferencz RM, et al. Laser powder bed fusion additive manufacturing of metals; physics, computational, and materials challenges. Appl Phys Rev. 2015;2:041304. doi:10.1063/1.4937809
- Chernyavsky D, Kononenko DY, Han JH, et al. Machine learning for additive manufacturing: predicting materials characteristics and their uncertainty. Mater Des. 2023;227:111699. doi:10.1016/j.matdes.2023.111699