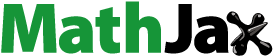
Abstract
Mejica BN, Ebert DA, Tanaka SK, Deas ML. 2023. Managing cyanobacteria with a water quality control curtain in Iron Gate Reservoir, California. Lake Reserv Manage. 39:291–310.
Iron Gate Reservoir, a eutrophic monomictic reservoir on the Klamath River, a Pacific coast river within the United States, stratifies thermally during summer and is subject to cyanobacteria blooms that produce microcystin toxins, causing public health concerns. An impermeable curtain was installed in Iron Gate Reservoir upstream of the powerhouse intake to control the withdrawal depth and improve water quality of releases to the downstream Klamath River. Thermal stratification minimizes vertical downward movement of cyanobacteria-laden near-surface waters, and the curtain was hypothesized to reduce cyanobacteria and toxin concentrations downstream of the curtain and dam. The Wedderburn number, which indicates unstable (mixed) or stratified conditions, was used to calculate mixed layer depths and define conditions under which the curtain would be likely to be effective. Changes in chlorophyll a, cyanobacteria gene, Microcystis spp. gene, and microcystin concentrations were used to quantify curtain effectiveness for different curtain depths and mixed layer depths. When curtain depth extended below the mixed layer, concentrations of total chlorophyll a, total cyanobacteria, total Microcystis spp., and total microcystin in waters downstream of the curtain were statistically significantly reduced by an average of 87, 90, 78, and 64%, respectively. Concentrations were not consistently reduced downstream of the curtain under other configurations. Findings indicate the curtain can be managed to take advantage of stratification within the epilimnion and address issues pertaining to downstream cyanobacteria and associated toxins, and should improve water quality and reduce public health notices for the 305.7 km of river downstream of Iron Gate Dam.
Strains of Microcystis spp. (e.g., Microcystis aeruginosa) found throughout the Klamath River Basin have been associated with high levels of microcystin (Kann and Corum Citation2006, Jacoby and Kann Citation2007, Kann and Corum Citation2009, Otten et al. Citation2015, Otten Citation2017), a widespread and potent cyanotoxin impacting public health and safety and water quality (Chorus and Bartram Citation1999, Van der Merwe Citation2014, Harke et al. Citation2016). Microcystins (e.g., microcystin-leucine arginine [LR]) are hepatotoxins (Chorus and Bartram Citation1999, Sivonen Citation2009, McLellan and Manderville Citation2017, WHO Citation2020) that can lead to health advisories in the Klamath River and reservoirs if toxin concentrations exceed public health standards in California (State Water Board Citation2016). Cyanotoxins can adversely affect mammals (Carmichael and Boyer Citation2016, McLellan and Manderville Citation2017) and may adversely affect aquatic vertebrates and invertebrates (Ferrão-Filho and Kozlowsky-Suzuki Citation2011, Bownik Citation2016) if concentrations are elevated.
Microcystis spp. and other cyanobacteria can control their buoyancy, and thus their vertical position in the water column, to maintain favorable conditions (e.g., remain or concentrate in optimal light conditions; Reynolds and Walsby Citation1975, Reynolds Citation1987, Reynolds et al. Citation1987, Walsby Citation1987, Graham et al. Citation2008). Cyanobacteria and associated toxins can be managed by physical controls (as opposed to chemical or biological controls) to reduce the frequency with which public health standards are exceeded in eutrophic waterbodies. Physical controls can include artificial mixing to minimize cyanobacterial blooms (Reynolds et al. Citation1983, Huisman et al. Citation2004, Visser et al. Citation2016), restricting the downstream movement of euphotic waters using selective withdrawal (Sabart et al. Citation2009), a water quality control curtain (Asaeda et al. Citation1996, Park et al. Citation2017) or mesh algal fence (Joo et al. Citation2003), or other means.
In Iron Gate Reservoir, Microcystis spp. and other cyanobacteria are more prevalent in near-surface waters than at deeper depths (Moisander PH, University of California Santa Cruz, 2008, unpubl. presentation). Genetic analysis of species composition within Iron Gate Reservoir and the Klamath River downstream of Iron Gate Dam suggests that phytoplankton were transported out of Iron Gate Reservoir with near-surface waters and that increasing withdrawal depth could lead to a reduction in cyanobacterial export from the reservoir (Otten et al. Citation2015).
This article explores the use of a variable-depth vertical water quality control curtain, installed in 2015 at a cost of about $1.6 million (USD), to isolate the mixed layer and reduce release of cyanobacteria from Iron Gate Reservoir to the Klamath River (Watercourse Citation2016). The mixed layer is characterized by lack of thermal stratification and may include only an upper layer of the epilimnion (when thermal epilimnetic stratification exists) or the entire epilimnion (when epilimnetic stratification is absent and seasonal parent stratification exists). The curtain takes advantage of persistent seasonal thermal stratification and frequent stratification within the epilimnion (late spring through fall) that coincide with periods of high cyanobacteria abundance, and cyanobacteria’s natural tendency to remain within the upper euphotic zone where they exploit density differences (i.e., thermal stratification) that minimize vertical mixing of cyanobacteria-laden near-surface waters with deeper waters (Reynolds Citation1987, Graham et al. Citation2008, Moisander PH, University of California Santa Cruz, 2008, unpubl. presentation). The curtain location and depth were designed to retain near-surface waters in the reservoir and release deeper waters containing less cyanobacteria and associated toxins to the downstream river (Watercourse Citation2016, Citation2017).
The curtain was hypothesized to be most effective at reducing cyanobacteria concentrations and associated toxins downstream of the curtain during periods of thermal stratification and curtain deployment to a depth below the mixed layer depth (h; the bottom of the mixed layer). In late spring and early summer, the developing seasonal parent thermocline is shallower (can occur between 5 m and 20 m), and thus, the curtain may be deployed below the entire epilimnion and/or below intermittent stratification within the epilimnion. As the season progresses, the parent thermocline generally deepens to a depth below the maximum curtain depth (>10.7 m), while shallower epilimnetic stratification (generally from the surface to 8 m) strengthens and occurs more frequently. Thus, deployment of the curtain to a depth below h often depends on the presence of intermittent epilimnetic stratification during summer through fall. The curtain was hypothesized to be less effective when deployed to depths shallower than h because under such conditions cyanobacteria could more readily be distributed below the curtain. The curtain was hypothesized to be ineffective when the curtain was not deployed (control).
Materials and methods
Continuous and discrete methods were used to collect data each year following curtain installation (2015–2021) to determine curtain effectiveness at reducing cyanobacteria and associated toxins in downstream waters (). Wedderburn numbers (Wn), calculated using continuous water temperature and meteorological data, were used to estimate stratification condition (i.e., h). A combination of h and deployed curtain depth was used to determine curtain condition. Hypotheses were assessed for different curtain conditions using 6 yr of discrete water quality data (total chlorophyll a [Chl-a] concentration, total cyanobacteria 16S rRNA gene concentration, total Microcystis spp. 16S rRNA gene concentration, and total microcystin concentration) collected at sites upstream and downstream of the curtain.
Table 1. Data collected in 2015–2021 used to determine curtain condition and to test curtain effectiveness.
Study site
Iron Gate Reservoir, located in northern California in the United States, is a eutrophic, monomictic reservoir on the mainstem Klamath River with a maximum depth of approximately 45 m, a total active storage of 65,330,035 m3, and a surface area of 3.80 km2 ( and ). Iron Gate Reservoir is formed by Iron Gate Dam, the lowermost dam of a series of hydroelectric dams that are part of the Lower Klamath River Hydroelectric Project on the Klamath River, a Pacific coast river that flows east to west through southern Oregon and northern California. Reservoir inflows originate from shallow, hypereutrophic Upper Klamath Lake (approximately 84.5 river km upstream), leading to eutrophic conditions within the reservoir (Oliver et al. Citation2014). Iron Gate Reservoir re-regulates the peaking flows from upstream power generation for release into the Klamath River downstream of Iron Gate Dam. During the summer and fall, when seasonal stratification occurs in the reservoir, extensive cyanobacteria blooms of Microcystis spp., Aphanizomenon flos-aquae, and other cyanobacteria species occur.
Figure 1. Klamath River from Upper Klamath Lake to the Pacific Ocean, and the dams of the Lower Klamath Hydroelectric Project including Iron Gate Dam.

Figure 2. Iron Gate Reservoir (left), project area (right), approximate fetch used in Wedderburn number calculations (gray dashed dotted line), and sampling locations.

The curtain location and design were based on several previous studies that investigated different approaches to isolate near-surface waters of Iron Gate Reservoir (Deas and Miao Citation2010, Watercourse Citation2013, Miao and Deas Citation2014, Watercourse Citation2015, Citation2016, Citation2017, PacifiCorp Citation2021). Algaecides, segregated coves, and similar prescriptions were explored to reduce algae concentrations within the reservoir and downstream of the reservoir. General findings were that treating this level of eutrophication was cost prohibitive at the reservoir-wide level, but that algaecide could be a component of a broader control strategy (Watercourse Citation2015), and a study reducing cyanobacteria downstream of Iron Gate Dam was the focus of this effort. Installed in 2015, the curtain spans approximately 245 m of the southwest corner of Iron Gate Reservoir approximately 75 m upstream of the powerhouse intake tower where maximum depth along the curtain is approximately 25.7 m (Watercourse Citation2016; and ). Seasonal stratification in the reservoir yields a typical summer epilimnion thickness of approximately 10–15 m, and epilimnetic stratification often occurs intermittently from late spring into early fall between approximately 0.1 m and 8 m. Hence, much of the water column in the vicinity of the curtain is within the epilimnion during seasonal stratification. The curtain was designed to be raised or lowered manually, extending from 1.5 m from the surface when fully furled to 10.7 m from the surface when fully deployed, herein referred to as design depth. The fully deployed depth of 10.7 m was designed to span much of the water column that is occupied by Microcystis spp. and to maintain low water velocities under the curtain (Watercourse Citation2017). While the intake tower draws reservoir waters from the surface down to 11 m, approximately, the curtain was designed to selectively draw deeper waters from the reservoir and preclude entrainment of near-surface waters (Watercourse Citation2016).
Figure 3. Iron Gate Reservoir upstream and downstream of the intake barrier curtain during deployment on 27 Jul 2017 (left) and Google Earth satellite image of Iron Gate Reservoir with intake barrier curtain deployment and downstream Klamath River on 23 Aug 2019 (right).

Although the fully furled curtain extended to a depth of 1.5 m, this depth was expected to be shallower than h and shallow enough that it would not materially reduce cyanobacteria or toxin concentrations downstream of the curtain in comparison to upstream of the curtain and served as the study control. Previous work in 2009 with an Acoustic Doppler Current Profiler (ADCP) in the vicinity of a 3 m curtain that was attached to the log boom (approximately 550 m upstream of Iron Gate Dam) demonstrated that waters readily passed under this shallow curtain (Deas and Miao Citation2010, Watercourse Citation2017). Local reservoir morphology and meteorology may result in cyanobacteria accumulations in the downstream end of the reservoir, but such conditions would probably extend well above curtain locations with similar conditions between the upstream of the curtain and downstream of the curtain sites, approximately 60–80 m apart.
Water quality sampling and monitoring
Sampling (water grab samples and temperature profiles) occurred immediately upstream and downstream of the curtain, and meteorological data were collected on Iron Gate Dam (, ). Grab samples (n = 502 upstream of the curtain and downstream of the curtain sample pairs) were collected in 2015–2020 and analyzed for total Chl-a concentration, total cyanobacteria 16S rRNA gene concentration, total Microcystis spp. 16S rRNA gene concentration, and total microcystin concentration to ascertain curtain efficacy at reducing cyanobacteria and toxin concentrations in relation to different combinations of h and curtain depths. Sampling at these sites began after curtain installation in 2015 and included periods when the curtain was fully furled (1.5 m) and deployed to various depths (ranging up to 10.7 m). Although the curtain design depth was 10.7 m, the curtain was raised to shallower depths throughout the late summer and fall during some years to address dissolved oxygen conditions in the Klamath River downstream of Iron Gate Dam. Concurrent work in the Klamath River downstream of Iron Gate Dam in 2019 demonstrated re-aeration of these waters (Watercourse Citation2020) above the habitat conservation plan (HCP) requirements (PacifiCorp Citation2012), and in 2019 and 2020, the curtain was managed to minimize cyanobacteria and related toxins in the Klamath River downstream of Iron Gate Dam, remaining at 10.7 m (design depth) for most or all of the season. Sampling occurred during the productive season (late Jun through Oct); most samples were collected during July through early September (samples were only collected on one day in Oct and on one day in Jun; ). Conditions each year were relatively similar with respect to onset and stability of stratification, reservoir stage, and meteorological conditions. Additionally, data collection over multiple years provided a temporal integration of conditions from the various bloom seasons. Cyanobacteria bloom timing and production levels varied, with blooms often occurring in late July through October. Floating platforms were installed at each of the sampling locations allowing for long-term deployment of monitoring equipment (e.g., autosamplers, profile samplers).
Grab samples were collected at discrete depths (i.e., 0.5, 1.5, 3.0, 6.0, 9.0, and 12 m) upstream and downstream of the curtain with a portable peristaltic pump in 2015–2020, and additional surface (0.5 m depth) samples were collected with a Teledyne ISCO 6712 portable autosampler in 2017 (). Exceptions include 2015 grab samples that were collected at 0.5 m, 12 m, and an integrated vertical sample from 0 to 8 m. Vertical profile samples were collected during the day and autosampler surface samples were collected through diel cycles. Autosamplers were programmed to take coincident samples at 4 h intervals over a 72 h period (24 Jul 2017–27 Jul 2017).
Grab samples were analyzed for total Chl-a concentration (micrograms per liter [µg/L]) using fluorescence detection, total cyanobacteria 16S rRNA gene concentration (gene copies per milliliter [gene copies/mL]) using real-time quantitative polymerase chain reaction (qPCR), total Microcystis spp. 16S rRNA gene concentration (gene copies/mL) using qPCR, and total microcystin concentration (µg/L) using enzyme-linked immunosorbent assay (ELISA). Total (unfiltered) microcystin included both free (not cell-bound) and cell-bound microcystin and is presented throughout. The 2015 total microcystin samples were analyzed by the United States Environmental Protection Agency Region 9 Laboratory in California, United States, using Envirologix ELISA kits, and 2016–2020 total microcystin samples were analyzed by Bend Genetics in California using Abraxis ADDA-MC ELISA kits. Although Chl-a is not specific to cyanobacteria species, Chl-a concentration is often used as a proxy for phytoplankton biomass. The total Microcystis spp. analysis using qPCR targeted a specific region of the phycocyanin gene and therefore included both toxigenic and nontoxic strains (Lyra et al. Citation2001, Tillett et al. Citation2001, Moisander et al. Citation2009). As many species of cyanobacteria, including Microcystis spp., are polyploidal (i.e., contain multiple gene copies per cell; Griese et al. Citation2011), total Microcystis spp. and total cyanobacteria gene concentrations are not directly comparable to cell concentrations.
Weighted thermograph chains with Onset U-22 Pro V2 temperature loggers at depths of 0.1, 0.5, 1.0, 1.5, 2.0, 3.0, 4.0, 5.0, 6.0, 8.0, 10, 15, and 20 m were deployed in 2016–2021 (). A logger at the 20 m depth was only deployed upstream of the curtain; the downstream of the curtain site was too shallow for a 20 m logger. Thermograph chains are a simple, low-resource intensive method to collect sufficient data to characterize thermal stratification. In 2015, YSI EXO2 multiparameter probes deployed from the reservoir sampling platforms collected temperature profiles continuously (15 min intervals) from approximately 0.2 to 12.0 m (approximate 1 m intervals), with 7 or 8 profiles collected daily. A meteorological station located on Iron Gate Dam collected meteorological data (15 min average wind speeds and air temperatures).
Data analysis
Grab sample data collected in 2015–2020 from upstream and downstream of the curtain sites (total Chl-a, total cyanobacteria, total Microcystis spp., and total microcystin concentrations) were paired based on sample date, sample depth, and constituent (n = 559 sample pairs for all constituents). Vertical profile grab sample pairs were collected on the same date approximately 1–2 h apart, while autosampler grab samples pairs were collected at the same date and time. Paired samples for various conditions (h and curtain depth) were used to determine effectiveness of the curtain at reducing downstream cyanobacteria concentrations.
Stratification and Wedderburn numbers
The mixed layer depth (h) was used to define conditions under which the curtain would be effective and was estimated using Wn, a dimensionless parameter that relates the stability of stratification (density and depth) to mixing energy (wind velocity) and represents short-term mixing patterns in the epilimnion (Fischer et al. Citation1979, Imberger and Hamblin Citation1982, Reynolds et al. Citation1987). Wn accounts for h, change in density (i.e., water temperature) between layers, shear stress acting on the water surface (i.e., wind), and fetch. Wn is calculated as
(1)
(1)
where Wn is the Wedderburn number, g′ is the reduced gravitational acceleration due to the density difference across the epilimnetic thermocline (m/s2), h is the depth of the mixed layer (m), u* is the characteristic shear velocity (m/s), and L is the fetch represented by the reservoir open water length in the direction of the wind (m).
Characteristic shear velocity, u* (m/s), is calculated as
(2)
(2)
where U is wind speed (m/s) at 10 m, ρA is density of air (kg/m3), ρw is density of water (kg/m3), and CD is the wind on water drag coefficient (Fischer et al. Citation1979). Reduced gravitational acceleration, g′ (m/s2), is a function of density differences across the zone of interest, often the epilimnetic thermocline in our case, and is calculated as
(3)
(3)
The fetch (L) was approximated as 3000 m, the longest line of sight in the vicinity of the curtain (). Winds measured on Iron Gate Dam demonstrate typical movement along the long axis of the lake with north-northeast or south-southwest winds that generally pick up in the afternoon through early evening. Hence, the estimated fetch was oriented in the north–south direction. Using the longest open water stretch in the reservoir as the fetch was a conservative estimate for Wn calculations; a longer fetch produces a smaller Wn at a specific depth and can produce a deeper h.
Wn < 1.0 indicates unstable (mixed) conditions that translate to an isothermal or near isothermal state between waters at different depths, whereas Wn > 1.0 indicates stability and unmixed waters (i.e., stratification; Imberger Citation1985, Reynolds et al. Citation1987, Spigel and Imberger Citation1987, Horne and Goldman Citation1994, Kalff Citation2002). When waters are stratified, the Wn transitions from <1.0 to >1.0 at the bottom of the mixed layer where the epilimnetic or parent thermocline occurs. The epilimnetic thermocline can fluctuate in thickness and vertical position over short timescales (i.e., hourly or daily), depending on density and wind energy.
To provide sub-daily stratification patterns, Wn values were calculated at hourly intervals using 2015–2021 hourly upstream of the curtain water temperature data, hourly wind speeds and air temperatures, and fetch. In 2015, water temperature data were not collected hourly, nor were they collected at each depth of interest; instead, water temperature data from the continuously profiling sonde were used to calculate Wn values when profile data existed (often daily or sub-daily) and data were interpolated to provide data at depths consistent with the thermograph chain data and Wn calculations from other years. All temperature data were converted to density data for Wn calculations. Wn values were calculated using water temperatures at the surface (0.5 m) and below the surface (e.g., 0.5 m vs. 1, 0.5 m vs. 1.5 m, 0.5 m vs. 2 m, etc.) down to 20 m. The Wn values at the 11 sequential depths were used to identify the depth where the Wn transitioned from unstable (Wn < 1.0) to stable (Wn > 1.0) and to estimate h, which was assumed to occur just above (0.25 to 0.5 m shallower than) the shallowest depth with Wn > 1.0.
Vertical thermal stratification profiles can be more complex than traditional textbook examples (e.g., multiple temporary thermoclines within the epilimnion, as discussed in Horne and Goldman [Citation1994]). To ensure identification of distinct stratification (temperature/density changes), secondary Wn values were also calculated using density (i.e., water temperature) differentials between each set of neighboring sampling depths (i.e., 0.5 m vs. 1 m, 1 m vs. 1.5 m, 1.5 m vs. 2 m, etc.) down to 20 m. The secondary mixed layer depth (h2) was assumed to occur just above (0.25 to 0.5 m shallower than) the shallowest depth with a secondary Wn > 1.0. Secondary Wn values were used to confirm h and provided insight into conditions when surface densities were not representative of the mixed layer, a gradual change in density with depth occurred, or multiple thermoclines existed and depth of epilimnetic stratification was not obvious. In nearly all instances the secondary Wn defined an h that was equal to or deeper than the h defined by the primary Wn.
Curtain condition and curtain effectiveness
Curtain condition was determined based on depth of the deployed curtain in relation to h (). The curtain condition was categorized as (C1) curtain deployed (>1.5 m) and curtain depth > h; (C2) curtain deployed and curtain at approximately the depth of h; (C3) curtain deployed and curtain depth < h; and (ND) curtain not deployed (curtain depth = 1.5 m; ). Sample data collected from the same depth on upstream and downstream sides of the curtain, paired for each collection date, were given the C1, C2, C3, or ND label based on the condition during the downstream curtain sample collection. Sets of sample pairs were compared to determine curtain effectiveness for each curtain condition. Sample pairs collected at least 0.5 m shallower than the deployed curtain during C1, C2, and C3 were compared, with subcategories analyzed based on depth of the sample pair in relation to h including (a) sample depth ≤ h (C1a, C2a, and C3a) and (b) sample depth > h (C1b, C2b, and C3b). Sample pairs collected from the approximate depth of the deployed curtain (within 0.1 m of the curtain, including samples at 3.0 m depth when the curtain was deployed to 3.0 m and at 6.0 m when the curtain was deployed to 6.1 m), as well as sample pairs collected just below the bottom of the deployed curtain (1.3–1.4 m below the curtain, including samples collected at 12 m depth when the curtain was deployed to 10.7 m, at 9 m when the curtain was deployed to 7.6 m, and at 6 m when curtain was deployed to 4.6 m), for all curtain conditions (C1, C2, or C3), were compared to check for leakage of cyanobacteria and toxins around and below the curtain. Additionally, sample pairs from all depths collected during ND conditions were compared (NDall; ).
Figure 4. Basic thermal structure of water column with epilimnetic stratification in vicinity of the curtain and Wedderburn number (Wn) within the epilimnion. Red represents warmer and blue cooler temperatures in the continous temperature scale

Figure 5. Same thermal conditions and different curtain depths (Z1, Z2, and Z3), with curtain conditions (C1, C2, and C3) based on curtain depth in relation to mixed layer depth (h). Curtain effectiveness at reducing cyanobacteria and toxins is expected to be highest during conditions present in the left panel, and to be reduced from left to right panels. Red represents warmer and blue cooler temperatures in the continous temperature scale.

Figure 6. Different thermal conditions, same curtain depth (Z1) in relation to the mixed layer depth (h). Curtain effectiveness at reducing cyanobacteria and toxins is expected to be highest during conditions present in the left panel, and less effective during conditions present in the right panel. Red represents warmer and blue cooler temperatures in the continous temperature scale.

Table 2. Curtain condition based on curtain deployment depth (zc) and mixed layer depth (h), and sample depth subcategory based on sample depth (zs) in relation to h.
Statistical analysis
Water quality data were not normally distributed, with cyanobacteria and toxin concentrations that changed through the season. Skewness for total Chl-a, total cyanobacteria, total Microcystis spp., and total microcystin 2015–2020 datasets analyzed ranged from 0.3 to 3.5 with a mean skewness of 2.1. Kurtosis for these same datasets ranged from −2.1 to 13.5 with a mean excessive kurtosis value of 4.7. Further, logical pairing existed within each sample group to compare data based on sample date and depth. To address these conditions a nonparametric sign test and nonparametric Wilcoxon signed-rank test for related samples were applied (Helsel and Hirch, Citation2002) to test differences between Iron Gate Reservoir upstream of the curtain and downstream of the curtain sample data. Tests were performed for each condition and sample depth subcategory.
One-tailed P values were reported; the direction of the difference was hypothesized based on the proportion of negative vs. positive differences (sign test) or the magnitude of the sum of positive ranks vs. the sum of negative ranks (Wilcoxon signed-rank test). For example, if there were more negative differences than positive differences, indicating reduction in concentration in the downstream direction occurred more often within a set of sample pairs, the hypothesis for the sign test was that upstream curtain concentrations were greater than downstream curtain concentrations. Similarly, if the Wilcoxon signed-rank test indicated the sum of negative ranks was greater than the sum of positive ranks, the hypothesis for this test was that upstream curtain concentrations were greater than downstream curtain concentrations. Differences were considered statistically significant (significant) when the P value was less than or equal to 0.05. Bias between each pair was calculated as the downstream value minus the upstream value, and the mean bias was reported for each constituent and condition with significant results or with sample sizes too small to test. Sample size was not considered except for sufficient sample size (i.e., minimum sample size was 5 pairs). Negative bias indicates reduction in concentrations from upstream of the curtain to downstream of the curtain.
Results
Curtain condition
Daily average h and curtain deployment depths illustrate curtain condition during 2015–2021 (). Daily average mixed layer depths are shown although hourly mixed layer depths were calculated and used for sample comparisons, as hourly mixed layer depths are difficult to illustrate at an annual scale. Epilimnetic stratification (and h) generally occurred at shallower depths in July through August, after which epilimnetic stratification weakened with mixing to deeper depths occurring more often as day length decreased and seasonal thermal loading abated. While there were daily and subdaily changes in h caused by thermal exchange at the air–water interface and wind patterns, seasonal h conditions were typically consistent between years (). Hourly h during the first week of June, August, and October 2018 demonstrate stratification is more stable in spring and midsummer, and less stable in the fall ().
Figure 7. Daily average mixed layer depths (h and h2, based on Wedderburn number [Wn] and secondary Wn upstream of the curtain, respectively), curtain depths, and grab sample collection dates during (A) 2015, (B) 2016, (C) 2017, (D) 2018, (E) 2019, (F) 2020, and (G) 2021. The curtain was installed on 26 Jun 2015. No grab samples were collected in 2021.
![Figure 7. Daily average mixed layer depths (h and h2, based on Wedderburn number [Wn] and secondary Wn upstream of the curtain, respectively), curtain depths, and grab sample collection dates during (A) 2015, (B) 2016, (C) 2017, (D) 2018, (E) 2019, (F) 2020, and (G) 2021. The curtain was installed on 26 Jun 2015. No grab samples were collected in 2021.](/cms/asset/c1588c2a-4925-462b-8587-3159d4c3de2e/ulrm_a_2259854_f0007_b.jpg)
Figure 8. Hourly and daily average mixed layer depths (h and h2, based on Wn and secondary Wn upstream of the curtain, respectively) and deployed curtain (light gray shading) during (A) the first week of Jun 2018, (B) the first week of Aug 2018, and (C) the first week of Oct 2018.

In 2015 from late July through September, the curtain depth often extended below h (C1 conditions; , left panel), except in mid August when mixing occurred to approximately 10 m (). In 2016 from late June through late August, the curtain extended below h (C1 conditions; ). The curtain was raised to 6.1 m in late August, which most of the time was just below h (C1 conditions). When the curtain was raised to 3.0 m in late September through October, the curtain was mostly above h (C3 conditions; , right panel; ). Even a curtain deployed to the design depth would have often resulted in C3 conditions in October, as waters were often mixed to deeper depths (). In 2017, 2018, and 2019, the curtain depth extended below h during periods in late July through early or mid August, and generally terminated within the mixed layer or close to h early in the summer and mid August to early September through fall (), resulting in C3 or C2 conditions (, right and middle panels). In 2020, the curtain was deployed to the design depth of 10.7 m all season except when it was raised to 4.6 m in late August through late September (). This resulted in C1 conditions until early September. In early September through October, C1, C2, and C3 conditions occurred as mixing periodically occurred to depths deeper than h. In 2021, the curtain was deployed to design depth all season, with C1 conditions occurring until mixing occurred to deeper depths (C2 and C3 conditions) in October ().
Curtain effectiveness
In total, 559 samples pairs (all analytes) were collected on 28 dates in 2015–2020 () and analyzed. Totals of 171, 118, 118, and 152 were total Chl-a, total cyanobacteria, total Microcystis spp., and total microcystin sample pairs, respectively. Differences in the number of sample pairs for each constituent occurred because total cyanobacteria and total Microcystis spp. were not analyzed in 2015 and some samples were lost during shipping. Of these, 100, 77, 77, and 88 total Chl-a, total cyanobacteria, total Microcystis spp., and total microcystin sample pairs, respectively, were collected at least 0.5 m above the depth of the deployed curtain or when the curtain was not deployed, and 17, 12, 12, and 16 total Chl-a, total cyanobacteria, total Microcystis spp., and total microcystin sample pairs, respectively, were collected either approximately at or just below the deployed curtain. Water temperature data to determine Wn values and curtain condition were unavailable for one sample collection date in October 2015. These total Chl-a sample pairs (n = 2) and total microcystin sample pairs (n = 2) were the only samples collected in October and were not included in the analysis or presented. Some of the integrated 0–8 m samples collected in August and September 2015 included depths both above and below h, and these total Chl-a sample pairs (n = 3) and total microcystin sample pairs (n = 3) were not included in the analysis or presented.
Total chlorophyll a
During C1 conditions, all sample pairs collected from a depth at least 0.5 m shallower than the curtain depth and from a depth ≤ h (C1a) demonstrated reduction in total Chl-a concentrations from upstream to downstream of the curtain, with an average reduction of 67.2 μg/L (n = 42, mean bias = −67.2 µg/L, average percent reduction of 87%; and ). Reduction in total Chl-a concentrations from upstream to downstream of the curtain was significant (P = 0.000 for both tests; ). During C1 conditions and for sample pairs collected from a depth at least 0.5 m shallower than the curtain depth and from a depth > h (C1b), all except one pair demonstrated reduction in total Chl-a concentrations from upstream to downstream of the curtain, but average reduction was less than for C1a samples. For C1b, average reduction was 9.7 μg/L (n = 15; average percent reduction of 40%; and ). Reduction in total Chl-a concentrations from upstream to downstream of the curtain was significant (P = 0.000 for both tests; ). During C2 conditions and for sample pairs collected from a depth at least 0.5 m shallower than the curtain depth (C2a), reduction in total Chl-a concentrations from upstream to downstream of the curtain was significant (P = 0.001 and P < 0.050 for sign and Wilcoxon signed-rank tests, respectively) and all sample pairs demonstrated reduction, with an average reduction of 30.7 μg/L (n = 9; average percent reduction of 52%; and ). During C3 conditions and for sample pairs collected from a depth at least 0.5 m shallower than the curtain depth (C3a), 1 of 3 sample pairs demonstrated reduction in total Chl-a concentrations from upstream to downstream of the curtain, with an average reduction of 9.7 μg/L (n = 3; average percent reduction of 5%; and ); sample size was too small for statistical tests. For sample pairs collected approximately at the bottom of the deployed curtain, reduction in total Chl-a concentrations from upstream to downstream of the curtain was significant (P = 0.013 and P < 0.050 for sign and Wilcoxon signed-rank tests, respectively) and all sample pairs demonstrated reduction; average reduction was 9.7 μg/L (n = 5; average percent reduction of 53%; ). For samples collected just below the bottom of the deployed curtain, reduction in total Chl-a concentrations from upstream to downstream of the curtain was not significant (P = 0.282 and P = 0.218 for sign and Wilcoxon signed-rank tests, respectively; ). When the curtain was not deployed, for all sample pairs and all curtain conditions combined (NDall, n = 31), neither test demonstrated significant reduction in total Chl-a concentrations (P = 0.557 and P = 0.268 for sign and Wilcoxon signed-rank tests, respectively) from upstream to downstream of the curtain ( and ).
Figure 9. (a) Total chlorophyll a concentrations (µg/L) for 42 sample pairs collected upstream and downstream of the curtain at different depths and periods during curtain condition and sample depth subcategory C1a (curtain deeper than the mixed layer depth and sample depth shallower than or at the mixed layer depth) and (b) corresponding sample depths by date. Vertical lines with icon at 4 m denote pooled mixed layer sample from 0 to 4 m.

Figure 10. (a) Total chlorophyll a concentrations (µg/L) for 15 sample pairs collected upstream and downstream of the curtain at different depths during curtain condition and sample depth subcategory C1b (curtain deployed deeper than the mixed layer depth and sample depth deeper than the mixed layer depth) and (b) corresponding sample depths by date.

Figure 11. (a) Total chlorophyll a concentrations (µg/L) for 9 sample pairs collected upstream and downstream of the curtain at different depths during curtain condition and sample depth subcategory C2a (curtain deployed at approximately the mixed layer depth and sample depth shallower than or at the mixed layer depth) and (b) corresponding sample depths by date. Vertical line with icon at 4 m denotes pooled mixed layer sample from 0 to 4 m.

Figure 12. (a) Total chlorophyll a concentrations (µg/L) for 3 sample pairs collected upstream and downstream of the curtain at different depths during curtain condition and sample depth subcategory C3a (curtain deployed to a depth shallower than the mixed layer depth and sample depth shallower than or at the mixed layer depth) and (b) corresponding sample depths by date.

Figure 13. (a) Total chlorophyll a concentrations (µg/L) for 31 sample pairs collected upstream and downstream of the curtain while the curtain was not deployed and all sample depths (NDall), and (b) corresponding sample depths by date.

Table 3. Sign and Wilcoxon signed-rank test results for each curtain condition and sample depth subcategory for total chlorophyll a (µg/L) sample pairs (n).
Additional constituents
The same general outcome observed for total Chl-a was observed for total cyanobacteria, total Microcystis spp., and total microcystin, with significant reduction in concentrations and smallest P values observed consistently from samples collected from within the mixed layer when the curtain was deployed during C1 conditions (C1a; ). Average percent reduction observed in these sample pairs was 90%, 78%, and 64% for total cyanobacteria gene concentrations, total Microcystis spp. gene concentrations, and total microcystin concentrations, respectively. As for total Chl-a, significant reductions in concentrations were also observed for total cyanobacteria and total microcystin C1b sample pairs across both tests, and for total cyanobacteria and total Microcystis spp. C2a sample pairs across both tests (). Limited data were available for C3 conditions (n = 3 or n = 2 for each constituent) and for sample pairs collected approximately at the depth of the deployed curtain (n = 4 for each constituent), hence statistical tests could not be performed. There were no significant differences in concentrations for sample pairs collected just below the deployed curtain (n = 8 or n = 12 for each constituent). There were also no significant differences in concentrations for when the curtain was not deployed, for all sample depths and all curtain conditions combined (NDall) except for NDall total microcystin samples (n = 25); reduction was significant for this set of sample pairs, although many of the total microcystin sample pair differences were small, with an average bias of −0.5 μg/L and percent change of −6% ().
Table 4. Sign and Wilcoxon signed-rank test results, mean bias, and mean percent reduction for each curtain condition and sample depth subcategory for total cyanobacteria (copies/mL), total Microcystis spp. (copies/mL), and total microcystin (µg/L) sample pairs (n).
Discussion
The curtain was hypothesized to be effective at reducing cyanobacteria concentrations and associated toxins downstream of the curtain during periods of stratification and curtain deployment to a depth below h (C1 condition). The curtain was predicted to be less effective when deployed to depths that did not extend below h (C2 or C3 conditions), and ineffective when the curtain was not deployed. Results supported these hypotheses.
Curtain condition and curtain effectiveness
Cyanobacterial blooms and associated toxins generally occur in Iron Gate Reservoir in July through October, coincident with seasonal and epilimnetic stratification; thus, the curtain was deployed during these months to reduce cyanobacteria and associated toxins in downstream waters. The curtain was often deployed below h (C1 conditions) during periods in July and August (). C1 conditions were expected to minimize the downstream movement of mixed layer waters, which contained higher concentrations of cyanobacteria. In fact, when C1 conditions occurred, total Chl-a, total cyanobacteria, total Microcystis spp., and total microcystin concentrations were significantly reduced downstream of the curtain in samples from depths ≤ h (C1a); reductions averaged 87, 90, 78, and 64%, respectively ( and and ). Total Chl-a, total cyanobacteria, and total Microcystis spp. concentrations were also reduced downstream of the curtain samples at depths between the curtain depth and h (C1b), but generally not to the extent of reduction that occurred in shallower C1a waters ( and and ). Leakage at or below the bottom of the deployed curtain was not typical; instead, reduction in total Chl-a concentrations was observed for sample pairs at approximately the depth of the curtain, and no significant differences were observed for sample pairs just below the depth of the curtain. By preventing the mixed layer waters from flowing toward the intake when the curtain was deployed to a sufficient depth, the curtain formed a barrier that retained upstream cyanobacteria in near-surface waters, much like a temperature control device for releasing deep waters, albeit potentially at a lower cost.
The curtain was raised to shallower depths throughout the late summer and fall during some years to address dissolved oxygen conditions in the Klamath River downstream of Iron Gate Dam (). However, data collected downstream of the dam demonstrated sufficient re-aeration through both turbine venting at the powerhouse (PacifiCorp Citation2011) and re-aeration in powerhouse releases below Iron Gate Dam (Watercourse Citation2020) to meet the HCP requirements (PacifiCorp Citation2012), such that raising the curtain ultimately was unnecessary with respect to dissolved oxygen. With installation of a similar water quality control curtain in other reservoirs to control dense cyanobacteria blooms, similar reduced dissolved oxygen conditions in deeper waters might be expected, especially during peak production.
Periodic mixing events become more common in late September and October in response to fall cooling of the reservoir and a decrease in density differentials allowing wind or other mixing events to more easily overcome vertical density differences. During shallower curtain deployments or mixing to deeper depths, C2 and C3 conditions often resulted () and, as hypothesized, the curtain still retained some cyanobacteria and toxins upstream of the curtain but became less effective than during C1 conditions (although fewer sample pairs were available for C2 and C3 conditions). For samples from depths shallower than the curtain depth during C2 conditions (C2a), total Chl-a, total cyanobacteria, and total Microcystis spp. concentrations were significantly reduced downstream of the curtain in comparison to upstream of the curtain, with reductions generally smaller in magnitude (and P values generally larger in magnitude) than for C1a sample pairs ( and and ). Limited data were available to test C3 conditions (n = 3 or n = 2 for each constituent), but most of these pairs did not demonstrate reduction from upstream of the curtain to downstream of the curtain ( and and ). Data at or just below the deployed curtain demonstrated that leakage of cyanobacteria or toxins under the curtain was not typical ( and ).
As expected, the curtain was not effective when not deployed (curtain depth = 1.5 m, ND). Significant reductions in total Chl-a, total cyanobacteria, and total Microcystis spp. concentrations did not occur during ND conditions. Total microcystin sample pairs demonstrated a significant reduction in concentrations from upstream to downstream of the curtain for NDall sample pairs, although differences were generally small ().
The curtain depth was not always > h when not deployed at the design depth of 10.7 m, although C1 conditions still occurred during shallower curtain deployments depending on epilimnetic stratification conditions (i.e., h) and between episodic mixing events in September and October. Mixing to 10.7 m (h ≥ 10.7 m) rarely occurred in July or August, while mixing to 10.7 m occurred occasionally in September and more frequently in October with the onset of fall cooling (). The design depth of 10.7 m may not be as effective in October unless stratification persists at or above this depth, such as occurred in late October 2018 and October 2019 ().
Ideally the curtain would be placed at the design depth prior to the cyanobacteria bloom period (e.g., May or Jun) and remain at that depth until cyanobacteria conditions abate in the reservoir. During the years evaluated in this discussion, low dissolved oxygen in deeper waters of the epilimnion at times led to a shallower deployment depth to ensure specific water quality requirements were met during periods coincident with increased cyanobacteria concentrations (i.e., Jul through Oct), which reduced curtain efficacy. Dissolved oxygen data collected in the Klamath River downstream of Iron Gate Dam demonstrated that re-aeration occurred (PacifiCorp Citation2011, Watercourse Citation2020). For water quality control curtain applications in other reservoirs, similar dissolved oxygen studies and/or turbine venting may be warranted.
Conclusions
Design, installation, and operation of a water quality curtain in Iron Gate Reservoir is a unique water quality control approach to segregate near-surface mixed layer waters from deeper waters by taking advantage of the tendency of buoyancy regulating cyanobacteria to occupy the euphotic zone, and thus to reduce entrainment of cyanobacteria and associated toxins into the intake for release to downstream Klamath River reaches. When stratification existed and the curtain was deployed to a depth > h, the curtain was most effective at reducing total Chl-a, total cyanobacteria, total Microcystis spp., and total microcystin concentrations in Iron Gate Reservoir waters downstream of the curtain and hence in the Klamath River downstream of Iron Gate Dam. During these conditions, concentrations of total chlorophyll a, total cyanobacteria, total Microcystis spp., and total microcystin in waters downstream of the curtain were significantly reduced by an average of 87, 90, 78, and 64%, respectively. When the curtain did not extend below h, the curtain did not consistently reduce concentrations in waters downstream of the curtain and reductions were generally smaller in magnitude. Therefore, when the curtain is used at design depth (10.7 m) or at least deployed to a depth > h, reduction in cyanobacteria downstream of the curtain and thus downstream of the dam can be expected.
The key to achieving consistently effective curtain performance and improved downstream water quality conditions is to ensure that curtain design depth is adequate to allow continuous deployment below h. This study indicates that an intake barrier curtain is an effective method to leverage the buoyancy control of cyanobacteria to retain this material in Iron Gate Reservoir. In other stratified eutrophic systems with the appropriate outlet works, an intake barrier curtain may provide a relatively inexpensive way to control downstream release of cyanobacteria and related toxins.
Acknowledgments
The authors thank Klamath Hydroelectric Settlement Agreement Interim Measure Implementation Committee for providing comments and guidance throughout this study. Thanks are extended to E&S Environmental Chemistry for sample collection and PacifiCorp operational staff on the Lower Klamath Hydroelectric Project for operating the curtain through the years. We also thank 3 anonymous reviewers whose comments helped improve and clarify this article. PacifiCorp provided funding for this work.
Disclosure statement
The authors report there are no competing interests to declare.
References
- Asaeda T, Priyantha DGN, Saitoh S, Gotoh K. 1996. A new technique for controlling algal blooms in the withdrawal zone of reservoirs using vertical curtains. Ecol Eng. 7(2):95–104. doi: 10.1016/0925-8574(96)00002-X.
- Bownik A. 2016. Harmful algae: effects of cyanobacterial cyclic peptides on aquatic invertebrates – a short review. Toxicon 124:26–35. doi: 10.1016/j.toxicon.2016.10.017.
- Carmichael WW, Boyer GL. 2016. Health impacts from cyanobacteria harmful algae blooms: implications for the North American Great Lakes. Harmful Algae. 54:194–212. doi: 10.1016/j.hal.2016.02.002.
- Chorus I, Bartram J, editors. 1999. Toxic cyanobacteria in water – a guide to their public health consequences, monitoring and management. London (UK): World Health Organization. E & FN Spon.
- Deas ML, Miao E. 2010. Exploratory velocity measurements in Iron Gate Reservoir with an acoustic doppler current profiler. Prepared for PacifiCorp. July 12; [cited 24 Apr 2023]. Available from https://www.pacificorp.com/energy/hydro/klamath-river/khsa-implementation.html.
- Ferrão-Filho AS, Kozlowsky-Suzuki B. 2011. Cyanotoxins: bioaccumulation and effects on aquatic animals. Mar Drugs. 9(12):2729–2772. doi: 10.3390/md9122729.
- Fischer HB, List EJ, Hoh RCY, Imberger J, Brooks NH. 1979. Mixing in inland and coastal waters. New York (NY): Academic Press.
- Graham JL, Loftin KA, Ziegler AC, Meyer MT. 2008. Chapter A7.5, Cyanobacteria in lakes and reservoirs – toxin and taste-and-odor sampling guidelines (ver. 1.0). In: U.S. Geological Survey techniques of water-resources investigations, book 9. Reston (VA): U.S. Geological Survey. p. 1-65. doi: 10.3133/twri09A7.5.
- Griese M, Lange C, Soppa J. 2011. Ploidy in cyanobacteria. FEMS Microbiol Lett. 323(2):124–131. doi: 10.1111/j.1574-6968.2011.02368.x.
- Harke MJ, Steffen MM, Gobler CJ, Otten TG, Wilhelm SW, Wood SA, Paerl HW. 2016. A review of the global ecology, genomics, and biogeography of the toxic cyanobacterium, Microcystis spp. Harmful Algae. 54:4–20. doi: 10.1016/j.hal.2015.12.007.
- Helsel, Dennis R., Hirch, Robert M. 2002. Chapter A3, statistical methods in water resources, In: Techniques of water-resources investigations of the United States geological survey, book 4, hydrologic analysis and interpretation. United States Geological Survey. September 2002, p. 1–510. [cited 1 June 2019]. Available from http://water.usgs.gov/pubs/twri/twri4a3/
- Horne AJ, Goldman CR. 1994. Limnology. New York (NY): McGraw Hill.
- Huisman J, Sharples J, Stroom JM, Visser PM, Kardinaal WEA, Verspagen JMH, Sommeijer B. 2004. Changes in turbulent mixing shift competition for light between phytoplankton species. Ecology 85(11):2960–2970. doi: 10.1890/03-0763.
- Imberger J. 1985. Thermal characteristics of standing waters: an illustration of dynamical processes. Hydrobiologia 125(1):7–29. doi: 10.1007/BF00045923.
- Imberger J, Hamblin PF. 1982. Dynamics of lakes, reservoirs, and cooling ponds. Annu Rev Fluid Mech. 14(1):153–187. doi: 10.1146/annurev.fl.14.010182.001101.
- Jacoby JM, Kann J. 2007. The occurrence and response to toxic cyanobacteria in the Pacific Northwest, North America. Lake and Reserv Manage. 23(2):123–143. doi: 10.1080/07438140709353916.
- Joo G, Jang M, Park S, Jung J, Roh J, Jeong K. 2003. The application of an algal fence for the reduction of algal intake into the water intake facility. Korean J Limnol. 36(4):467–472.
- Kalff J. 2002. Limnology. Upper Saddle River (NJ): Prentice-Hall, Inc.
- Kann J, Corum S. 2006. Summary of 2005 toxic Microcystis aeruginosa trends in copco and iron gate reservoirs on the Klamath River CA. Technical memorandum prepared for the Karuk Tribe Dept. of Natural Resources, Orleans, CA, March; [cited 24 Apr 2023]. Available from https://www.waterboards.ca.gov/water_issues/programs/tmdl/records/region_1/2009/ref2952.pdf.
- Kann J, Corum S. 2009. Toxigenic Microcystis aeruginosa bloom dynamics and cell density/chlorophyll a relationships with microcystin toxin in the Klamath River, 2005–2008. Technical memorandum prepared for the Karuk Tribe Dept. of Natural Resources, Orleans, CA, May; [cited 24 Apr 2023]. Available from http://www.karuk.us/images/docs/wqdocuments/2008_Karuk_Toxic_Cyanobacteria_summary.pdf.
- Lyra C, Suomalainen S, Gugger M, Vezie C, Sundman P, Paulin L, Sivonen K. 2001. Molecular characterization of planktic cyanobacteria of Anabaena, Aphanizomenon, Microcystis and Planktothrix genera. Int J Syst Evol Microbiol. 51(Pt 2):513–526. doi: 10.1099/00207713-51-2-513.
- McLellan NL, Manderville RA. 2017. Toxic mechanisms of microcystins in mammals. Toxicol Res (Camb). 6(4):391–405. doi: 10.1039/c7tx00043j.
- Miao E, Deas ML. 2014. Assessment of an intake barrier for water quality control at Iron Gate Reservoir – 2013 study results. Prepared for PacifiCorp, April; [cited 2023 Apr 24]. Available from https://www.pacificorp.com/energy/hydro/klamath-river/khsa-implementation.html.
- Moisander PH, Lehman PH, Ochiai M, Corum S. 2009. Diversity of Microcystis aeruginosa in the Klamath River and San Francisco Bay delta, California, USA. Aquat Microb Ecol. 57(1):19–31. doi: 10.3354/ame01320.
- Oliver AA, Dahlgren RA, Deas ML. 2014. The upside-down river: reservoirs, algal blooms, and tributaries affect temporal and spatial patterns in nitrogen and phosphorus in the Klamath River, USA. J Hydrol. 519(A):164–176. doi: 10.1016/j.jhydrol.2014.06.025.
- Otten TG. 2017. Application of genetic tools for improved cyanobacterial bloom monitoring in the Klamath river system: implications for public health monitoring. Bend Genetics, LLC. April; [cited 24 Apr 2023]. Available from https://www.pacificorp.com/energy/hydro/klamath-river/khsa-implementation.html.
- Otten TG, Crosswell JR, Mackey S, Dreher TW. 2015. Application of molecular tools for microbial source tracking and public health risk assessment of a Microcystis bloom traversing 300 km of the Klamath River. Harmful Algae. 46:71–81. doi: 10.1016/j.hal.2015.05.007.
- PacifiCorp. 2011. Results of 2010 turbine venting tests to improve dissolved oxygen below Iron Gate Dam. September; [cited 24 Apr 2023]. Available from https://www.pacificorp.com/content/dam/pcorp/documents/en/pacificorp/energy/hydro/klamath-river/water-quality-reports-and-data/reports/2010TurbineVentingTestResultsF.pdf.
- PacifiCorp. 2012. PacifiCorp Klamath Hydroelectric Project: Interim Operations Habitat Conservation Plan for Coho Salmon PacifiCorp. February.
- PacifiCorp. 2021. Intake barrier curtain summary report. May; [cited 24 Apr 2023]. Available from https://www.pacificorp.com/energy/hydro/klamath-river/khsa-implementation.html.
- Park C, Park M, Kim K, Kim N, Kim Y, Gwon E, Kim B, Lim B, Hwang S. 2017. A physical pre-treatment method (vertical weir curtain) for mitigating cyanobacteria and some of their metabolites in a drinking water reservoir. Water 9(10):775. doi: 10.3390/w9100775.
- Reynolds CS. 1987. Cyanobacterial water-blooms. In: Callow P, editor. Advances in botanical research. Vol. 13. London (UK): Academic Press. p. 67–143.
- Reynolds CS, Oliver RL, Walsby AE. 1987. Cyanobacterial dominance: the role of buoyancy regulation in dynamic lake environments. N Z J Mar Freshwater Res. 21(3):379–390. doi: 10.1080/00288330.1987.9516234.
- Reynolds CS, Walsby AE. 1975. Water-blooms. Biol Rev. 50(4):437–481. doi: 10.1111/j.1469-185X.1975.tb01060.x.
- Reynolds CS, Wiseman SW, Godfrey BM, Butterwick C. 1983. Some effects of artificial mixing on the dynamics of phytoplankton populations in large limnetic enclosures. J Plankton Res. 5(2):203–234. doi: 10.1093/plankt/5.2.203.
- Sabart M, Pobel D, Latour D, Robin J, Salencon M, Humbert J. 2009. Spatiotemporal changes in the genetic diversity in French bloom-forming populations of the toxic cyanobacterium, Microcystis aeruginosa. Environ Microbiol Rep. 1(4):263–272. doi: 10.1111/j.1758-2229.2009.00042.x.
- Sivonen K. 2009. Cyanobacterial toxins. In: Schaechter M, editor. Encyclopedia of microbiology. 3rd ed. Amsterdam: Elsevier Scientific Publ. Co. p. 290–307.
- Spigel RH, Imberger J. 1987. Mixing processes relevant to phytoplankton dynamics in lakes. N Z J Mar Freshwater Res. 21(3):361–377. doi: 10.1080/00288330.1987.9516233.
- [State Water Board] State Water Resources Control Board. 2016. Appendix to the CCHAB preliminary changes to the Statewide Voluntary Guidance on CyanoHABs in recreational waters. January.
- Tillett D, Parker DL, Neilan BA. 2001. Detection of toxigenity by a probe for the microcystin synthetase A gene (mcyA) of the cyanobacterial genus Microcystis: comparison of toxicities with 16S rRNA and phycocyanin operon (phycocyanin intergenic spacer) phylogenies. Appl Environ Microbiol. 67(6):2810–2818. doi: 10.1128/AEM.67.6.2810-2818.2001.
- Van der Merwe D. 2014. Chapter 31 – Freshwater cyanotoxins. In: Gupta RC, editor. Biomarkers in toxicology. Boston (MA): Academic Press. p. 539–548. doi: 10.1016/B978-0-12-404630-6.00031-2.
- Visser PM, Ibelings BW, Bormans M, Huisman J. 2016. Artificial mixing to control cyanobacterial blooms: a review. Aquat Ecol. 50(3):423–441. doi: 10.1007/s10452-015-9537-0.
- Walsby AE. 1987. Mechanisms of buoyancy regulation by planktonic cyanobacteria with gas vesicles. In: Fay P, Baalen CV, editors. The Cyanobacteria. Amsterdam (The Netherlands): Elsevier. p. 377–392.
- [Watercourse] Watercourse Engineering Inc. 2013. 2012 assessment of an intake cover for water quality control at Iron Gate Reservoir. Prepared for PacifiCorp Energy, July; [cited 24 Apr 2023]. Available from https://www.pacificorp.com/energy/hydro/klamath-river/khsa-implementation.html.
- [Watercourse] Watercourse Engineering Inc. 2015. 2014 localized treatment of Long Gulch Cove in Iron Gate Reservoir using hydrogen peroxide based algaecide. Prepared for PacifiCorp Energy. April; [cited 24 Apr 2023]. Available from https://www.pacificorp.com/energy/hydro/klamath-river/khsa-implementation.html.
- [Watercourse] Watercourse Engineering Inc. 2016. Water quality effects of an intake barrier curtain to reduce algae concentrations downstream of Iron Gate Reservoir. Prepared for PacifiCorp. July; [cited 24 Apr 2023]. Available from https://www.pacificorp.com/energy/hydro/klamath-river/khsa-implementation.html.
- [Watercourse] Watercourse Engineering Inc. 2017. 2016 evaluation of intake barrier curtain in Iron Gate Reservoir to improve water quality in the Klamath River. Prepared for PacifiCorp. October; [cited 24 Apr 2023]. Available from https://www.pacificorp.com/energy/hydro/klamath-river/khsa-implementation.html.
- [Watercourse] Watercourse Engineering, Inc. 2020. Summary of dissolved oxygen monitoring in the Klamath River downstream of Iron Gate Dam in 2019. Prepared for PacifiCorp. November; [cited 24 Apr 2023]. Available from https://www.pacificorp.com/energy/hydro/klamath-river/khsa-implementation.html.
- [WHO] World Health Organization. 2020. Cyanobacterial toxins: microcystins – background document for development of WHO guidelines for drinking-water quality and guidelines for safe and recreational water environments. Geneva (Switzerland): World Health Organization. WHO/HEP/ECH/WSH/2020.6. License: CC BY-NCSA 3.0 IGO; [cited 24 Apr 2023]. Available from https://apps.who.int/iris/handle/10665/338066.