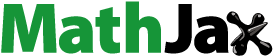
Abstract
The global use of “asbestos” in various commercial products has led to a wide range and pervasive legacy of disease. One such use of chrysotile asbestos was brake pads and was utilized commonly in automobiles and heavy vehicles. The result of incorporation of chrysotile into brake pads is associated with the exposure of mechanics fitting and servicing vehicles to liberated chrysotile fibers. Despite the proven exposure, the relative risk of malignant mesothelioma (MM) in this occupational population is broadly seen as low. The toxicity of particulates, including fibers such as chrysotile, is driven by a combination of dose and physicochemical properties. As such, it is plausible that chrysotile released from brake pads may have undergone modification, thereby altering the pathogenicity profile. The impact of high sheer stress causing shortening of long fibers, heat modification, binding of resin matrix to the fiber surface on the relative toxicity of brake debris with regards to MM is considered. It is apparent that released chrysotile can undergo significant modification, reducing the long fiber dose although not all modifications may lead to reduced toxicity.
Introduction
During the early twentieth century, “asbestos” was widely regarded as a wonder material due to the array of useful properties it possesses, such as fire and heat resistance (the word “asbestos” is derived from the Greek “ἄσβεστος” or “unquenchable”, “inextinguishable”). In the case of chrysotile asbestos, the flowing fibrils also made it possible to weave the mineral fibers into fireproof cloth. “Asbestos” was also abundant and mined on a large scale in many countries, most notably Canada and Russia but also many other countries such as South Africa, China, and Italy.
Rather than being a single entity, “asbestos” is a collective term for a group of naturally occurring silicate fibers and are broadly classified into serpentine or amphibole. The serpentine group consists only of chrysotile asbestos, a flexible and flowing fiber of thin chrysotile fibrils that can be woven while the amphibole group consists of five different forms of “asbestos”, namely crocidolite, amosite, tremolite, anthophyllite, and actinolite. Crocidolite and amosite asbestos were used commercially to some degree including significant usage in naval and merchant shipping as insulation materials in the form of asbestos filled mattresses, lagging and prior to 1963, sprayed asbestos (Harries Citation1968). However, of the different forms of “asbestos”, chrysotile was the most extensively used worldwide (Virta Citation2006). The availability and useful properties of chrysotile, amosite, and crocidolite asbestos fibers promoted their use in many different environments and products. Their lightweight, fireproofing, and insulating capacity made them indispensable in the technological revolution at the beginning of the twentieth century. “Asbestos” fibers such as chrysotile found uses in brake and clutch linings, insulation for fuel tanks for the growing aviation industry, cement boards and roofing tiles in the building industry and even as “artificial snow” sold to the public.
With widespread use and as such, exposure, the more perilous side of “asbestos” became more apparent. In the UK, the first documented impact of “asbestos” on respiratory health was in 1898 as part of the HM Chief Inspector of Factories and Workshops Annual Report (Deane Citation1899). Over the course of the twentieth century, studies further strengthened the link between “asbestos” exposure and respiratory diseases such as fibrosis and lung cancer. However, it was not until the seminal study by Wagner et al. (Citation1960) that malignant mesothelioma (MM), a rare and universally fatal cancer of the serosal tissues, and its association to the crocidolite asbestos of the “Cape Blue Asbestos” was fully recognized. In December 1976, the International Agency for Research on Cancer (IARC) evaluated asbestos and classified “asbestos” in all forms as a Group 1 carcinogen (carcinogenic to humans) (IARC Citation1977).
Epidemiological studies have incontrovertibly demonstrated “asbestos” as being the causal factor for morbidity and mortality associated with a wide range of occupational as well as non-occupational exposure scenarios involving different forms of “asbestos”. Nevertheless, the association between working with chrysotile-containing friction products such as brake pads and excess risk of MM has proven to be more controversial. Various peer-reviewed studies demonstrate no excess risk of MM in mechanics who have worked with chrysotile-containing brake pads despite exposure studies showing varying degrees of exposure to chrysotile fibers. However, such conclusions are hotly debated with questions raised over approaches to assessing excess risk in cohorts as well as exposure. However, few studies have explicitly considered the possible modification of chrysotile fibers resulting from the encasement in resin and subsequent liberation during grinding of brake pads. As the toxicity of particles and fibers is dictated purely by physicochemical characteristics and dose, it seems clear that any modification of the chrysotile could have implications for relative toxicity. Changes to the size, shape, surface properties, crystal structure, etc. could alter the nature of biological interactions making the released chrysotile more or less hazardous as compared with pristine fibers. The purpose of this review is to examine whether or not there is a toxicological explanation as to why chrysotile liberated from brake pad surfaces may show a modified toxicity profile.
Use of chrysotile in friction productions
With the industrial revolution, there has been a continuous need for resilient and effective braking systems for factory machinery and with their advent, automobiles. An example of this need is the very first steam-driven automobile developed by Nicholas Cugnot in the 1700s. Sadly, an accident occurred when at the heady speed of 10 km/h, the inventor realized he had no way of stopping the invention (bar a wall) (Maluf et al. Citation2006). Since this early foray into motorized transport, friction products have continued to develop with automotive, aerospace, and industrial applications. Typically, friction products consist of five main elements: binders, fibers, fillers, frictional additives, and abrasives (Grigoratos and Martini Citation2015). The binders serve to provide structural integrity by holding the components together with phenolic resins commonly used as polymeric binders and account for 20–40% of the brake liner. Fibers are used to provide mechanical strength; reinforcing the brake pad (Ganguly and George Citation2008) and account for 6–35% of the brake mass although in certain formulations, this can be much higher (Weir and Meraz Citation2001; Madl et al. Citation2008). These fibers are typically classified as metallic, mineral, ceramic or organic, and in modern friction materials, these often consist of glass fiber, copper, steel, Kevlar, and potassium titanate (Grigoratos and Martini Citation2015). For many years, chrysotile asbestos fibers were used as a reinforcing material due to their tensile strength and capacity to withstand heat; a technological improvement over those previously used such as camel hair and cotton-based textiles. Fillers such as silicates, ground slag, and metal oxides are also included to reduce production cost as well as improve the properties of the pads while carbonaceous materials such as graphite and carbon black are commonly used as lubricants to modify the wear characteristics of the brake pad. Finally, abrasives such as aluminum oxide and iron oxide are also included to increase friction and limit the build-up of transfer films which could reduce the effectiveness of the brake (Grigoratos and Martini Citation2015). The specific composition of brake pads varies between types of liner and manufacturer and over time have changed with technological advancement.
The use of “asbestos” in brake pads has been phased out in most countries, and in the UK, the use of “asbestos” in brake pads was banned from 1999, with the exception that pre-1973 vehicles could continue to be fitted with “asbestos”-containing brake shoes until 2004 (HSE Citation2009). In the USA, brake pads, clutch facings, and other friction materials containing “asbestos” are still permitted (EPA Citation2018) although no longer manufactured and as such, in very limited circulation.
Release and characteristics of chrysotile-containing brake debris
In the fitting of friction pads during the servicing of vehicles, the leading edge of the pad was often beveled to produce a smoother interaction between the brake and the opposing metal surface (Weir and Meraz Citation2001). Also, replacement of friction pads on heavy-duty vehicles and industrial equipment often required the pads to be drilled to allow attachment to the shoe or backing plate. This machining of pads to permit their optimal attachment and operation naturally results in the release of fine debris made up of the components of the friction pads, including chrysotile asbestos. The physicochemical properties of the released debris are crucial in determining the ability of the material to be inhaled and deposit in the lung thereby providing a tissue dose. Also, physicochemical properties dictate the interaction with the body such as the rate of clearance, reactivity, and ability to translocate. Particle toxicity is not solely determined by bulk composition but instead a more nuanced collective of shape, size, surface properties, composition, and solubility.
There have been a limited number of studies looking at the morphological characteristics of debris released from asbestos-containing brake pads during machining.
An early study looked at the percentage of free fibers released from brake pads and found that less than 1% was released (relative to the 50% in the lining). Where these were observed, they were in the form of fiber bundles and fibrils with and without attached matrix particles (Lynch Citation1968).
The presence of free fibers was also observed in two later studies by Rohl, the first looking at chrysotile content in a range of 10 samples gathered within automobile repair shops in the US and the second within 39 samples from the US, four European countries and Australia. In the first study, the brake debris was in the form a heterogenous mix of particulate matter, free chrysotile fibers and fibers associated within resin. Chrysotile fiber bundles and fibrils were noted in all 10 samples although some of the fibers were observed without characteristic chrysotile morphology indicating partial or complete recrystallization (Rohl et al. Citation1976). The vast majority of the fibers were <5 µm in length which was in line with previous analysis by Hatch (Citation1970) which showed 94% of fibers were <5 µm.
In the second study, free chrysotile fiber bundles and fibrils were found in all 39 samples (Rohl et al. Citation1977). While there was little evidence of significant alteration, the vast majority (∼80%) were less than 0.4 µm in length although the percentage of regulated fibers was noted detailed.
More recently, a study by Weir and Meraz (Citation2001) used a combination of grinding and drilling of brake pads composed of 50% ± 10% chrysotile and a phenolic or cresylic resin base with additional friction modifiers. The liberated particulates were analyzed by scanning electron microscopy (SEM) and transmission electron microscopy (TEM) using NIOSH 239 procedures. Similar to the earlier studies described above, the authors found that the debris collected was composed of both particles and fibers with the majority of particulates being resin matrix. In addition, the majority of the fibrous materials was found to be bound to resin matrix and were compositionally and morphologically consistent with chrysotile asbestos.
While the study by Weir and Meraz provided insight into the types of particles found within the debris, they did not seek to isolate and characterize the more relevant respirable fraction of the debris or crucially, the number of classifiable fibers present (based on the WHO definition, WHO Citation1997). A later study by Atkinson et al. (Citation2004) evaluated the size and type of free particulates collected from chrysotile-containing brake components using TEM. However, whereas Weir and Meraz simulated grinding and drilling of the brake pads to generate particulates, Atkinson rinsed the unused brake pad to recover any loose surface debris. Therefore, the exposure scenario most associated with this form of sample collection would be the general handling of the brake pads rather than solely machining, the latter being more likely to result in aerosolization of particles with a possible machining associated alteration (e.g. heating and fragmentation). Analysis of the surface particulates showed appreciable numbers of fibrous structures ranging from fibrils and bundles to clusters with and without matrix attachments. The percentage of chrysotile particulates (fragments) ranged from 44.7% to 76.1% depending on test sample with a mean length ranging from 2.42 μm to 3.73 μm and width of 0.03 μm. It is worth noting that the primary fiber counting rules for most current methods state that a countable fiber should be longer than 5 μm, narrower than 3 μm, and have an aspect ratio greater than 3:1 (WHO Citation1997; HSE Citation2005; NOHSC Citation2005; Baron Citation2017). Therefore, based on this convention for assessing fiber exposure in the workplace, any material shorter than 5 μm or wider than 3 μm would not be counted as a fiber which, as discussed later, is an important component of fiber associated toxicity. The numbers of fibrils within the Atkinson study which met the counting criteria for a respirable fiber varied dependent on the four samples tested with values of 4.8%, 6.7%, 10.3%, and 22.5%. Chrysotile bundles were found to be around twice the mean length of fibrils, and overall, 23.5–44.2% of the bundles measured met the length and width criteria for a countable fiber. Also, the authors outlined size cutoffs for respirability of 10 μm for non-fibrous structures and diameter equal to or less than 3.5 μm for long fibrous particulates (fibrils and bundles). Based on such criteria, they suggested that 100% of the fibrils would be respirable with a slightly lower proportion of respirable bundles (88.5–98.5%). The least respirable material was fragments of the matrix with fibers attached (66.7–82.6%). It is important to consider however that such estimation of respirability was based on assumptions rather than empirical evidence and the selected cutoffs are rather generous when we consider recognized conventions of respirability. In terms of non-fibrous structures, particle size having 50% penetration for the thoracic and respirable fractions are 10 μm and 4.0 μm, respectively ((CEN) Citation1993; (ACGIH) Citation2005; Brown et al. Citation2013), therefore assuming respirability (i.e. penetration beyond the ciliated airways) based on a physical size of 10 μm is a significant overestimation. Furthermore, in relation to airborne particles, a study by Brown et al. (Citation2013) modeled particle deposition and predicted less than 20% penetration of 10 μm particles into the thorax. In relation to fibers, the mostly widely used convention and also that of World Health Organization (WHO Citation1997) is that a respirable fiber has a length greater than 5 μm, width less than 3 μm and an aspect ratio of greater than 3:1 (see Poland et al. Citation2018 for a wider discussion of evolution of fiber size limits). The limits set by Atkinson et al. sit out with these limits.
Overall, the characteristics of liberated particles from asbestos-containing brake pads show that chrysotile is indeed released and tends to be in the form of shorter fibers (i.e. <5 μm) with resin matrix attachments. There is evidence of heat modification including recrystallization but such modification appears to affect a minority of chrysotile structures. While the majority of particulates released are non-fibrous resin matrix, chrysotile that meets the standard definition of a fiber are also observed.
There is no doubt that “asbestos” poses a significant risk to the respiratory health of exposed individuals. The legacy of suffering that the widespread use of chrysotile asbestos has brought is irrefutable and certainly not disputed within this article. When considering chrysotile use in friction products, the literature provides evidence that:
Chrysotile can be released during brake maintenance activities (e.g. grinding) leading to possible worker exposure;
Exposure to chrysotile can result in a range of diseases including asbestosis, lung cancer and mesothelioma (IARC Citation2012) therefore presenting a significant hazard;
Levels of exposure can exceed occupational exposure limits (see later) yet epidemiolocal analysis have shown no excess risk.
Such findings seem counter intuitive and the purpose of this review is to consider this relationship between chrysotile release from friction products and mesothelioma. The intention is not to perform a repeat of previous analysis to determine relative risk of MM in mechanics as recent and excellent analysis exists (Garabrant et al. Citation2016a). Instead the purpose is to explore if physicochemical modification of chrysotile liberated from friction products provides a toxicological basis for altered toxicity of chrysotile. However, first, it is important to summarize the evidence surrounding asbestos-associated disease in exposed mechanics and the nature of their exposures.
Occupational exposure to chrysotile fibers in mechanics
The hazard profile of a substance is an important modifier when considering potential risks but when we also consider that high exposure to even low toxicity substances can cause disease (Cherrie et al. Citation2013), no exposure to even the most toxic of substances means an absence of risk of disease. Therefore, exposure is the key variable when defining risk of disease resulting from toxicants with toxicity (hazard) being an important, but secondary variable. This concept sits at the heart of toxicology as told by the father of toxicology, Paracelsus (Philippus Aureolus Theophrastus Bombastus von Hohenheim) (1493–1541) whom is said to have stated:
All things are poison and nothing is without poison; only the dose makes a thing not a poison. (Eikermann and Houle Citation2016)
Chrysotile is classified as a Group 1 carcinogen (IARC Citation2012) with numerous studies in various exposed populations showing relationships between chrysotile exposure and diseases such as asbestosis, lung cancer and MM (Hein et al. Citation2007; Berman and Crump Citation2008a; Loomis et al. Citation2010; Pierce et al. Citation2016). As such, when considering the relationship between disease and chrysotile use in friction products the first question to ask is “do chrysotile containing brake pads release asbestos fibers?”. It is not the purpose of this review to summarize all published analysis of chrysotile exposure resulting from machining brake pads (see Paustenbach et al. Citation2003, Citation2004; Kelsh et al. Citation2007 for a more comprehensive review). Instead, we seek to summarize a few key studies that highlight that chrysotile asbestos can be released during the grinding of chrysotile containing brake pads and exposures with levels varying greatly, including both above and below prescribed exposure limits. It is important to differentiate between those working with friction products (primarily mechanics) and those manufacturing them. The latter group would be exposed to pure chrysotile asbestos during the coating and mixing of the fibers with resin (Berry and Newhouse Citation1983) leading to the possibility of repeated high levels of exposure. Overall, the studies available for exposures in brake mechanics can be grouped into four types:
Historical exposure assessments (e.g. pre-1980s);
Modern retrospective analysis of historical exposure assessments;
Exposure characterization during reconstruction of brake maintenance activities using asbestos-containing brake pads;
Modern workplace analysis of mechanic shops currently working with chrysotile-containing brake pads (not UK or USA).
These types of studies are all present in the literature and have their respective benefits and draw backs. Historical assessments often suffer from relatively poor characterization of exposure and especially in relation to the physicochemical characteristics of particulates. Modern retrospective analysis make use of said limited historical data and therefore, are somewhat limited by the original (poor) characterization. Exposure characterization during a recreation is not necessarily the same as the event, no matter how much it seeks to be; not least given the variability in work places, etc. and therefore has its own inherent limitations. Perhaps the most reliable would be modern workplace analysis of premises currently working with chrysotile-containing brake pads such as seen in Columbia and Iran. However, even these are associated with their own issues such as possible changes in work practices, use of more modern tools, etc.
Considering recent workplace analysis first, a study by Cely-Garcia et al. (Citation2012) monitored the personal exposures of workers conducting brake repair and maintenance within brake repair shops (BRSs) in Bogotá, Columbia. The authors used a combination of personal and area samples to determine release/exposure and in particular, compliance with occupational exposure limits for asbestos. The study used a combination of the NIOSH Method 7400 which employs phase contrast microscopy (PCM) to detect fibers and NIOSH Method 7402 which uses TEM. While the use of TEM does allow the identification of fiber type owing to diffraction properties and energy-dispersive X-ray spectroscopy, counting rules limit counts to those particulates with diameter greater than 0.25 μm that meet the definition of a fiber (aspect ratio 3:1, longer than 5 μm) (NIOSH Citation1994). The following of such counting rules may underestimate exposure by discounting those fibers above the crucial 5 μm in length, yet are less than 0.25 μm in diameter. Fibers below 0.25 μm in diameter are fully respirable and therefore can present a tissue dose. However, the authors presented both the TEM counts and the phase contrast microscopy equivalent (PCME), i.e. following diameter conventions. The authors found that in 11 personal 8-h time-weighted average (TWA) measurements across three BRS, eight of the 11 samples when analyzed by TEM had optically equivalent fiber levels above 0.1 f cm−3. The difference in method is highlighted by the fact that PCM analysis of the same samples showed no such breaches of the 0.1 f cm−3 limit although five of the 11 samples were not counted due to particulate overloading of the filter (Cely-Garcia et al. Citation2012). This disparity was even more apparent when looking at concentrations measured during short term activities using both NIOSH methods 7400 and 7402. Over a 31-min sampling period in which a worker cut and ground chrysotile – containing brake pads, unriveted old brake linings and ground used brake pads from a different set of used brake pads, fiber concentration by TEM was 6.088 f cm−3 while PCME was 0.092 f cm−3. A later study, by the same authors in two transmission repair shops, again in Colombia found that on at least one of the 3–5 days sampling was conducted, all three riveters monitored showed exposures in exceedance of the OSHA PEL and Colombian permissible limit value. Furthermore, in relation to short term (30 min) exposures 4.3% exceeded the US OSHA exposure limit of 1 f cm−3 (Salazar et al. Citation2015). These results indicate that while exposures are typically below exposure limits, they were at times breached even during such a short sampling period indicating possible unsafe work practices.
A further small study, again by the same author looked at “asbestos” exposure in Columbian BRSs, this time considering heavy vehicles in two BRS, across 10 workers. They found 8-h TWA personal exposures ranged from 0.003 to 0.157 f/cm–3. Interestingly, they also found that three of the examined workers upon chest X-ray, showed evidence of pleural plaques (PP) which, while not pre-malignant or an independent risk factor for MM (Maxim et al. Citation2015), PP can indicate previous asbestos exposure (Cely-Garcia et al. Citation2015). The authors of both studies noted the small sample size of each study as an area of weakness and also that the level of chrysotile-containing brake manipulation in high income countries was not routine as noted in Bogotá. However, the studies do show exposure to chrysotile fibers through the grinding and riveting of asbestos containing brake pads as well as dry brushing of associated dust can occur and at levels that can exceed prescribed limits.
Considering reevaluations of historical data, a 2007 study by Finley et al. (Citation2007) reviewed “asbestos” exposures in brake workers between 1950 and 2000 to determine cumulative “asbestos” exposures in f/cm3 based on job tenure (years) and 8-h TWA airborne fiber concentrations (f/cm3). The data used came from US studies using PCM following the NIOSH methodology to determine fiber exposures in personal sampling. The study is interesting as the wide date range allows the consideration of the impact of dust-controlled measures as 42 of the samples collected in the 1970s was from BRS not employing dust control measures while 42 collected in the 1980s did. The cumulative exposure was compared to a theoretical lifetime cumulative fiber dose of 4.5 f/cm3/year which was based on 45 years exposure at the OSHA Permissible Exposure Limit (PEL) of 0.1 f/cm3.
The analysis showed that mechanics in the 1970s undertaking high levels of brake repair (minimum eight jobs per week) received a greater chrysotile exposure than those with low levels of brake repair (maximum four jobs per week). These exposure levels were at least 10 times higher than levels in the 1980s after dust control measures were introduced (2.63–2.79 f/cm3 year in the 1970s as compared to 0.06–0.09 f/cm3 year in 1980s). Yet even at the highest modeled levels, brake repair workers experienced a cumulative lifetime exposure of below 3 f/cm3 year, which is significantly lower than the cumulative lifetime exposure at the OSHA PEL. It should also be noted that the theoretical cumulative lifetime exposure presented above is based upon the current OSHA PEL of 0.1 f/cm3 whereas the limit between 1976 and 1985 was 2 f/cm3 (90 f/cm3 year) or the lower limit of 0.2 f/cm3 between 1986 and 1994 (9 f/cm3 year). Such low levels are also in agreement with the findings of Paustenbach et al. (Citation2003) in their review of data from nearly 200 brake jobs which showed estimated and measured 8-h TWAs of between <0.002 and 0.68 f/cm3, with a mean of 0.04 f/cm3 for mechanics servicing automobiles and light trucks. Higher levels were found for mechanics servicing heavy trucks and buses (range <0.002–1.75 f/cm3, mean of 0.2 f/cm3) which is somewhat higher than the average 8-h TWA of 0.009 f/cm3 for mechanics during brake removal from heavy vehicles as determined by Madl et al. (Citation2009). Both averages are below current occupational exposure limits.
These levels determined by the Finely, Paustenbach, and Madl studies were lower than those found within European studies where mean cumulative exposures were 0.54, 2.2, and 2.6 f/cm3 year (Rodelsperger et al. Citation1986; Gustavsson et al. Citation1990; Plato et al. Citation1995) but with upper ranges as high as 13 f/cm3 year in bus garage workers (Gustavsson et al. Citation1990). Even within this latter study of bus garage workers, analysis of lung cancer risk showed increasing risk with increasing cumulative exposure to diesel exhaust, but not with cumulative “asbestos” exposure (Gustavsson et al. Citation1990), nor did the later study by Plato et al. (Citation1995) show significant alteration of lung function.
In recreating historical activities with chrysotile containing brake pads, Mowat et al. (Citation2005) determined airborne exposure to chrysotile during the sawing, sanding, drilling, and clean-up of dust from asbestos containing phenolic (Bakelite) molding material. They found chrysotile in 25 of the 40 personal samples collected although estimated 8-h TWAs ranging from 0.006 to 0.08 f/cm3 based on undertaking these activities for 0.5–2 h per day (reasonable given the varied work activities of a mechanic).
An interesting study design sought to reconstruct an exposure scenario for asbestos containing brake pad maintenance to assess possible “asbestos” fiber exposure (Blake et al. Citation2003). Rather than simply machining chrysotile-containing brake pads, the authors instead used a far more elaborate reconstruction whereby four identical passenger vehicles from the 1960s were fitted with chrysotile-containing brake pads. The vehicles were then driven over a distance of ∼2253 km before being brought into a repair facility and the brakes replaced with new chrysotile-containing brake pads using tools, methods and working conditions common to the 1960s. A range of different activities were assessed for the release of asbestos fibers and analysis by PCM and TEM (NIOSH Method 7400 and 7402, respectively) showed only chrysotile asbestos was released.
To the most part, chrysotile was the only asbestos fiber noted in the analysis of air samples taken during brake repair and servicing. However, a study addressing potential “asbestos” exposure in mechanics in Iran replacing chrysotile-containing brake pads in 2008 found that while the majority of fibers detected were chrysotile (∼70%), tremolite and actinolite fibers were also detected (Kakooei et al. Citation2011). The reason for the detection of amphiboles in this study yet not others may be due to several factors. The first is whether or not the imported chrysotile used within the production of these Iranian brake pads possessed a significant level of amphibole contamination and if this markedly differed from that of other brake pads. It is not clear if the imported chrysotile used within the manufacture of these brake shoes was the same as that used in the manufacture of chrysotile sheet cement, also within Iran. However, an exposure study in an chrysotile asbestos cement manufacturing plant by some of the same authors found 44.1% of asbestos fibers detected within air samples were amphiboles (Panahi et al. Citation2011). The second factor is the potential impact of the detection method. As the authors note, OSHA specifies the use of PCM measurements for occupational exposure to “asbestos” fibers and so many studies employ the NIOSH 7400 and 7402 methods. However, these methods are limited to the detection of fibers greater than 0.25 μm in diameter and so thinner fibers may not be counted but are still biologically relevant. Kakooei et al. (Citation2011) however employed PCM and SEM based on methods by the Asbestos International Association (AIA Citation1984) which allowed counting of much thinner fibers also.
In the 1976 study by Rohl et al. (Citation1976), the concentration of chrysotile fibers during automobile brake service at franchised auto dealer garages, taxi fleet repair shops and a municipal truck repair shop in New York City. Across four samples taken during the blowing out of brake drum dust with compressed air concentrations ranged from 6.6 to 29.8 fibers/mL (mean 16.0) within a few feet of the operator. Even within a distance of 10–20 ft of the activity, mean fiber concentration was 2.6 fibers/mL. During light grinding of brake shoes, mean fiber concentrations were 4 fibers/mL while very high mean concentrations of 37 fibers/mL were found during beveling of truck break shoes (Rohl et al. Citation1976).
Even just within this partial summary of exposures measured in various brake repair workshops, it is clearly evident that there is a high degree of variability both within and between studies. This is in itself unsurprising given the wide variety of factors that can affect exposures in an occupational environment. Differences in size and ventilation in the workshop, numbers of brake repairs undertaken, and individual work practices can all have profound effects on exposure even before study design and analysis methods are taken into consideration. As with any exposure characterization study, not least of the occupational environment, there is always the wish for more information, be that specific characterization of the particle properties, exposure scenario, duration, etc. Studies have become progressively more detailed which is important in linking exposure to disease yet historical data (as well as more modern studies) can be severely lacking.
Overall, there is evidence that in some studies there is evidence of exposures that exceed occupational exposure limits for chrysotile although the frequency and duration of such exceedance varies. In broader analysis of historical data as well as more recent recreations, estimated as well as measured 8-h TWAs also indicated that levels firmly below exposure limits. It is self-evident that where exposures are below limits, the risk of MM and other “asbestos” related diseases is minimal and where these limits are exceeded, the risks increase. The frequency, duration and degree to which limits are exceeded will have a significant impact on the relative risk of disease.
Incidence of “asbestos”-associated disease in mechanics
This review is primarily focused on MM, however it is useful to also consider the prevalence of other “asbestos”-associated diseases as this provides further background as to the impact of chrysotile exposure in this exposed population.
The published literature regarding “asbestos”-associated disease in vehicle mechanics consists of individual case studies and broader epidemiological studies. It is these larger studies that are most often considered the “gold standard” as they are able to look at the incidence within the worker population, thereby addressing the relative association between “asbestos” exposure in brake mechanics and MM. This is important as while MM is a very rare disease in the absence of “asbestos” exposure, there is a background incidence which is difficult to addressed in isolated case studies. This is not to say that case studies are in some way inferior, they simply serve different purposes to epidemiological studies and each have their own benefits and drawbacks. Case studies play a key role in identifying common factors such as key exposures and potential risk factors through more in-depth patient history. However, case studies may also suffer from observer bias whereby a focus is placed on presumed source exposure as a causal event rather than more broadly. Furthermore, and key to assessing the broader issue of excess risk of MM owing to chrysotile exposure from brake debris, is that a case study does not facilitate the testing of hypothesis related to elevated risk from a given exposure (i.e. they do not provide evidence of causation (Wong Citation2006)). Larger, epidemiological studies test the association between a risk factor (i.e. chrysotile exposure) and disease (MM), and as they seek to identify and correct for confounders (e.g. diesel exhaust exposure) and disease incidence in the wider population, they can more effectively show a causal link. This is why epidemiological studies can be particularly useful where the cause of a common disease (e.g. lung cancer) may be multi-factorial and relatively low risk. Case studies can be particularly valuable where diseases are rare with a single or limited number of risk factors, such as is the case with MM.
Regarding epidemiological studies and also, with any investigation, there are many variables to account for and critical decisions such as the selection parameters for a control cohort (e.g. age, sex, occupation, etc.) can have profound effects on the outcome. An additional issue, particularly with very large studies is the depth to which a researcher may be able to go into a specific occupational history and exposure. Estimates often are based on job titles and as such, subject to considerable variability, not least when addressing multiple workplaces where attributes such as room size, ventilation, site practices, etc. will affect exposures.
Malignant mesothelioma
Taken on balance, epidemiological studies, for the most part, do not support an excess risk of MM in auto mechanics. Further, meta-analysis studies of the available data have similarly endorsed a lack of casual relationship. One of the most recent analyses of relative risk estimates for MM in mechanics was published in 2016 by Garabrant et al. (Citation2016a). This meta-analysis was based upon screening of the broader literature using a range of search terms intended at capturing information on MM incidence in mechanics more generally, as well as brake repair more specifically. This latter activity being more directly associated with occupational exposure to chrysotile although as noted by Teschke (Citation2016) there may well be considerable variation in such exposures (see Garabrant et al. Citation2016b for response to Teschke). Of 996 records reviewed, 17 were determined suitable for a quantitative meta-analysis based on systematic review of study quality using published criteria. Summary relative risk estimates (SRREs) for all studies was 0.80 (95% CI: 0.61–1.05) leading them to conclude no statistically significant increases in MM among mechanics (Garabrant et al. Citation2016a). Such findings are in line with values determined by Wong (Citation2001) of six studies (shown in ). These studies represent ∼1500 MM cases and Wong et al. determined a relative risk of 0.90 (95% CI 0.66–1.23) for automobile mechanics. Similar conclusions have been established by Laden et al. (Citation2004) and Goodman et al. (Citation2004) although are not universally shared (Egilman and Billings Citation2005).
Table 1. Summary of study findings on relative risk of malignant mesothelioma amongst automobile mechanics. Adapted from Kelsh et al. (Citation2007).
An updated summary of findings from studies addressing MM among automobile mechanics from Kelsh et al. (Citation2007) is presented in . As noted elsewhere, this is not a comprehensive review of every analysis pertaining to MM in mechanics. It is primarily to demonstrate the apparent disparity between exposure to a known carcinogen yet lack of a clear link to excess disease risk in the exposed population. For a more detailed review, readers are directed to the original table by Kelsh et al. (Citation2007) and Garabrant et al. (Citation2016a).
Teschke (Citation2016) makes an interesting point regarding the challenges of assessing exposure–response relationships in occupations with highly variable exposures. In relation to job titles, broad categories such as ‘garage workers’, ‘auto repair and related services’, and ‘auto mechanics’ can represent a very broad range of activities, many of which do not involve brake repair and as such would likely have chrysotile exposures similar to background. Even more specific categories are still subject to high degrees of exposure variability due to the multi-faceted nature of vehicle maintenance and repair (i.e. brake work likely comprises a sub-task of a mechanics role with frequency dependent on demand). As such, when examining the link between chrysotile exposure during brake repair and the development of disease, it is important to focus on those individuals with exposure rather than simply job categories. As noted by Teschke, use of an occupation – response approach in an occupation where exposure is highly varied may well lead to attenuation by subjects with background or low exposure levels (Teschke Citation2016). Such an approach would address the question of if mechanics as a broad occupational category are at excess risk of MM or other disease but may not adequately address if chrysotile exposure acquired during brake repair leads to increased risk of disease. Given this point, it is useful to not only consider larger studies, but also case studies.
One of the first case studies of MM in a brake worker to be reported was published in the Lancet by Langer and McCaughey (Citation1982). It described the case of a 55-year-old man with 36 years of occupational history servicing automobiles and replacing brake linings with no other history of occupational contact with “asbestos”; chrysotile or amphiboles. Analysis of digested lung tissue using electron microscopy showed the presence of mostly non-fibrous particulates but under high magnification (×10 000), chrysotile fibrils became apparent. Most were relatively short, however approximately 10% were longer than 10 μm demonstrating long fiber exposure. The lack of alternative exposures to such fibers, the long history of possible exposure and the tissue analysis of fiber burden coupled with no reported amphibole fibers in the examined lung tissue makes this a compelling case study. More recent published case studies relating to legal cases involving mechanics with MM also exist within the literature (Finkelstein Citation2015; Meisenkothen Citation2017).
While compelling case studies such as those described do exist, others are less so and reflect the challenges of occupational history and latency. A case study published Huncharek et al. (Citation1989) described a nonsmoking 47-year-old who had worked as a mechanic for 11 years preceding his diagnosis with MM and described chrysotile exposure from clutch and brake repair work (Huncharek et al. Citation1989). The man had previously been employed at a young age as an aircraft mechanic and had various jobs in heavy equipment and automotive factories but his only recalled exposure to “asbestos” was as a brake mechanic. While the case study suggests a causal link between exposure as a brake mechanic and pleural MM, the relatively quick onset of MM given exposure much later in life casts possible doubt as to the causal link. Mesothelioma typically has a much longer latency period and a study by Lanphear and Buncher (Citation1992) reviewed 21 articles comprising 1105 cases and established that of these, 99% had a latent period of more than 15 years with a median estimated latent period of 32 years from initial exposure. A more recent study of Italian cases estimated the latency period to be as high as 44.6 years (Marinaccio et al. Citation2007). While such an early onset would be considered atypical, it is not impossible but the presence of a history of likely earlier exposures (e.g. aircraft mechanic) which would correspond to a more typical latency period provides a more credible hypothesis.
Pleural plaques
Concerning PP, a European study investigated the impact of “asbestos” exposure in car mechanics across 925 car mechanics and 109 controls (Marcus et al. Citation1987). Within this population, 41 cases of PP were identified and none in the control population. A later study by Ameille et al. (Citation2012) sought to address nonmalignant disease in automobile mechanics, specifically the frequency of pleural and peritoneal abnormalities. Using high-resolution computed tomography (HRCT) imaging, they investigated a cohort of automobile mechanics with no other known source of “asbestos” exposure, referred for routine screening for “asbestos” – related diseases. Of the 103 participants, PP were noted in five cases (4.9%) including one case of asbestosis. Following further analysis to correct for confounders, the authors noted a significant correlation between occupational exposure to “asbestos” and PP. However, the low prevalence despite the use of a highly sensitive diagnostic tool suggests low cumulative exposure (Ameille et al. Citation2012). The most recent study addressing PP in automobile mechanics found that in a group of 10 mechanics working with chrysotile-containing brake pads in Bogotá, Colombia, three had evidence of PP (Cely-Garcia et al. Citation2015).
While limited in number; these studies do indicate that there is a low but definite prevalence of PP in mechanics working with chrysotile. As PP are often considered to be benign and mostly asymptomatic although there have been associations with reduced lung function parameters such as FEV and TLC (Clin et al. Citation2011). However, the clinical significance of this and causal relationship (as opposed to unidentified abnormalities or other factors) is debated (Kerper et al. Citation2015) and may mean the wider prevalence may not be detected. However, while PP is a clear marker of exposure, they are not pre-malignant, and association with MM is based on common exposure.
Lung cancer
Attributing lung cancer purely to “asbestos” exposure is difficult given the numerous risk factors for this disease, not least tobacco smoke exposure. Indeed, it has long been recognized that co-exposure tobacco smoke and asbestos fibers can have a significant effect on the risk of lung cancer (Vainio and Boffetta Citation1994; IARC Citation2012). Both tobacco smoke and “asbestos” fibers are complex carcinogens and the early events initiation through to the development of lung cancer is also highly complex. Such complexity is thought to be reflected in the observation that relationship between tobacco smoke and “asbestos” ranges from supramultiplicative to less than additive (Henderson et al. Citation2004). A central component of the mechanisms of such a synergistic effect is role of reactive oxygen species (ROS) which can be produced by both exposures, leading to oxidative stress/damage and persistent inflammation (Valavanidis et al. Citation2013). Co-exposure with cigarette smoke can also enhance retention of chrysotile and amosite in humans (Churg and Stevens Citation1995) as well as increase the fibrogenic effects of asbestos (Churg Citation2003).
While co-exposure to tobacco smoke and asbestos has a synergistic relationship in the development of lung cancer, there is no such relationship regarding mesothelioma (Henderson et al. Citation2004; Berman and Crump Citation2008b).
A critical review of epidemiologic studies of lung cancer by Laden et al. (Citation2004) found no significant increase in the risk of lung cancer among male automobile mechanics occupationally exposed to “asbestos” from brake repair. Similarly, a study by Gustavsson et al. (Citation1990) addressing bus garage workers showed no increased risk with cumulative “asbestos” exposure but did show increasing risk with cumulative exposure to diesel exhaust, a Group 2A carcinogen (IARC Citation2014).
Lung fibrosis (asbestosis)
There are limited studies on the prevalence of asbestosis in vehicle mechanics and given the relatively high exposures to “asbestos” associated with asbestosis, this is unsurprising given the nature of exposures in this occupational group. A study by Boillat and Lob (Citation1973) looked at nine worksites in Vaud, Switzerland and fiber counts at three sites noted a maximum of 13.8 f cm–3. Through lung function and radiographic analysis of 39 workers, there were no definitive cases of asbestosis although results from two participants were considered uncertain. A much more recent study by Erdinc et al. (Citation2003) looked at the respiratory health of 74 “asbestos” exposed automobile mechanics as well as 12 unexposed office workers in 1992 and then again seven years later. Changes were noted in 10 workers who were found to have peribronchial thickening attributed to smoking although there was an absence of radiographic asbestosis (Erdinc et al. Citation2003).
The lack of asbestosis cases associated with chrysotile exposure from brake repair is perhaps not surprising. As noted by Lemen (Citation2004), with improvements in dust control measures the risk of developing asbestosis is “probably eliminated but the risks of cancer are not”.
In summary, there are various case studies of MM in mechanics yet epidemiological studies, on the whole, do not support an excess risk in automobile mechanics. There appears to be more of an association between brake repair involving chrysotile-containing brake pads and PP, yet the studies are somewhat limited in number. There seems to be little evidence of asbestosis in mechanics exposed to chrysotile via brake work, and similarly, evidence for excess lung cancer risk associated with chrysotile exposure in this occupational group is limited. As noted above, there is clear evidence for exposure to chrysotile among mechanics working in brake repair, handling and machining chrysotile containing brake shoes yet there is little conclusive evidence of an excess risk of MM, the most specific and sensitive forms of asbestos-associated disease. It seems somewhat counter-intuitive that there can be repeated occupational exposure to chrysotile, a Class 1 carcinogen, at levels that can exceed exposure limits yet no increased risk of disease. Chrysotile is undoubtedly not an inconspicuous mineral fiber and rather than promulgate the contention that chrysotile is non-harmful, our focus is to consider if chrysotile released from brake shoes is modified in some way as to alter its toxicological profile a thereby account for this risk discrepancy.
Toxicology of asbestos-containing brake debris
There appears to be limited toxicological evidence surrounding chrysotile-containing brake debris with, to date, only a handful of experimental papers setting out to explore potential toxicity. One of the earliest studies carried out by Davis et al. addressed the biological effects of heated chrysotile and brake lining dust in a murine model of both intrapleural and intraperitoneal injection up to one year following exposure (Davis and Coniam Citation1973). The authors were able to show that the intrapleural response to chrysotile asbestos, which had been heated to a variety of temperatures up to and including 1000 °C, was one which was driven more by the altered physical shape of the particles and fibers, i.e. samples where the majority of particles where of low aspect ratio, than the actual change in dust chemistry. Interestingly, when a sample of brake lining dust was examined under electron microscopy, it was found to contain very little evidence of recognizable chrysotile. Furthermore, intrapleural injection of the same sample into mice produced few and little granulomas as well as minor fibrosis (Davis and Coniam Citation1973). More recently, work by Bernstein et al. (Citation2015) set out to understand the biokinetics and potential toxicity in the lung and pleural space following inhalation of brake dust from chrysotile containing brake drums. In this study the authors' exposed rats via inhalation (6 h/day for five days) to filtered air (control), brake dust derived from chrysotile containing brake pads, a mixture of pure chrysotile asbestos and brake dust or the amphibole asbestos, crocidolite (positive control). In animals taken out to one year after exposure, the authors reported that there was no statistically significant evidence of a pathological response noted at any of the measured time points following either brake dust or brake dust/chrysotile mix. In contrast, animals exposed to crocidolite showed inflammation of the lung and pleural cavity as well as a significant fibrotic response. The authors attributed this, in part, to the relatively biosoluble nature of the chrysotile fibers when compared to the more biopersistant crocidolite asbestos which persisted in the animals through the lifetime of the study (Bernstein et al. Citation2015). Although a well-designed and executed study, the work by Bernstein et al., perhaps lacks a control group of chrysotile alone exposed animals which would have helped to understand further and benchmark the nature of the in vivo response to chrysotile asbestos within a brake debris matrix.
Biological effective dose
When considering the toxicology of asbestos fibers or any particle type for that matter, it is essential to understand the idea of the biologically effective dose (BED). The most fundamental principle in toxicology is that the “dose makes the poison” and the concept of dose for particles can be thought of more accurately as what is driving the response. In conventional particle toxicology, the dose is defined by mass or concentration of particles per unit tissue or per number of cells or surface area of cells in cell culture (Donaldson et al. Citation2012). Mass is the way that particles are often measured in the workplace and the environment for risk management purposes, with the key exception of fibers that are counted by number. Povey (Citation2008) define the BED as “the active dose of the agent of interest”. Also, that “the nearer the dose specified can be to the active dose of the real agent of interest (the BED), the more likely it is that an association may exist between an agent and a disease” (Povey Citation2008). More specifically, we have defined the BED in particle toxicology as “the entity within any mass dose of particles that drives a critical pathophysiological relevant form of toxicity in tissue, such as inflammation, genotoxicity or cellular proliferation” (Donaldson et al. Citation2012). If only a portion of the total mass dose is the BED, then the implication is that a quantity of the retained mass dose is not biologically effective. Toxic effects are subtle and complex and invariably depend on molecular effects at the cellular level that depend on the physicochemical properties of the particles. Therefore, mass is a surrogate for the critical dose and increasing mass increases the delivery of the dose that drives the toxic effect. The BEDs of some of the best known pathogenic particles have been identified; for example, in the case of quartz it is the active surface area, and in the case of pathogenic fibers such as RCF and “asbestos”, it is the long, biopersistent fibers. It is notable that despite knowledge of the BED for some particles, they are still measured by mass since the variability in the BED between particle types and the difficulty in measuring the BED currently precludes it.
The role of physicochemical properties in particle toxicity: implications for brake debris
The two driving forces in the toxicity of particles and fibers are the physicochemical characteristics and the dose. The physicochemical characteristics define the nature of the biological interaction and relative “potency” while the dose largely defines the toxicity. A very small dose of a hazardous particle may not cause a relevant biological response, pathology or disease but a very large dose of even a low toxicity particulate can, conversely, lead to disease. It is for this reason that simply relying on name is somewhat unhelpful when trying to define potential hazard and subsequently, risk. This is because name often refers to bulk composition (e.g. TiO2) rather than physicochemical characteristics (e.g. anatase TiO2 fibers, 0.2 µm diameter, 22 µm long with a coating of Y and surface charge of X). The latter being far more informative in terms of hazard identification and risk analysis. In this respect, the term “asbestos” is unhelpful as it tells us very little about the fiber in question. It does not describe the diameter (important in determining aerodynamic diameter and hence, lung deposition), length (important in frustrating cell mediated clearance), nor composition (important in determining biodurability and possible reactivity). Even narrowing down to, for example, the term “amosite” tells us more but still not enough in terms of the possible toxicity of a material. For example in the study of Davis et al. (Citation1986), a sample of raw amosite with a very high proportion of long fibers were injected into the peritoneal cavity of rats, a surrogate of the pleura cavity. Mesotheliomas were detected in 95% of animals with a mean induction period of approximately 500 days. However, grinding of the long fiber sample produced a sample that was largely the same in terms of composition yet almost all the fibers were <5 µm in length. Injection of same mass dose of the short fiber sample led to only one detected mesothelioma (4%) showing a marked difference in carcinogenicity. A similar differential was also noted after 12-month inhalation despite greater lung burden of the short fibers. The long fiber sample generated widespread pulmonary fibrosis yet no fibrosis after exposure to the short amosite sample. A later study by the same author, this time focusing on chrysotile showed that short fiber chrysotile generated six times less asbestosis despite three times greater lung burden than the long. During the post exposure period, the short fibers were cleared much more rapidly that the long (Davis and Jones Citation1988).
These, as well as more recent studies looking at nanofibers (discussed later) demonstrate the profound effect modification of the physicochemical properties of fibers can have on their relative pathogenicity. summarizes what is often referred to as the fiber pathogenicity paradigm or the 3D’s of fiber toxicology namely dose-dimension-durability (Donaldson and Tran Citation2004; Greim et al. Citation2014).
Figure 1. The fiber pathogenicity paradigm relating physicochemical properties to fate of long and short fibers in the body. The items on the left of the diagram are parameters which dictate behavior. Adapted from Donaldson et al. (Citation2006).
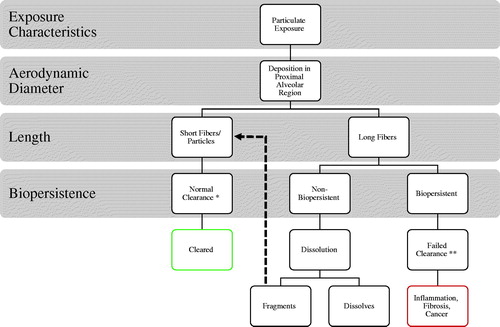
The paradigm itself is robust as it reflects the key physicochemical differences between high toxicity (e.g. crocidolite) and low toxicity (e.g. biosoluble synthetic vitreous fibers (SVFs)) which impact on their relative toxicity. The importance of dose is, in itself, obvious but a key determinate in an alveolar dose is dimension. Diameter is the key factor here as together with density it is a major determinate of aerodynamic diameter and therefore, respirability (Jones Citation1993). In , assuming both long and short fibers are equally respirable and deposit in the alveolar region, the short fibers (i.e. <5 µm) can be internalized and cleared by alveolar macrophages normally. Long fibers (i.e. >15 µm) however frustrate attempts at phagocytosis and hinder effective clearance (Schinwald et al. Citation2012a, Citation2012b). Therefore, short fibers provide much less of a problem for the lung as compared to long fibers and this is demonstrated in the studies of Davis et al. described above. While dimension (both diameter and length) are important factors, a further factor is durability. Fibers that a biosoluble such as certain SVF may be leached in the biological milieu of the lung leading to either congruent or incongruent dissolution. The ultimate outcome is these long fibers either dissolve away (hence no longer present a dose) or progressively fragment, joining the short fiber population which is cleared. This was demonstrated by Searl et al. (Citation1999) whereby the short fiber numbers of the non-durable glass fiber MMVF10 increased over a 12 month period after intratracheal installation in rats. This indicated breakage of the long fibers adding to the population of short fibers. The combination of long length presenting a physical barrier to macrophage clearance from the lung and durability presenting a chemical barrier to clearance by dissolution combine to determine the biopersistence of a fiber. The greater the biopersistence, the greater the dose (owing to accumulation) and the greater the pathogenic effect (discussed later).
The following sections discuss the relative impact of various physicochemical characteristics on particle/fiber toxicity and their implication for chrysotile within brake debris.
Fiber length
It is worth considering that the toxicity of a particulate toward the mesothelium does not simply rely upon surface reactivity but also on retention. A simple demonstration of the importance of retention on eliciting a localized response within the pleural space was shown by Murphy et al. (Citation2011). Using a direct injection approach to deliver a range of particulates to the pleural cavity of C57BL/6 mice, they assessed the acute response to a range of low and high toxicity particulates. These included a crystalline silica (Dörentruper quartz (DQ12)) known to be highly inflammogenic in the lungs and pathogenic in vitro (Clouter et al. Citation2001; Bruch et al. Citation2004; Peeters et al. Citation2014), coal dust and low toxicity polystyrene beads of 3 μm and 10 μm in diameter. All of the particles with the exception of 10 μm polystyrene beads were sufficiently small to exit the pleural cavity via pore-like stomata within the parietal pleura, a major clearance route to the mediastinal lymph nodes (Wang Citation1975, Citation1985; Sahu and Casciano Citation2009). Despite the inherent toxicity of DQ12, injection of 5 μg per mouse led to no significant increase in granulocyte number in the pleural lavage fluid (a marker of acute inflammation); a response replicated for coal dust and 3 μm beads. In contrast, the 10 μm beads caused a significant (p < 0.001 vs. vehicle control) increase in granulocytes indicating irritation of the mesothelium despite the fact that relative to crystalline silica, the polystyrene beads possess a benign surface. These results demonstrate clearly that irrespective of inherent surface related toxicity, only those particles retained may cause a mesothelial response. In addition, using dynamic SPEC/CT imaging, radiolabelled short carbon nanotubes were monitored from the point of injection for their regional distribution. Initially, a diffuse signal across and confined to the pleural cavity was seen yet within an hour began to accumulate in the cranial mediastinal lymph nodes where by 24 h, it was almost exclusively located (Murphy et al. Citation2011). The control [111In]DTPA label alone showed a different distribution with rapid translocation to the bladder within the first hour suggesting a specific route for particle clearance. What this and numerous other studies have shown is that retention in the pleural space is key for the elicitation of toxicity (Davis et al. Citation1986; Poland et al. Citation2008; Poland et al. Citation2012; Schinwald et al. Citation2012a, Citation2012b). Particles short enough to pass through the pleural stomata and enter into the underlying lymphatic system do not present a sustained dose at the mesothelial surface and are instead, cleared rapidly causing minimal effect. In contrast however, larger particulates (i.e. long fibers) that transit into the pleural space can be retained and accumulate with repeat exposure leading to a pathological response with possible progression to clinical disease (Donaldson et al. Citation2010).
The idea of the importance of physical parameters for fibers, i.e. length, diameter and composition was first described by Stanton and Wrench for materials such as “asbestos”, aluminum oxide and fibrous glass (Stanton and Wrench Citation1972). The authors demonstrated that for a range of “asbestos” samples, the production of MM required a significant number of those fibers to be greater than 10–20 µm in length. While this did seem to be the case for “asbestos” fibers, certain glass fibers which contained a large proportion of fibers longer than 10–20 µm seemed to produce a much smaller pathological response that would have been predicted given the number of long fibers within the samples. Interestingly, while not a major point of discussion, this observation was one of the first ideas of the role of biopersistance which nowadays is one of the fundamental concepts defining the fiber pathogenicity paradigm (Donaldson et al. Citation2010). More than a decade on from that publication, Stanton had accumulated considerably more data and was able to conclude that:
…The probability of pleural sarcoma (mesothelioma) correlated best with the number of fibers that measured 0.25 µm or less in diameter and more than 8 µm in length, but relatively high correlations were also noted with fibers in other size categories having diameters up to 1.5 µm and lengths greater than 4 µm…. (Stanton et al. Citation1981)
A major hurdle facing research aimed at demonstrating a specific threshold length for fibers is the simple fact that naturally occurring fibers are by definition a heterogenous mix in terms of their length. The exception to this is one where intentionally milled fibers can all be short, but all long fiber samples may contain short material in addition to longer fibers of varying lengths. Thankfully, with advances in nanotechnology and in particular, materials science, it is now possible to produce materials with discrete length distributions which therefore offers the possibility for the accurate determination of the threshold length for pleural retention/reactivity. Work by Schinwald et al. utilized just such an approach with a range of nanofibers of varying composition (silver, carbon, and nickel) and discreet lengths (Schinwald et al. Citation2012a, Citation2012b). The different fiber samples were directly applied to the pleural space followed by sampling of the pleural space for evidence of acute pathogenic effects. A very clear threshold for fibers ≥5 µm in length inducing pleural inflammation was noted whereas none of the shorter fibers (≤4 µm) produced an inflammatory response despite considerably different chemical composition. Above the length threshold the response flattened off which may be a consequence of long fibers aggregating in the restrictions of the pleural space. This was the first study showing a quantitative association between fiber length and pleural inflammation using fibers of different kinds in tightly defined length classes. The identified threshold length of 5 µm provides persuasive toxicological confirmation of a hypothetical length threshold that had evolved over the previous half century and so represents an important step forward.
While these studies showed selective retention of longer fibers in the pleural space with subsequent inflammation displaying a clear length cutoff, these were acute effects and such a response is not MM. However, more recent studies in rodents have shown that carbon nanotubes can cause MM after direct application to the mesothelial surface and thus far the scientific data largely support the contention of size related generation of MM. An early 2-year carcinogenicity bioassay (direct injection into the peritoneal space as a surrogate for the pleural space) showed no evidence of MM yet the carbon nanotubes used were very short (<1 μm) yet based on the length threshold hypothesis, no MM would be expected (Muller J et al. Citation2009). More recently a large study by Rittinghausen et al. found that pleural exposure to four different carbon nanotube samples, all of which possessed a mean length greater than 5 μm, led to 70–98% induction of MM (Rittinghausen et al. Citation2014).
Biopersistence
Biopersistence of a material relates to its ability to withstand removal from the biological environment. The biopersistence of a particle or fiber is dictated as a combination of chemical dissolution in the biological milieu (i.e. biodurability) and physical clearance such as by alveolar macrophages in the lung. The length component in resisting physical removal from the lung has been addressed in depth in the preceding section and therefore the focus here is on impact of dissolution on fiber biopersistence.
While “asbestos” is often referred to collectively, the composition of the differing forms of “asbestos” differ greatly and this is most apparent when comparing serpentine (chrysotile) and amphibole forms. Amphibole fibers such as amosite, crocidolite, and tremolite are able to persist in the body with little change for decades, facilitating not only dose accumulation with repeated exposures but also chronic interaction with the biological environment. In contrast, chrysotile has been shown in various studies to be less biopersistent in the lung although the specific half-life (WT1/2) of fibers >20 µm (i.e. those resistant to physical clearance) varies by study and source of chrysotile. At the most rapidly clearing end of the spectrum is Calidria chrysotile which, after inhalation exposure in rodents was shown to have a WT1/2 for fibers >20 µm of only 7 h (Bernstein et al. Citation2005). This exceptionally rapid removal of long fibers is likely to reflect the structure of the long Calidria chrysotile fibers rather than chrysotile more generally. This is because the Calidria chrysotile fibers were found to be largely composed of multiple shorter fibrils (Bernstein et al. Citation2005) which, in the biological environment may have rapidly dissociated leading to a rapid decrease in long fiber dose. Longer chrysotile WT1/2 for fibers >20 µm have been determined for “Brazilian chrysotile” – 1.3 days (Bernstein et al. Citation2004) and “Canadian chrysotile” – 11.4 days (Bernstein et al. Citation2005). Longer WT1/2 for a different Canadian chrysotile fiber samples >14 µm have been determined by Searl (Citation1997) – 47.6 days, while Coin et al. found an even longer WT1/2 (>16 µm) of 116 days for a separate Canadian chrysotile sample (Coin et al. Citation1992). This variation in biopersistence half-life of chrysotile is unsurprising for a variety of reasons, not least differences in experimental design and analysis owing to lack of an applied standard approach within earlier studies. A strength of the later Bernstein studies is that they employ a standardized test method (Bernstein and Riego-Sintes Citation1999) used for determining the half-life of mineral fibers used within EU regulation (Regulation (EC) no. 1272/2008). The use of standardized approaches across different studies (and study authors) would be beneficial. A further reason for the variability, mostly notable demonstrated by the difference between the WT1/2 of Calidria and other sources of chrysotile, is differences in composition and structure. Biopersistence is a function of size and composition and chrysotile from different sources differ in their physicochemical properties to varying degrees and as such, would be expected to differ in relative biopersistence.
The process of dissolution and clearance of chrysotile from the lung is summarized in and described by Gualtieri et al. (Citation2018) as being a two-step process although it may be more holistically described as a three-step process. The first step could be considered as being the longitudinal splitting of chrysotile fibers due to the breakage of weak bonds holding the chrysotile fibrils together. This leads to an initial increase in the long fiber number in the lung, a phenomenon that has been observed in several in vivo studies (Bellmann et al. Citation1986; Roggli et al. Citation1987; Coin et al. Citation1992, Citation1994). The second step is the rapid dissolution of magnesium from the chrysotile fibrils which derives from brucitic layer [Mg(OH)2] which alternates between the silicate layers (Fubini Citation1997) within the fibers as shown in . This layer is known to be susceptible to leaching especially in acidic conditions (Virta Citation2002) such as that of a cell phagolysosome. in vivo radioisotope studies in rats have shown that as much as 35% of the structural magnesium of chrysotile can leached from the fiber within 1 month. Further analysis showed that the surface magnesium which accounted for ∼7% of the test sample dissolved within the first few days after administration (Morgan et al. Citation1971). After leaching of magnesium, a weakened fibrous metastable silicate pseudomorph (Gualtieri et al. Citation2018) is left which is subject to further dissolution (step 3) at a slower rate than that of the bructic layer and transverse fragmentation. As described by Gualtieri et al. (Citation2018), the lifetime of the chrysotile fiber is controlled by the dissolution rate of the silica layer which persists beyond that of the brucitic layer in the form of a pseudomorph.
Figure 2. Diagram of the chemical structure of chrysotile and leaching mechanisms leading to dissolution and fragmentation. (I) Deposition of chrysotile fibers in the lung environment. (II) Longitudinal splitting of the fiber into thinner fibrils. (III) Leaching of magnesium from the brucitic layer resulting in the formation of a weakened metastable pseudomorph formed of the remaining silica structure. (IV) Transverse breakage of the weakened silica structure into short fragments.
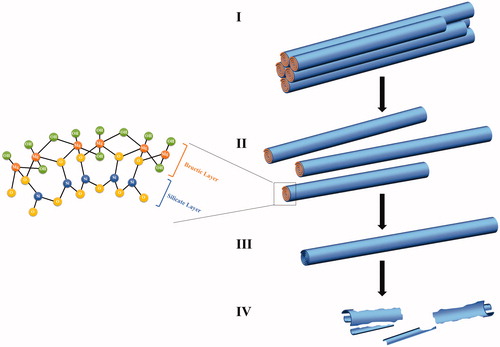
As noted by Coin et al. (Citation1994), the transverse breakage of chrysotile leading to shortening of long fibers is described in numerous studies has been shown to generate an increase in the short fiber population (Searl Citation1997). A longer term study by Kimizuka et al. (Citation1987) found that initially the short fiber population diminished from 38% to 13% (indicating clearance) but by 2 years post exposure had increased to 56%, likely due to fragmentation of long fibers.
This chemical dissolution of magnesium resulting in fragmentation leads to the formation of short fibers which can be more readily cleared, either through the activity of alveolar macrophages (Schinwald et al. Citation2012a, Citation2012b) or passing through the pleural stomata toward the mediastinal lymph nodes (Murphy et al. Citation2011). This clearance means that as compared to more chemically durable fibers (e.g. tremolite), long chrysotile fibers are overall less biopersistent. The overall effect of low biopersistence is the progressive removal of dose from the lungs with time while more durable fibers are retained and accumulate. This is demonstrated by Gilham et al. (Citation2016) who undertook a population-based study of pleural MM patients with occupational histories and measured asbestos lung burdens in occupationally exposed workers and in the general population. They found that of the fibers detected in lung tissue, 75% were amosite, 18% were crocidolite while chrysotile only accounted for 1.9% of the fibers detected. This phenomenon is well known, with chrysotile fibers representing disproportionately small amounts compared to amphiboles despite chrysotile being the main exposure (Churg and Wright Citation1994). One argument against such an observation is that studies such as that by Gilham often count only those fibers with an aspect ratio of >3:1 and length >5 µm. However, studies counting all asbestos fibers show that short chrysotile asbestos fibers do predominate in the lung and pleural tissue of MM patients (Suzuki et al. Citation2005). This is unsurprising given that the principal exposure of such workers is to chrysotile fibers of varying lengths, the low biodurability of chrysotile leads to fragmentation in vivo (thereby contributing to the short fiber population) and shorter, thinner fibers, or compact particles will likely translocate more easily than longer, thicker fibers. Indeed, it has been suggested that in terms of fiber translocation to the parietal pleura, the critical dimensions were a diameter of <0.4 μm and a length <10 μm (Lentz et al. Citation2003). The presence of large numbers of short chrysotile fibers in asbestos workers is therefore a definite marker of exposure but does not necessarily translate to a causal relationship. The conclusion presented Suzuki et al. (Citation2005) is that because short, thin, asbestos fibers were the predominant fiber type detected, they must contribute to the causation of MM. This is a rather rudimentary argument assigning simple primary exposure (dose) as the casual factor in development of MM. Indeed, in other exposures such as carbon particles derived from air pollution, there is a pleural dose in the form of “black-spots” (Mitchev et al. Citation2002). The formation of such black spots closely correlates to lymphatic channels (i.e. routes of egress from the pleural cavity) and are caused by incorporation of foreign particles leading to mild fibrosis and an inflammation (Muller et al. Citation2002). Furthermore, respiratory exposure to crystalline silica, a Class 1 carcinogen (IARC Citation1997), can also result in pleural dose leading to diffuse pleural fibrosis and thickening (Ferrer et al. Citation1994, Citation2008). However, despite these particles being a primary exposure and in the example of quartz is carcinogenic in the lung, they do not cause MM. Given the effect of chronic exposure to particles on the pleural mesothelium (inflammation, fibrosis, and thickening), it is highly likely that chronic exposure to short chrysotile would cause a similar reaction possibly leading to PP. Yet to equate primary dose to the causation of MM is a significant leap, not least as amphibole lung burdens account very well for MM incidence (Rodelsperger et al. Citation1999; Gilham et al. Citation2016).
Considering fiber biopersistence more generally, it is clear that biopersistence does play an important role as the pathogenicity of fibers and has been clearly demonstrated across a wide range of studies. As summarized by Hesterberg et al. (Citation2012) respiratory exposure to higher durability fibers such as refractory ceramic fibers and crocidolite asbestos lead to fibrosis and tumor formation in rodent models while low durability stone wool fibers such as MMVF 34 do not. The importance of fiber biopersistence in dictating carcinogenic potential is underscored by the regulatory evaluation of man-made vitreous fibers (MMVFs) within the EU. MMVF with an alkaline oxide and alkali earth oxide content greater than 18% by weight (EC Citation2008) are classified under regulation (EC) no. 1272/2008 as a category 2 carcinogen. However within Note Q of the regulation, MMVF can be exonerated of the classification as possibly carcinogenic based on suitable experimental evidence indicating a lack of biopersistence and/or carcinogenicity (EC Citation2008). The experimental evidence of biopersistence in this case comes from inhalation or installation exposure of rats to fibers longer than 20 μm (thereby focusing on dissolution rather than cell mediated clearance) demonstrating a weighted half-life less than 10 days (inhalation) or 40 days (installation) (Bernstein and Riego-Sintes Citation1999). Here, the level of biopersistence of a fiber (meeting the 18% rule) is directly linked to its hazard status and labeling as carcinogen.
In vivo biopersistence studies of various different fibers have shown the wide range of lung clearance half times for fibers longer than 20 μm. As summarized by Mossman B et al. (Citation2011), the nontoxic biosoluble fibers MMVF34 and X607 have a rapid T½ of as little as 6 and 9.8 days, respectively (Bernstein et al. Citation1996; Hesterberg, Hart, et al. Citation1998b). This contrasts greatly to the far more durable and pathogenic amosite and crocidolite asbestos which have a T½ of 418 and 536 days, respectively (Bernstein et al. Citation1996; Hesterberg, Chase, et al. Citation1998).
It should be noted that biopersistence is not the sole arbiter of particulate toxicity and there are numerous examples of low aspect ratio soluble particles that cause disease. It is simply that biopersistent particles present a longer-term dose that, due to slow clearance, can accumulate more readily. Also, the target tissues may also be different including associated disease. It is well established that while a wide range of particulates, differing in size, shape, and durability can induce effects such as inflammation, fibrosis, and tumor formation in the lung (as well as effects distal to the route of entry such as cardiovascular effects), MM is limited to a select few fibers.
Fiber heat modification
During braking, the kinetic energy of the vehicle is converted into heat energy through the friction generated by the brake pads being applied to the drum (Belhocine and Bouchetara Citation2012). Similarly, the act of grinding or drilling brake pads can also cause substantial heat. The purpose of asbestos in brake pad composition was to make use of the very high stability of asbestos against mechanical, thermal, and chemical loads (Ostermeyer and Müller Citation2008). However, chrysotile asbestos is not impervious to heat and exposure, even for brief periods to high temperatures can alter the structure of chrysotile fibers. This effect of heat on chrysotile asbestos is described within various studies and articles reaching back to the early twentieth century (Davis and Coniam Citation1973; Valentine et al. Citation1983) but continues to be a topic of interest, including the use of heat treatment as a recycling strategy for chrysotile-containing materials (Gualtieri and Tartaglia Citation2000; Yoshikawa et al. Citation2015).
The effect of high temperatures on chrysotile can be profound, and with increasing heat, chrysotile may undergo thermal decomposition due to the dehydroxylation of the chrysotile fibrils. Specifically, with increasing temperature, chemically bound water within the chrysotile structure is driven off which can lead to an endothermic mass loss between 600 °C and 800 °C (Zaremba et al. Citation2010). According to Langer (Citation2003), this dehydroxylation can begin at temperatures as low as 150 °C with ∼2.5% of the structural water being lost between 150 °C and 500 °C (Hodgson Citation1979). Further water is driven off between 500 °C and 800 °C (Hargreaves and Taylor Citation1946) although almost complete water loss occurs at 650 °C (Langer Citation2003). This results, not only in changes in hydration levels but also significant structural and textual alterations. The process is well described by Zaremba et al. (Citation2010) who note that the dehydroxylation of the octahedrally coordinated hydroxyl groups of chrysotile (Mg3Si2O5(OH)4) lead to a complete breakdown of the mineral structure and the formation of an amorphous mixture of silica and magnesia known as “serpentine anhydride” (Zaremba et al. Citation2010). This amorphous structure is the primary product of the dehydroxylation (Trittschack and Grobéty Citation2013), is unstable (Cattaneo et al. Citation2003), re-crystallization occurs leading to the formation of forsterite (Mg2SiO4) and, with further heating, enstatite (Mg2SiO3) (Koshi et al. Citation1969) in the following process:
Experimental analysis by Zaremba et al. (Citation2010) of this process using X-ray diffraction (XRD) show an interesting progression through the states from chrysotile to the mineral forsterite as summarized as follows:
After heating the raw material up to 600 °C for 3 h, the intensity of the peaks at 7.3 Å and 3.64 Å is slightly decreased and new weak peaks at d = 2.77 Å, 2.51 Å, 2.46 Å, and 1.75 Å appear due to formation of forsterite as a new crystalline phase;
When the sample is heated up to 650 °C, the peaks at 7.3 Å and 3.64 Å disappear indicating the destruction of chrysotile;
After heating of the sample up to 725 °C, the strong peaks of forsterite appear.
When considering the effects of heat on chrysotile fibers, the transformation of chrysotile starts on the wall edges at approximately 450 °C and propagates to the inner walls of the fiber where the layers begin to dehydroxylate only above 600 °C (Trittschack and Grobéty Citation2013). This means that the full structural reorganization and re-crystallization may only occur once both the chrysotile wall edges and inner layers have undergone dehydroxylation yet heat alteration would begin first at the fiber wall edge and penetrate with time. This may result in partially modified chrysotile fibers where temperature/heating time is insufficient to allow full penetration of the fiber.
The importance of heat modification of chrysotile stems from the changes to the toxicological profile of the heated chrysotile. A study by Valentine et al. (Citation1983) examined the impact of heating on chrysotile toxicity toward cultured human fibroblasts and bovine alveolar macrophages. They found that heating of Canadian chrysotile to either 200 °C or 400 °C led to ∼40% increase in macrophage viability as compared with unheated chrysotile. Also, the authors also showed that with increasing temperature (70 °C, 200 °C, or 400 °C) there was a reduced growth inhibition in fibroblast cultures. The reason behind the modified toxicity was thought to be due to the observation of temperature-dependent luminescence of heated asbestos fibers. This thermoluminescence is believed to be caused by the thermally induced release of electrons trapped within asbestos lattice imperfections leading to a modification of surface charge and hence, modified membrane interactions and lower molecular adsorption. It is interesting to note that the authors also found that irradiation of heated asbestos regenerated the thermoluminescent pattern of thermally discharged asbestos minerals and that such irradiation resulted in reactivation of asbestos cytotoxicity (Valentine et al. Citation1983). Studies using IR spectroscopy point toward modification of external hydroxyl group population on the surface of chrysotile fibers while heat treatment followed by irradiation may re-populate this functional group (Fisher et al. Citation1987).
An additional impact of heat on chrysotile is an alteration of their biodurability and hence, biopersistence. Using an acellular in vitro biodurability approach with simulated lung fluids at pH 4.5, Gualtieri et al. (Citation2012) demonstrated that chrysotile heated to 1200 °C had a much lower dissolution time compared to unheated chrysotile (20 days vs. 298 days). The lower biodurability would be expected to result in a lower biopersistence through a combination of increased dissolution rate and decreased length due to loss of asbestiform habit and changed chemical composition leading to reduced strength and fragmentation (Giantomassi et al. Citation2010).
While the somewhat limited in vitro studies examining the toxicity of heated chrysotile show a marked reduction in toxicity, likely due to modification of surface properties, in vivo studies have proved somewhat equivocal. This may, in part, be due to the different transitional states with increasing temperature. Heating of chrysotile has been shown to cause an increase in the fibrogenic activity after lung instillation with increased activity noted between 150 °C and 800 °C as compared to unheated chrysotile but with most prominent effects seen after heating at 600 °C (Wozniak et al. Citation1991). With increasing temperature to 1200 °C, there was no increased toxicity over untreated chrysotile. This change in response to rising temperatures may account for the apparent disparity with a later study by Takata et al. (Citation2009). Here the authors examined if the pulmonary response to forsterite differed to that of unheated chrysotile. Using a high intratracheal dose of 1 mg, rats were exposed to both fiber types and the response examined 3–7 days post exposure. Chrysotile caused a significant inflammatory response with high concentrations of the oxidative DNA adduct 8-hydroxy-29-deoxyguanosine (8-OHdG) within granulomas as well as bronchiolar and alveolar epithelial cells (Takata et al. Citation2009). Treatment with forsterite led to a much more muted inflammatory response, no progressive fibrosing lesions and weak, transient 8-OHdG expression indicating significantly less oxidative DNA damage. It is worth noting that the forsterite sample contained far fewer fibers than the chrysotile sample (50% vs. 96% of the total particulates, respectively). This muted response seems to contradict that observed by Wozniak, yet in the Takata study, the fibers were heated to 1000 °C to generate the forsterite sample. It may well be the case that if a lower temperature was used in the Takata study (i.e. not resulting in the formation of forsterite), that a reduction in toxicity may not have been observed.
A key variable in the potential modification of toxicity resulting from heating is temperature achieved (and duration) at the brake pad surface during grinding and if these are commensurate with those required for thermal degradation of chrysotile. Considering the regular use and wear of brake pads, a desirable level of thermal tolerance needed of a chrysotile replacement for brake pads as described by Paustenbach et al. (Citation2004) in their review of asbestos in brake linings was 500 °C. This temperature is at the borderline of where heat alterations of chrysotile may begin to occur. This thermal tolerance is in line with modern assessments of disc/pad interface temperatures in friction braking as described by Qi and Day (Citation2007). Here, the authors investigated the factors affecting the interface temperature, including the number of braking applications, sliding speed, braking load and type of friction material and results showed temperatures below 500 °C although this may not represent the brake temperatures noted in vehicles of the 1960s/70s. In a review by Langer (Citation2003), the author reported that pads could be subjected to extreme thermal and shear stress leading to “normal” service temperatures of up to 650 °C but also “hot spots” with temperatures well reaching as high as 1000 °C. However, the operational temperature of brake pads is likely to be very much dependent on the nature of braking with longer durations or braking in rapid succession likely to lead to a higher build-up of heat than short, infrequent braking. Similarly, the load may have a bearing with the temperatures achieved during heavy vehicle breaking being higher than those of lighter vehicles. While this provides insight into possible heat modification of fibers in brake dust to which mechanics can be exposed during changing of old brake pads (e.g. due to the blow-out of old dust), it tells us little of the temperatures achieved during grinding (i.e. modification of new brake pads). While the action of grinding the face of brake pads will naturally lead to the generation of heat, the temperature ranges typically achieved during grindings have not been established.
It is clear that heating of chrysotile will undoubtedly occur during the production of brake pads but also during the machining during fitting and most notably, during operation. Heating does affect the toxicological properties of chrysotile, likely due to alterations of the surface properties at lower temperatures and destruction of chrysotile at high temperatures (>1000 °C). However, the level and duration of heating during grinding and use wear is likely to be highly variable and be very much operator (mechanic or driver) dependent.
Fiber/matrix interactions
Brake pads are a complex mix of binders, fibers, fillers, frictional additives, and abrasives (Grigoratos and Martini Citation2015). In their production, the particulate components are bound together with a resin, compressed and cured to form a solid brake pad. As such, it is feasible that the resin (with or without other bound particulates) may adhere to chrysotile fragments and fibers even after their release through grinding. Such surface binding was noted by Weir and Meraz (Citation2001) who described the presence of adhered resin matrix as particulate fragments bound to parts of a fiber, but there is also the possibility of a surface coating.
When considering the impact of matrix binding on the toxicity of chrysotile derived from brake pads, the first aspect of consideration is the effect on lung deposition and internal dose. The somewhat peculiar aerodynamic properties of a fiber allowing their efficient penetration of the lung despite long length (e.g. >20 µm) is well described within the literature (Lippmann Citation1990; Jones Citation1993). However, modification of fiber size, shape and density through binding of brake debris matrix may alter these aerodynamic properties. This may occur through changes in the particle/fiber-hybrid shape leading to altered drag, change in density of the overall structure (thereby increasing the aerodynamic diameter) but also alter the weight distribution of the structure with possible implications for interception in the airways. This hypothesis was discussed by Weir and Meraz who stated that fibers with resin attached would likely have aerodynamic properties considerably different from “clean” free fibers. The attachment or coating of a fiber would likely serve to increase its aerodynamic diameter (Dae), owing to increased density, size, and possible drag, meaning that deposition would occur more efficiently higher up the respiratory tract where particle clearance is more efficient (Asgharian et al. Citation2001). However, the degree to which this significantly affects the aerodynamic characteristics and depositional profile of bound fibers would be very much dependent on the level of matrix binding. As such, the effect of matrix binding on chrysotile Dae should be viewed as a continuum from little to no alteration to significant modification rendering a previously respirable fiber non-respirable. In terms of the former, this may be in the form of a very thin, even surface coating which does not alter the diameter, shape, or density significantly while in the case of the latter, this may be the presence of a large matrix particle as a component of the fiber.
Surface reactivity
The role played by the physicochemical properties of particulates and fibers in driving a pathogenic response has been well recognized since the early research days of particle toxicology. Among the physicochemical properties, those relating to the surface are of particular interest as it is the surface of particles and fibers which interact first hand with the surrounding target tissue and cells following deposition in the organism (Fubini Citation1997; Oberdorster and Graham Citation2018). The biological effects of different forms of the same material, such as silica, as well as chemically modified samples, can be related to the physiochemical properties. Quartz (crystalline silica) is known to have a highly reactive surface which plays an integral part in its toxicity (Donaldson and Borm Citation1998; Duffin et al. Citation2001). Treatment of the quartz surface with aluminum salts, such as aluminum lactate, has been shown to passivate the surface reactivity of the quartz particles. In doing so, treatment significantly reduces their ability to produce harmful free radicals including the induction of molecular signaling events leading to a proinflammatory response (Duffin et al. Citation2001, Citation2002). The differences in surface reactivity among specimens of minerals with the same chemical composition and crystal structure, which determine their different pathogenic potentials, are modified by physicochemical processes taking place at the surface (Fubini Citation1997). Mild grinding can activate “asbestos” through the exposure of ferrous iron; however, prolonged grinding has been shown to profoundly modify and even inactivate chrysotile asbestos (Langer et al. Citation1978; Langer Citation2003). This would suggest that there is a correlation between the reactivity of the asbestos fiber and the potential toward an adverse health effect.
In relation to chrysotile in brake debris, coating of the chrysotile surface with insoluble, low toxicity cured resin could lead to passivation of the highly positive surface charge of the chrysotile as well as presenting a steric barrier between the chrysotile surface and biological environment. However, this hypothesis assumes that the resin matrix, complete with its particulate components (e.g. carbon black, iron, etc.), is in itself, a low toxicity material.
The importance of surface charge on particle toxicity has been demonstrated for various particulates (Cho et al. Citation2012) but has also been shown for chrysotile. To compare the relative potency charged versus discharged chrysotile, Davis et al. (Citation1988) exposed rats via inhalation to UICC chrysotile A for 12 months. One group was treated with dust carrying the normal electrostatic charge while the other was exposed to dust discharged by exposure to ionizing radiation resulting in a reduction in surface charge. After exposure, the animals exposed to chrysotile still possessing its normal charge had significantly more fibers retained in their lungs than animals exposed to discharged dust with greater pulmonary fibrosis and tumor development as a result (Davis et al. Citation1988). This elegant study demonstrates the possible impact alteration in surface charge may have on fiber retention and pathogenicity and therefore coating with brake resin, could serve as a modifier in chrysotile toxicity.
While coating with resin may have a beneficial impact on toxicity by neutralizing surface charge, it may also form a source of increased reactivity depending on the physicochemical characteristics of the resin matrix. This is because the brake matrix is not solely composed of phenolic resin and instead is an amalgamation of various substances including transition metals. These particles are used as to improve the performance and wear characteristics of the brake pads but the presence of such transition metals may act a source of reactivity owing to the relatively low energy gap between different possible oxidation states which can result in oxidative stress and inflammation (Zhang et al. Citation2012). As described earlier, metal particles such as iron oxide are incorporated into brake pads and as such these are distributed throughout the resin matrix, forming a component of particles bound to chrysotile fibers. The impact of iron in mineral fiber toxicity is well described within the literature (Fubini and Mollo Citation1995; Hardy and Aust Citation1995b; Kamp and Weitzman Citation1999; Aust et al. Citation2011) and while crocidolite has the highest level of iron (∼27%), chrysotile also contains iron (∼3–6%) (IARC Citation2012). Redox-active iron, either as an intrinsic component of the fiber or as a bound “contaminant” can contribute to oxidative stress via the formation of superoxide (O2•−), hydrogen peroxide (H2O2), and hydroxyl radical (•OH) (Turi et al. Citation2004). It is persistent oxidative stress, in part driven by iron-mediated ROS production, leading to chronic inflammation, DNA damage, and altered gene expression that is thought to contribute to the injurious effects of “asbestos” fibers (Kamp and Weitzman Citation1999; Benedetti et al. Citation2015). As such, the presence of higher levels of iron on the surface of chrysotile through contamination with iron containing resin matrix may logically result in increased redox-activity of the coated fiber. The impact of modified iron levels mineral fiber toxicity and the generation of MM have been shown across numerous studies employing various techniques to reduce iron such as via the use of chelators (Jiang et al. Citation2016) and even phlebotomy (Ohara et al. Citation2018). However, an elegant study by Gazzano et al. (Citation2007) took a different approach. Here a modified synthetic chrysotile nanofiber, devoid of iron or any other contaminant to study the impact of increasing levels of iron. The fibers without iron did not generate oxidative stress, genotoxicity, or cytotoxicity in vitro within a macrophage cell line. However, the same fibers loaded with 0.57% and 0.94% (w/w) iron were found to induce oxidative damage in the form of DNA strand breaks and lipoperoxidation. A later study by some of the same authors found that even the smallest iron contamination imparts radical reactivity to chrysotile (Turci et al. Citation2011) and so contamination with iron from resin matrix may well increase toxicity.
Despite this, the relative importance of higher levels of surface bound iron to the carcinogenicity of chrysotile fibers over time is uncertain as it is not only intrinsic iron that can contribute to persistent fiber reactivity and oxidative damage. It is well recognized that all forms of “asbestos” have the ability to bind endogenous iron such as ferritin from the biological environment leading to the formation of ferruginous bodies (Fubini et al. Citation1997; Ghio et al. Citation2004). It is thought that this localized concentration of iron though binding to a persistent particulate can lead to metal-catalyzed oxidative stress, DNA damage, and apoptosis resistance (Hardy and Aust Citation1995a; Ghio et al. Citation1997; Pascolo et al. Citation2013). However, for iron to contribute to toxicity it must be bioavailable and redox-active. Within the body, iron (as with many transition metals essential for health) is tightly controlled to limit reactivity. It was noted by Ghio et al. (Citation1997) that while ferruginous bodies formed around crocidolite administered to the lungs of guinea pigs, this did not necessarily result in increased oxidant production. They observed that asbestos bodies showed reduced oxidant production as compared to uncoated fibers which was thought to be due to iron in the form of ferritin not being chemically reactive in oxidant production (Ghio et al. Citation1997; Turi et al. Citation2004). The importance of bioavailability is also relevant to transition metals such as iron within brake debris as it is not simply the presence of iron or other transition metal that leads to generation of ROS. Encased or encapsulated iron that does not have a bioavailable surface would not be redox active and instead, behave in the body simply as a resin particle. It is only once the surface is bioavailable that the particulate iron can contribute to toxicity via ROS production leading to oxidative damage.
It is important to stress that in relation to MM specifically, there is no association between exposure to particulate matter including particulate iron or diesel exhaust particles, a recognized carcinogen (IARC Citation2014) and MM. Therefore, any concern relating to metal mediated toxicity and MM relates purely to the possible modification of chrysotile.
Biodurability
The coating of chrysotile may also have a significant implication for the durability of the fiber in an acidic biological environment such as a macrophage phagolysosome. As previously discussed, magnesium from the brucitic layer of the chrysotile fiber is leached leading to two significant changes in the fiber. The first is a reduction in surface charge as it is the positive magnesium ions that generate the positive surface charge of the fiber and their removal leads to a neutralization of the fiber surface. An insoluble layer preventing leaching would mean the positive surface charge is retained although this may be a moot point as the coating may neutralize the surface. The second and perhaps more important change would be to the overall biopersistence of long fibers. Biopersistence is determined by a particulates resistance to chemical dissolution (biodurability) and mechanical clearance. Chemical dissolution can lead ultimately to the shortening of long fibers thereby enabling their efficient clearance via macrophages. Therefore, a coating of insoluble resin could serve to insulate the rapidly leached brucitic layer as well as the more slowly leached silicate layers from an external low pH environment, preventing leaching and as such, reduce the rate of weakening and fragmentation of long fibers. In effect, a coating could turn relatively low durability chrysotile fibers into durable analogs leading to reduced clearance and increased residence time. It should be noted that this refers explicitly to long fibers as short fibers (i.e. <15 μm in the lung, <5 μm in the pleura, Schinwald et al. Citation2012a, Citation2012b) would be cleared irrespective of chemical durability.
The overall effect on the toxicity of chrysotile fibers held within a matrix then ground creating chrysotile containing dust is difficult to judge although there is indeed the potential for modification. There have however been studies looking at the modification of toxicity of an analogous material, namely carbon nanotubes. Carbon nanotubes are an engineered nanofiber composed of carbon atoms formed into a cylindrical structure with exceptional thermal and electrical conductance properties as well as hardness and tensile strength. They have however come under scrutiny owing to the physicochemical attributes of certain types of carbon nanotube that identified them as a possible pathogenic fiber (i.e. long length, small diameter, and biodurability) (Poland et al. Citation2008). Later studies have shown the potential for long, thin carbon nanotubes to cause MM in wild-type animal models (Rittinghausen et al. Citation2014; Chernova et al. Citation2017) as well as significant lung inflammation and fibrosis (Ma-Hock et al. Citation2013). To determine the release of carbon nanotubes from composites and their toxicological properties, Wohlleben et al. (Citation2011) incorporated carbon nanotubes into the thermoplastic resin polyoxymethylene and cement paste. The composites were ground and the pulmonary toxicity of the dust compared to carbon nanotubes alone as well as the base composites without the addition of carbon nanotubes. Assessing lung lavage parameters for evidence of inflammation three days and three months after installation showed that both the cement and cement + carbon nanotube dusts caused a slight inflammatory response in the lungs as characterized by a neutrophil influx as well as histological changes. Similarly, a limited response was evident after installation of the thermoplastic resin dust with and without carbon nanotubes with (multi)focal granulomatous inflammation of minimal grade noted. In both cases, the response had mostly resolved by the 3-month post exposure period. While limited inflammatory effects were observed after treatment, there was no significant difference between the dusts containing carbon nanotubes and those without. This contrasts greatly with the effects caused by the installation of carbon nanotubes alone which created a prominent neutrophilic inflammation and increase in a variety of inflammatory parameters. Analysis of the released fragments during a range of different abrasion methods (Taber abrader and do-it-yourself sandpaper) showed no release of carbon nanotubes from the thermoplastic resin and surface-structured fragments (hairy layer of carbon nanotubes emerging from the surface), but no free fibers in the case of hand sanded cement. Overall, the study showed no additional hazard potential associated with the inclusion of carbon nanotubes into thermoplastics or cement over and above the expected dust irritation reaction noted for the dusts without carbon nanotubes (Wohlleben et al. Citation2011). A later study using the same type of multi-walled carbon nanotubes (Nanocyl NC7000) also investigated the toxicity of carbon nanotube containing epoxy resin composite dust. Similarly, they found that the dust particles with and without carbon nanotubes caused a brief inflammatory response (dust reaction) yet there were no differences in lung parameters from the carbon nanotube-containing epoxy and the reference epoxy (Saber et al. Citation2016). Interestingly, the authors also examined the hepatic response to particle installation and observed inflammation and necrotic histopathological changes in mice treated with the carbon nanotube-containing epoxy sanding dust but not with the reference epoxy. The reasoning behind this differential effect was considered to be due to slow translocation of carbon nanotubes to the liver and possibly other distal organs as evidenced by granulomas in the liver similar to those observed after carbon nanotube treatment alone and presence of black material in liver macrophages (Saber et al. Citation2016). These findings from both studies are interesting as they suggest that a pathogenic fiber incorporated into a matrix and then released via abrasion does not show the same level of respiratory toxicity as the pristine fiber. It is therefore not merely plausible but likely that chrysotile within a phenolic resin brake matrix would display a different toxicological response as compared to pristine chrysotile and drawing on the experience with carbon nanotubes, this may be drastically reduced. However, as noted in the study by Saber et al. (Citation2016), differential effects may be noted elsewhere in the body.
Therefore, as concluded by Wang et al. (Citation2017) in reviewing the transformation of released asbestos, carbon fibers and carbon nanotubes from composite materials, their toxicity may be significantly altered from the pristine fibers. This, in turn, should be taken into account in the risk assessment of fiber-containing composites.
Other factors influencing the mesotheliomagenic potential of chrysotile containing brake debris
Mesothelioma – inducing potency of chrysotile asbestos
Hand in hand with exposure to a toxicant, including asbestos fibers, is the question of potency. While “asbestos” fibers are regulated the same and all classified as Class 1 carcinogen, ample evidence from both animal and human studies tell us that they are not equally as potent at causing disease, especially MM. It is not the view of the authors that such a group classification is inappropriate or should be altered, such a classification provides suitable and much needed protection. However, when considering specific diseases, the physicochemical properties of a given fiber undoubtedly modify the potency. A meta-analysis by Berman and Crump (Citation2008a) relating fiber size and mineral type to “asbestos”-related cancer risk tested various hypothesize. They found that the hypothesis that chrysotile and amphibole asbestos are equally potent was confidently rejected by every metric analyzed. Furthermore, they were unable to reject by any metric the hypothesis that pure chrysotile is non-potent for MM (Berman and Crump Citation2008a). Overall, they established that the relative potency of chrysotile ranged from zero to about 1/200th of that of amphibole asbestos (depending on metric). They concluded that while the risk from chrysotile is certainly not negligible, there results are consistent with the evidence that chrysotile did not cause a large proportion of MM in the UK. Hodgson and Darnton (Citation2010), placed the risk of MM from chrysotile at least an order of magnitude lower than the risk for amphibole fibers (0.007% per f/mL.year vs. 0.5 for crocidolite, 0.1 for amosite). As summarized by Pierce et al. (Citation2008), a US Environmental Protection Agency group concluded that amphiboles are 800 times more potent than chrysotile in inducing MM (four times in relation to lung cancer). A more modest risk ratio was described by Hodgson and Darnton (Citation2000) of 1:100:500 for chrysotile, amosite, and crocidolite respectively.
The consideration in the relative MM causing potency of chrysotile is important when considering the nature of exposures in vehicle mechanics and propensity for MM. Where exposures are low and intermittent and solely to chrysotile from brake pads, given the relative potency of chrysotile in causing MM, such exposures may be insufficient to lead to an appreciable incidence of MM although this is not necessarily the case for lung cancer. It is also important to consider the general question of “does chrysotile cause mesothelioma” in relation to the context of this review as we are not considering pristine chrysotile. Instead we are considering chrysotile that has been bound in a resin matrix, heated and ground and therefore, subject to possible modification. The general question is still highly relevant but the answer, which is hotly debated, should not be read-across from mined chrysotile to chrysotile in brake debris without first considering the physicochemical properties of the released chrysotile.
Amphibole contamination
A somewhat contentious and hotly debated topic in the risk of MM associated with chrysotile exposure is the so-called “amphibole hypothesis”. Contamination of chrysotile ore with amphiboles such as tremolite, is common and therefore there is the potential for mixed fiber exposure. This is supported by analysis of lung tissue in chrysotile workers which have shown significantly higher proportion of amphibole fibers in MM cases (Roggli et al. Citation2002). In the UK, a study by McDonald et al. (Citation1997) looking at the incidences of MM in Thetford chrysotile miners and millers noted a substantially increased risk associated with years of employment in a group of five mines (area A), but not in a peripherally distributed group of 10 mines (area B). The risk was not affected by the level of dust exposure, leading the authors to suggest mineralogical differences between the two geographical regions was the cause. Lung tissue analyses showed that the concentration of tremolite fibers was much higher in area A than in area B (McDonald et al. Citation1997). Such observations led to the “amphibole hypothesis” which purports that it is the much more durable amphiboles that cause MM in exposed workers rather than the less durable chrysotile (Mossman et al. Citation1990). Considering this hypothesis in light of the BED concept, while there may be a mixed exposure to chrysotile containing a sub-fraction of tremolite, it would be the long, biodurable amphiboles that present a key pro-oncogenic driver and chrysotile to a lesser extent. However, this hypothesis is not universally supported and a key criticism is that despite differing levels of amphibole contamination in different regions, this is not always borne out by differing mortality rates from MM (Stayner et al. Citation1996). In addition, a review of the amphibole hypothesis by Stayner et al. (Citation1996) found that while chrysotile may be less potent than amphiboles for inducing MM, there was little evidence to indicate lower lung cancer risk in the case of “pure” chrysotile exposures.
The higher potency of amphiboles in causing MM suggests that where they are present as a contaminating element, they may well increase the potency of an exposure in generating MM. Conversely, an absence of amphiboles would suggest a reduced potency, thus requiring heavier chrysotile exposure to generate a significant risk of MM. This is not to suggest that amphibole-free chrysotile is in anyway “safe”, but simply that it may present a different toxicity profile as compared to a mixed exposure.
The relevance of the role of the amphibole hypothesis to disease incidence in mechanics hinges very much on the presence of said amphiboles within the brake pads themselves. Although plausible given the common contamination of chrysotile with amphiboles, studies characterizing the released particles have not reported the presence of amphiboles. Furthermore, the majority of reported sampling studies of the occupational environment of mechanics has not described the presence of amphibole although there are exceptions (Kakooei et al. Citation2011). However, as amphiboles comprise a tiny contaminating fraction of some chrysotile deposits and limited release of free fibers from brake pads, the lack of observation is not unlikely. Additionally, taking the above comment that the development of MM in man owing purely to chrysotile exposure requires an extremely large dose, such exposures are not present in mechanics.
Summary and conclusions
The highly emotive and litigious nature of asbestos and asbestos-associated disease is both understandable and just. The extensive use of what was once considered a “wonder material” meant widespread exposure in the occupational as well as the built environment leading to a litany of disease. In the UK, 2595 people died of MM in 2016 (HSE 2018) with a similar number (2597) dying in the US in 2015 which has been relatively consistent year on year (Mazurek et al. Citation2017). These numbers do not represent the sole sum of asbestos associated deaths and certainly not the physical and emotional suffering caused by exposure.
The repair and servicing of brake pads containing asbestos is associated with exposure, although the levels (below or above occupational exposure limits) vary between site and study. Epidemiological and meta-analysis studies, in terms of weight of evidence, show no significant risk of MM in mechanics working with such Chrysotile-continaing friction products. This, it seems, is a disconnect between exposure and response where exposure exceeds current limits yet may well be explained by alteration of the pathogenic properties of chrysotile during encapsulation and release from brake pads. Here we have presented the possible modifications that may occur to chrysotile fibers during this process and the subsequent impact on the toxicity of such particulates. In summary, the attributes of chrysotile and modifications that may occur in relation to brake pads and MM risk are:
Low potency of chrysotile at causing MM - requirement for high fiber dose;
Low release of WHO classifiable fibers from brake pads – short fibers predominate;
Low release of free fibers – fibers often have matrix associated with part of the fiber;
Low biodurability of chrysotile fibers (possibly linked to lower potency);
Possible coating of fibers causing passivation of surface;
Grinding and braking can lead to significant heating of brake pads and heat modification of chrysotile toxicity has been well established;
Infrequent reporting of co-exposure to amphiboles relating to brake pad repair/servicing.
From a toxicological perspective, there is certainly the possibility of a modification of chrysotile toxicity as it pertains to released particles from asbestos containing brake pads. Indeed, as compared to bulk chrysotile exposures, the potential for modification is substantial. However, evidence is required rather than supposition and for that, there is a need for in depth characterization studies of the respirable material released during brake repair and servicing.
The fact of the matter is and remains that particle toxicity is determined by the dose and physicochemical characteristics of a particulate, not its name. Particle toxicology is replete with examples of modification of toxicity owing to alteration of physiochemical properties. As discussed previously, coating of highly reactive quartz with aluminum lactate passivates the surface and high toxicity particulate into a low toxicity particulate by modifying the physicochemical characteristic that underlies its toxicity; its BED. Conversely, alteration of the physicochemical characteristics of a particle can also increase its toxicity. A clear example of this is the effect the formation of low aspect ratio titanium dioxide (TiO2) particles, a low toxicity material, into long fibers. Instillation into the lung caused minimal response in the case of the particulate TiO2 while the long fibers caused inflammation and fibrosis despite being compositionally the same as the low aspect ratio particles (Hamilton et al. Citation2009). In this case, the presence of long fibers rather than the surface properties of TiO2 were the BED causing toxicity. That elegant study demonstrates clearly the power and importance of considering the physicochemical properties of a material, including any modifications arriving from manufacture, use etc., when assessing possible risks. Ironically it is the advent of new fibrous materials that has, to an extent, increased the interest (and funding) in the ability of fibers to cause MM (Service Citation1998; The Royal Society, Royal Academy of Engineering Citation2004; Poland et al. Citation2008) despite the fact that asbestos derived MM is an ever-present problem on the increase.
When considering chrysotile in brake pads, it is not just possible that alteration of the physiochemical parameters could be modified by the production/use but highly unlikely that it would not. The combination of being encased in resin under pressure and heat to form the brake pads and then being released under high sheer stress and heat generated by a grinding surface certainly provides extreme environmental conditions whereby coating, dihydroxylation under heat, fragmentation, etc. could occur.
Historical data from case and cohort studies, by its very nature, make it difficult to conduct meaningful new analysis; not least where information on exposure characteristics is lacking (i.e. physicochemical properties of released particles, exposure levels and durations). Indeed, the current literature is replete with claim and counter claim surrounding such analysis and re-analysis (Finley et al. Citation2012, Citation2013; Finkelstein Citation2013) which further serves to obfuscate rather than clarify. However, physicochemical analysis of released debris and toxicological studies making use of our improved understanding of the mechanisms leading to MM such as gene silencing and deletions (Chernova et al. Citation2017) offer a testable way forward. As argued by Welch (Citation2007), there is a need to consider all avenues of evidence to draw a scientifically reasoned conclusions surrounding possible links between brake debris exposure and MM. It is proposed that a more critical evaluations of the particulates released from asbestos-containing brake pads are needed with a significant focus on the physicochemical characteristics of the respirable component of the dust.
Declaration of interest
Concept Life Sciences and IOM Consulting undertake independent consulting activities for private companies, regulators, and government agencies. This work was funded by Honeywell International Inc. and Ford Motor Company. The sponsors and their representatives’ roles were limited to providing study funding; they have had no input into the development of the paper, nor have the sponsors or any of their in-house or outside legal counsel reviewed the paper prior to publication. The authors have not appeared in any regulatory or legal proceedings related to the content of this paper. The content and conclusions presented are the professional work of the authors and as such, may not represent the views of the sponsor or their employees. Honeywell and Ford are defendants in asbestos-product litigation involving automotive brakes.
Acknowledgements
The authors wish to acknowledge feedback and discussions from Dr Matthew Boyles, Institute of Occupational Medicine and funding from Honeywell International Inc. and Ford Motor Company. We would also like to gratefully acknowledge the time and efforts of the reviewers selected by the Editor and their valuable comments which, as ever, promote balanced scientific discussion.
References
- [ACGIH] ACoGIH. 2005. TLVs and BEIs: based on the documentation of the threshold limit values for chemical substances and physical agents and biological exposure indices. Cincinnati (OH): ACGIH.
- [CEN] ECfS. 1993. Workplace atmospheres-size fraction definitions for measurement of airborne particles. London, England: CEN, British Standards Institute.
- Agudo A, González CA, Bleda MJ, Ramírez J, Hernández S, López F, Calleja A, Panadès R, Turuguet D, Escolar A, et al. 2000. Occupation and risk of malignant pleural mesothelioma: a case-control study in Spain. Am J Ind Med. 37:159–168.
- AIA. 1984. Method for the determination of airborne asbestos fibres and other inorganic fibres by scanning electron microscopy. Health and Safety Publ RTM No. 2. London: AIA.
- Ameille J, Rosenberg N, Matrat M, Descatha A, Mompoint D, Hamzi L, Atassi C, Vasile M, Garnier R, Pairon JC. 2012. Asbestos-related diseases in automobile mechanics. Ann Occup Hyg. 56:55–60.
- Asgharian B, Hofmann W, Miller FJ. 2001. Mucociliary clearance of insoluble particles from the tracheobronchial airways of the human lung. J Aerosol Sci. 32:817–832.
- Atkinson MA, O'Sullivan M, Zuber S, Dodson RF. 2004. Evaluation of the size and type of free particulates collected from unused asbestos-containing brake components as related to potential for respirability. Am J Ind Med. 46:545–553.
- Aust AE, Cook PM, Dodson RF. 2011. Morphological and chemical mechanisms of elongated mineral particle toxicities. J Toxicol Environ Health B Crit Rev. 14:40–75.
- Baron P. 2017. Chapter FI, measurement of fibers. In: Ashley K, O’Connor PF, editors. NIOSH manual of analytical methods (NMAM). 5th ed. Washington (DC): Centers for Disease Control and Prevention.
- Belhocine A, Bouchetara M. 2012. Thermal analysis of a solid brake disc. Appl Therm Eng. 32:59–67.
- Bellmann B, Konig H, Muhle H, Pott F. 1986. Chemical durability of asbestos and of man-made mineral fibers in vivo. J Aerosol Sci. 17:341–345.
- Benedetti S, Nuvoli B, Catalani S, Galati R. 2015. Reactive oxygen species a double-edged sword for mesothelioma. Oncotarget. 6:16848–16865.
- Berman DW, Crump KS. 2008a. A meta-analysis of asbestos-related cancer risk that addresses fiber size and mineral type. Crit Rev Toxicol. 38:49–73.
- Berman DW, Crump KS. 2008b. Update of potency factors for asbestos-related lung cancer and mesothelioma. Crit Rev Toxicol. 38:1–47.
- Bernstein D, Rogers R, Smith P. 2005. The biopersistence of Canadian chrysotile asbestos following inhalation: final results through 1 year after cessation of exposure. Inhal Toxicol. 17:1–14.
- Bernstein DM, Chevalier J, Smith P. 2005. Comparison of Calidria chrysotile asbestos to pure tremolite: final results of the inhalation biopersistence and histopathology examination following short-term exposure. Inhal Toxicol. 17:427–449.
- Bernstein DM, Morscheidt C, Grimm H-G, Thévenaz P, Teichert U. 1996. Evaluation of soluble fibers using the inhalation biopersistence model, a nine-fiber comparison. Inhal Toxicol. 8:345–385.
- Bernstein DM, & Riego-Sintes JM. 1999. Methods for the Determination of the Hazardous Properties for Human Health of Man Made Mineral Fibres (MMMF) (Report No. EUR 18748), Brussels, European Commission Joint Research Centre, European Chemicals Bureau.
- Bernstein DM, Rogers R, Smith P. 2004. The biopersistence of Brazilian chrysotile asbestos following inhalation. Inhal Toxicol. 16:745–761.
- Bernstein DM, Rogers RA, Sepulveda R, Kunzendorf P, Bellmann B, Ernst H, Creutzenberg O, Phillips JI. 2015. Evaluation of the fate and pathological response in the lung and pleura of brake dust alone and in combination with added chrysotile compared to crocidolite asbestos following short-term inhalation exposure. Toxicol Appl Pharmacol. 283:20–34.
- Berry G, Newhouse ML. 1983. Mortality of workers manufacturing friction materials using asbestos. Br J Ind Med. 40:1–7.
- Blake CL, Van Orden DR, Banasik M, Harbison RD. 2003. Airborne asbestos concentration from brake changing does not exceed permissible exposure limit. Regul Toxicol Pharmacol: RTP. 38:58–70.
- Boillat MA, Lob M. 1973. Risk of asbestosis in workers employed in replacing automobile brake linings. Schweiz Med Wochenschr. 103:1354–1359.
- Brown J, Gordon T, Price O, Asgharian B. 2013. Thoracic and respirable particle definitions for human health risk assessment. Part Fibre Toxicol. 10:12.
- Bruch J, Rehn S, Rehn B, Borm PJ, Fubini B. 2004. Variation of biological responses to different respirable quartz flours determined by a vector model. Int J Hyg Environ Health. 207:203–216.
- Cattaneo A, Gualtieri AF, Artioli G. 2003. Kinetic study of the dehydroxylation of chrysotile asbestos with temperature by in situ XRPD. Phys Chem Miner. 30:177–183.
- Cely-Garcia MF, Sanchez M, Breysse PN, Ramos-Bonilla JP. 2012. Personal exposures to asbestos fibers during brake maintenance of passenger vehicles. Ann Occup Hyg. 56:985–999.
- Cely-Garcia MF, Torres-Duque CA, Duran M, Parada P, Sarmiento OL, Breysse PN, Ramos-Bonilla JP. 2015. Personal exposure to asbestos and respiratory health of heavy vehicle brake mechanics. J Expos Sci Environ Epidemiol. 25:26–36.
- Chernova T, Murphy FA, Galavotti S, Sun XM, Powley IR, Grosso S, Schinwald A, Zacarias-Cabeza J, Dudek KM, Dinsdale D, et al. 2017. Long-fiber carbon nanotubes replicate asbestos-induced mesothelioma with disruption of the tumor suppressor gene Cdkn2a (Ink4a/Arf). Curr Biol. 27:3302–3314.e6.
- Cherrie JW, Brosseau LM, Hay A, Donaldson K. 2013. Low-toxicity dusts: current exposure guidelines are not sufficiently protective. Ann Occup Hyg. 57:685–691.
- Cho WS, Duffin R, Thielbeer F, Bradley M, Megson IL, Macnee W, Poland CA, Tran CL, Donaldson K. 2012. Zeta potential and solubility to toxic ions as mechanisms of lung inflammation caused by metal/metal-oxide nanoparticles. Toxicol Sci. 126:469–477.
- Churg A, Stevens B. 1995. Enhanced retention of asbestos fibers in the airways of human smokers. Am J Respir Crit Care Med. 151:1409–1413.
- Churg A, Wright JL. 1994. Persistence of natural mineral fibers in human lungs: an overview. Environ Health Perspect. 102:229–233.
- Churg A. 2003. Interactions of exogenous or evoked agents and particles: the role of reactive oxygen species. Free Radic Biol Med. 34:1230–1235.
- Clin B, Paris C, Ameille J, Brochard P, Conso F, Gislard A, Laurent F, Letourneux M, Luc A, Schorle E, et al. 2011. Do asbestos-related pleural plaques on HRCT scans cause restrictive impairment in the absence of pulmonary fibrosis? Thorax. 66:985–991.
- Clouter A, Brown D, Hohr D, Borm P, Donaldson K. 2001. Inflammatory effects of respirable quartz collected in workplaces versus standard DQ12 quartz: particle surface correlates. Toxicol Sci. 63:90–98.
- Coggon D, Inskip H, Winter P, Pannett B. 1995. Differences in occupational mortality from pleural cancer, peritoneal cancer, and asbestosis. Occup Environ Med. 52:775–777.
- Coin PG, Roggli VL, Brody AR. 1992. Deposition, clearance, and translocation of chrysotile asbestos from peripheral and central regions of the rat lung. Environ Res. 58:97–116.
- Coin PG, Roggli VL, Brody AR. 1994. Persistence of long, thin chrysotile asbestos fibers in the lungs of rats. Environ Health Perspect. 102:197–199.
- HSE. 2018. Asbestos-related diseases in Great Britain, 2018. HSE Books, Sadbury, Suffolk.
- Davis J, Addison J, Bolton R, Donaldson K, Jones A, Smith T. 1986. The pathogenicity of long versus short fibre samples of amosite asbestos administered to rats by inhalation and intraperitoneal injection. Br J Exp Pathol. 67:415–430.
- Davis JM, Bolton RE, Douglas AN, Jones AD, Smith T. 1988. Effects of electrostatic charge on the pathogenicity of chrysotile asbestos. Br J Ind Med. 45:292–299.
- Davis JM, Jones AD. 1988. Comparisons of the pathogenicity of long and short fibres of chrysotile asbestos in rats. Br J Exp Pathol. 69:717–737.
- Davis JMG, Coniam SW. 1973. Experimental studies on the effects of heated chrysotile asbestos and automobile brake lining dust injected into the body cavities of mice. Exp Mol Pathol. 19:339–353.
- Deane L. 1899. Report on the health of workers in asbestos and other dusty trades. London: HMSO.
- Donaldson K, Aitken R, Tran L, Stone V, Duffin R, Forrest G, Alexander A. 2006. Carbon nanotubes: a review of their properties in relation to pulmonary toxicology and workplace safety. Toxicol Sci. 92:5–22.
- Donaldson K, Borm PJ. 1998. The quartz hazard: a variable entity. Ann Occup Hyg. 42:287–294.
- Donaldson K, Murphy F, Duffin R, Poland C. 2010. Asbestos, carbon nanotubes and the pleural mesothelium: a review of the hypothesis regarding the role of long fibre retention in the parietal pleura, inflammation and mesothelioma. Part Fibre Toxicol. 7:5.
- Donaldson K, Schinwald A, Murphy F, Cho WS, Duffin R, Tran L, Poland C. 2012. The biologically effective dose in inhalation nanotoxicology. Acc Chem Res.
- Donaldson K, Tran CL. 2004. An introduction to the short-term toxicology of respirable industrial fibres. Mutat Res. 553(1–2):5–9.
- Duffin R, Clouter A, Brown DM, Tran CL, MacNee W, Stone V, Donaldson K. 2002. The importance of surface area and specific reactivity in the acute pulmonary inflammatory response to particles. Ann Occup Hyg. 46:242–245.
- Duffin R, Gilmour PS, Schins RP, Clouter A, Guy K, Brown DM, MacNee W, Borm PJ, Donaldson K, Stone V. 2001. Aluminium lactate treatment of DQ12 quartz inhibits its ability to cause inflammation, chemokine expression, and nuclear factor-kappaB activation. Toxicol Appl Pharmacol. 176:10–17.
- EC. 2008. Regulation 1272/2008 of the European Parliament and of the Council of 16 December 2008 on classification, labelling and packaging of substances and mixtures, amending and repealing Directive 67/548/EEC and 1999/45/EC and amending Regulation (EC) No 1907/2006. Off J Eur Union. 51:1–1357.
- Egilman DS, Billings MA. 2005. Abuse of epidemiology: automobile manufacturers manufacture a defense to asbestos liability. Int J Occup Environ Health. 11:360–371.
- Eikermann M, Houle TT. 2016. Antagonism of neuromuscular block: all things are poison; only the dose makes a thing not a poison. Br J Anaesth. 116:157–159.
- EPA. 2018. U.S. federal bans on asbestos. Washington D.C. USA: EPA; [accessed 2018 Apr 3]. https://www.epa.gov/asbestos/us-federal-bans-asbestos.
- Erdinc M, Erdinc E, Cok G, Polatli M. 2003. Respiratory impairment due to asbestos exposure in brake-lining workers. Environ Res. 91:151–156.
- Ferrer J, Garcia-Valero J, Montes JF. 2008. Chapter 10, pleural reaction to mineral dusts. In: Light RW, Lee YCG, editors. Textbook of pleural diseases. 2nd ed. Boca Raton, FL: CRC Press; p. 11.
- Ferrer J, Orriols R, Tura JM, Lirola J, Xaus C, Vidal X. 1994. Energy-dispersive X-ray analysis and scanning electron microscopy of pleura. Study of reference, exposed non-pneumoconiotic, and silicotic populations. Am J Respir Crit Care Med. 149(Pt 1):888–892.
- Finkelstein MM. 2013. Letter concerning the paper by Finley and colleagues: dx.doi.org/10.1016/j.yrtph.2012.05.015. Regul Toxicol Pharmacol. 65:178–179.
- Finkelstein MM. 2015. Asbestos fibres in the lungs of an American mechanic who drilled, riveted, and ground brake linings: a case report and discussion. Ann Occup Hyg. 59:525–527.
- Finley BL, Pierce JS, Paustenbach DJ, Galbraith DA. 2013. Response to a letter to the Editor by Dr. Murray M. Finkelstein regarding the article by Finley et al. (2012). Regul Toxicol Pharmacol. 65:180–181.
- Finley BL, Pierce JS, Paustenbach DJ, Scott LL, Lievense L, Scott PK, Galbraith DA. 2012. Malignant pleural mesothelioma in US automotive mechanics: reported vs expected number of cases from 1975 to 2007. Regul Toxicol Pharmacol: RTP. 64:104–116.
- Finley BL, Richter RO, Mowat FS, Mlynarek S, Paustenbach DJ, Warmerdam JM, Sheehan PJ. 2007. Cumulative asbestos exposure for US automobile mechanics involved in brake repair (circa 1950s–2000). J Expo Sci Environ Epidemiol. 17:644–655.
- Fisher GL, Mossman BT, McFarland AR, Hart RW. 1987. A possible mechanism of chrysotile asbestos toxicity. Drug Chem Toxicol. 10:109–131.
- Fubini B, Barcelo F, Arean CO. 1997. Ferritin adsorption on amosite fibers: possible implications in the formation and toxicity of asbestos bodies. J Toxicol Environ Health. 52:343–352.
- Fubini B, Mollo L. 1995. Role of iron in the reactivity of mineral fibers. Toxicol Lett. 82–83:951–960.
- Fubini B. 1997. Surface reactivity in the pathogenic response to particulates. Environ Health Perspect. 105:1013–1020.
- Ganguly A, George R. 2008. Asbestos free friction composition for brake linings. Bull Mater Sci. 31:19–22.
- Garabrant DH, Alexander DD, Miller PE, Fryzek JP, Boffetta P, Teta MJ, Hessel PA, Craven VA, Kelsh MA, Goodman M. 2016a. Mesothelioma among motor vehicle mechanics: an updated review and meta-analysis. Ann Occup Hyg. 60:8–26.
- Garabrant DH, Alexander DD, Miller PE, Fryzek JP, Boffetta P, Teta MJ, Hessel PA, Craven VA, Kelsh MA, Goodman M. 2016b. Response to Kay Teschke. Re: mesothelioma among motor vehicle mechanics: an updated review and meta-analysis. Ann Hyg. 60:1036–1037.
- Gazzano E, Turci F, Foresti E, Putzu MG, Aldieri E, Silvagno F, Lesci IG, Tomatis M, Riganti C, Romano C, et al. 2007. Iron-loaded synthetic chrysotile: a new model solid for studying the role of iron in asbestos toxicity. Chem Res Toxicol. 20:380–387.
- Ghio AJ, Churg A, Roggli VL. 2004. Ferruginous bodies: implications in the mechanism of fiber and particle toxicity. Toxicol Pathol. 32:643–649.
- Ghio AJ, LeFurgey A, Roggli VL. 1997. In vivo accumulation of iron on crocidolite is associated with decrements in oxidant generation by the fiber. J Toxicol Environ Health. 50:125–142.
- Giantomassi F, Gualtieri AF, Santarelli L, Tomasetti M, Lusvardi G, Lucarini G, Governa M, Pugnaloni A. 2010. Biological effects and comparative cytotoxicity of thermal transformed asbestos-containing materials in a human alveolar epithelial cell line. Toxicol In Vitro. 24:1521–1531.
- Gilham C, Rake C, Burdett G, Nicholson AG, Davison L, Franchini A, Carpenter J, Hodgson J, Darnton A, Peto J. 2016. Pleural mesothelioma and lung cancer risks in relation to occupational history and asbestos lung burden. Occup Environ Med. 73:290–299.
- Goodman M, Teta MJ, Hessel PA, Garabrant DH, Craven VA, Scrafford CG, Kelsh MA. 2004. Mesothelioma and lung cancer among motor vehicle mechanics: a meta-analysis. Ann Occup Hyg. 48:309–326.
- Greim H, Utell MJ, Maxim LD, Niebo R. 2014. Perspectives on refractory ceramic fiber (RCF) carcinogenicity: comparisons with other fibers. Inhal Toxicol. 26:789–810.
- Grigoratos T, Martini G. 2015. Brake wear particle emissions: a review. Environ Sci Pollut Res. 22:2491–2504.
- Gualtieri AF, Pollastri S, Bursi Gandolfi N, Gualtieri ML. 2018. In vitro acellular dissolution of mineral fibres: a comparative study. Sci Rep. 8:7071.
- Gualtieri AF, Tartaglia A. 2000. Thermal decomposition of asbestos and recycling in traditional ceramics. J Eur Ceram Soc. 20:1409–1418.
- Gualtieri AF, Viani A, Sgarbi G, Lusvardi G. 2012. In vitro biodurability of the product of thermal transformation of cement-asbestos. J Hazard Mater. 205–206:63–71.
- Gustavsson P, Plato N, Lidstrom EB, Hogstedt C. 1990. Lung cancer and exposure to diesel exhaust among bus garage workers. Scand J Work Environ Health. 16:348–354.
- Hamilton RF, Wu N, Porter D, Buford M, Wolfarth M, Holian A. 2009. Particle length-dependent titanium dioxide nanomaterials toxicity and bioactivity. Part Fibre Toxicol. 6:35.
- Hansen ES. 1989. Mortality of auto mechanics. A ten-year follow-up. Scand J Work Environ Health. 15:43–46.
- Hansen J, Meersohn A. 2003. Kraeftsygelighed blandt danske lonmodtagere (1970–97) fordelt pa Arbejdstilsynets 49 branchegrupper. Kobenhavn: Institut for Epidemiologisk Kraeftforskning, Kraeftens Bekaempelse.
- Hardy JA, Aust AE. 1995a. Iron in asbestos chemistry and carcinogenicity. Chem Rev. 95:97–118.
- Hardy JA, Aust AE. 1995b. The effect of iron binding on the ability of crocidolite asbestos to catalyze DNA single-strand breaks. Carcinogenesis. 16:319–325.
- Hargreaves A, Taylor WH. 1946. An X-ray examination of decomposition products of chrysotile (asbestos) and serpentine. Miner Mag. 27:204–216.
- Harries PG. 1968. Asbestos hazards in naval dockyards. Ann Occup Hyg. 11:135–145.
- Hatch D. 1970. Possible alternatives to asbestos as a friction material. Ann Occup Hyg. 13:25–29.
- Hein MJ, Stayner LT, Lehman E, Dement JM. 2007. Follow-up study of chrysotile textile workers: cohort mortality and exposure–response. Occup Environ Med. 64:616–625.
- Henderson DW, Rödelsperger K, Woitowitz H-J, Leigh J. 2004. After Helsinki: a multidisciplinary review of the relationship between asbestos exposure and lung cancer, with emphasis on studies published during 1997–2004. Pathology. 36:517–550.
- Hessel PA, Teta MJ, Goodman M, Lau E. 2004. Mesothelioma among brake mechanics: an expanded analysis of a case-control study. Risk Anal. 24:547–552.
- Hesterberg TW, Anderson R, Bernstein DM, Bunn WB, Chase GA, Jankousky AL, Marsh GM, McClellan RO. 2012. Product stewardship and science: safe manufacture and use of fiber glass. Regul Toxicol Pharmacol: RTP. 62:257–277.
- Hesterberg TW, Chase G, Axten C, Miller WC, Musselman RP, Kamstrup O, Hadley J, Morscheidt C, Bernstein DM, Thevenaz P. 1998. Biopersistence of synthetic vitreous fibers and amosite asbestos in the rat lung following inhalation. Toxicol Appl Pharmacol. 151:262–275.
- Hesterberg TW, Hart GA, Chevalier J, Miiller WC, Hamilton RD, Bauer J, Thevenaz P. 1998. The importance of fiber biopersistence and lung dose in determining the chronic inhalation effects of X607, RCF1, and chrysotile asbestos in rats. Toxicol Appl Pharmacol. 153:68–82.
- Hodgson AA. 1979. Chemistry and physics of asbestos. In: Michaels L, Chissick SS, editors. Asbestos: properties, applications, and hazards. Hoboken, NJ: John Wiley & Sons; p. 67–114.
- Hodgson JT, Darnton A. 2000. The quantitative risks of mesothelioma and lung cancer in relation to asbestos exposure. Ann Occup Hyg. 44:565–601.
- Hodgson JT, Darnton A. 2010. Mesothelioma risk from chrysotile. Occup Environ Med. 67:432.
- HSE. 2005. Asbestos: the analysts’ guide for sampling, analysis and clearance procedures. Sudbury, Suffolk: HSE Books.
- HSE. 2009. Health and Safety in Motor Vehicle Repair and Associated Industries, HSG261 HSE Books, Sadbury, Suffolk.
- Huncharek M, Muscat J, Capotorto JV. 1989. Pleural mesothelioma in a brake mechanic. Br J Ind Med. 46:69–71.
- IARC. 1997. IARC monographs on the evaluation of carcinogenic risks to humans. Volume 68. Silica, some silicates, coal dust and para-aramid fibrils.
- IARC. 1977. IARC monographs on the evaluation of carcinogenic risks to humans. Volume 14: Asbestos. Lyon: IARC.
- IARC. 2012. IARC monographs on the evaluation of carcinogenic risks to humans. Volume 100C: arsenic, metals, fibres and dusts. Lyon: IARC.
- IARC. 2014. IARC monographs on the evaluation of carcinogenic risks to humans. Volume 105: diesel and gasoline engine exhausts and some nitroarenes. Lyon: IARC.
- Jarvholm B, Brisman J. 1988. Asbestos associated tumours in car mechanics. Br J Ind Med. 45:645–646.
- Jiang L, Chew SH, Nakamura K, Ohara Y, Akatsuka S, Toyokuni S. 2016. Dual preventive benefits of iron elimination by desferal in asbestos-induced mesothelial carcinogenesis. Cancer Sci. 107:908–915.
- Jones AD. 1993. Respirable industrial fibres: deposition, clearance and dissolution in animal models. Ann Occup Hyg. 37:211–226.
- Kakooei H, Hormozy M, Marioryad H. 2011. Evaluation of asbestos exposure during brake repair and replacement. Ind Health. 49:374–380.
- Kamp DW, Weitzman SA. 1999. The molecular basis of asbestos induced lung injury. Thorax. 54:638–652.
- Kelsh MA, Craven VA, Teta MJ, Mowat FS, Goodman M. 2007. Mesothelioma in vehicle mechanics: is the risk different for Australians? Occup Med (Lond). 57:581–589.
- Kerper LE, Lynch HN, Zu K, Tao G, Utell MJ, Goodman JE. 2015. Systematic review of pleural plaques and lung function. Inhal Toxicol. 27:15–44.
- Kimizuka G, Wang NS, Hayashi Y. 1987. Physical and microchemical alterations of chrysotile and amosite asbestos in the hamster lung. J Toxicol Environ Health. 21:251–264.
- Koshi K, Hayashi H, Sakabe H. 1969. Biological and mineralogical studies on serpentine minerals in heat treated state. Ind Health. 7:66–85.
- Laden F, Stampfer MJ, Walker AM. 2004. Lung cancer and mesothelioma among male automobile mechanics: a review. Rev Environ Health. 19:39–61.
- Langer AM, McCaughey WT. 1982. Mesothelioma in a brake repair worker. Lancet. 2:1101–1103.
- Langer AM, Wolff MS, Rohl AN, Selikoff IJ. 1978. Variation of properties of chrysotile asbestos subjected to milling. J Toxicol Environ Health. 4:173–188.
- Langer AM. 2003. Reduction of the biological potential of chrysotile asbestos arising from conditions of service on brake pads. Regul Toxicol Pharmacol. 38:71–77.
- Lanphear BP, Buncher CR. 1992. Latent period for malignant mesothelioma of occupational origin. J Occup Med. 34:718–721.
- Lemen RA. 2004. Asbestos in brakes: exposure and risk of disease. Am J Ind Med. 45:229–237.
- Lentz TJ, Rice CH, Succop PA, Lockey JE, Dement JM, LeMasters GK. 2003. Pulmonary deposition modeling with airborne fiber exposure data: a study of workers manufacturing refractory ceramic fibers. Appl Occup Environ Hyg. 18:278–288.
- Lippmann M. 1990. Effects of fiber characteristics on lung deposition, retention, and disease. Environ Health Perspect. 88:311–317.
- Loomis D, Dement J, Richardson D, Wolf S. 2010. Asbestos fibre dimensions and lung cancer mortality among workers exposed to chrysotile. Occup Environ Med. 67:580–584.
- Lynch JR. 1968. Brake lining decomposition products. J Air Pollut Control Assoc. 18:824–826.
- Madl AK, Gaffney SH, Balzer JL, Paustenbach DJ. 2009. Airborne asbestos concentrations associated with heavy equipment brake removal. Ann Occup Hyg. 53:839–857.
- Madl AK, Scott LL, Murbach DM, Fehling KA, Finley BL, Paustenbach DJ. 2008. Exposure to chrysotile asbestos associated with unpacking and repacking boxes of automobile brake pads and shoes. Ann Occup Hyg. 52:463–479.
- Ma-Hock L, Strauss V, Treumann S, Kuttler K, Wohlleben W, Hofmann T, Groters S, Wiench K, van Ravenzwaay B, Landsiedel R. 2013. Comparative inhalation toxicity of multi-wall carbon nanotubes, graphene, graphite nanoplatelets and low surface carbon black. Part Fibre Toxicol. 10:23.
- Maluf O, Angeloni M, Milan MT, Spinelli D, Bose Filho WW. 2006. Development of materials for automotive disc brakes. Minerva. 4:149–158.
- Marcus K, Jarvholm BG, Larsson S. 1987. Asbestos-associated lung effects in car mechanics. Scand J Work Environ Health. 13:252–254.
- Marinaccio A, Binazzi A, Cauzillo G, Cavone D, Zotti RD, Ferrante P, Gennaro V, Gorini G, Menegozzo M, Mensi C, et al. 2007. Analysis of latency time and its determinants in asbestos related malignant mesothelioma cases of the Italian register. Eur J Cancer (Oxford, England: 1990). 43:2722–2728.
- Maxim LD, Niebo R, Utell MJ. 2015. Are pleural plaques an appropriate endpoint for risk analyses? Inhal Toxicol. 27:321–334.
- Mazurek J, Syamlal G, Wood J, Hendricks S, Weston A. 2017. Malignant mesothelioma mortality – United States, 1999–2015. MMWR Morb Mortal Wkly Rep. 66:214–218.
- McDonald AD, Case BW, Churg A, Dufresne A, Gibbs GW, Sebastien P, McDonald JC. 1997. Mesothelioma in Quebec chrysotile miners and millers: epidemiology and aetiology. Ann Occup Hyg. 41:707–719.
- McDonald AD, McDonald JC. 1980. Malignant mesothelioma in North America. Cancer. 46:1650–1656.
- McElvenny DM, Darnton AJ, Price MJ, Hodgson JT. 2005. Mesothelioma mortality in Great Britain from 1968 to 2001. Occup Med (Lond). 55:79–87.
- Meisenkothen C. 2017. Malignant mesothelioma in a motor vehicle mechanic: case report and review of the literature. New Solut. 26:524–542.
- Mitchev K, Dumortier P, De VP. 2002. 'Black Spots' and hyaline pleural plaques on the parietal pleura of 150 urban necropsy cases. Am J Surg Pathol. 26:1198–1206.
- Morgan A, Holmes A, Gold C. 1971. Studies of the solubility of constituents of chrysotile asbestos in vivo using radioactive tracer techniques. Environ Res. 4:558–570.
- Mossman B, Lippmann M, Hesterberg T, Kelsey K, Barchowsky A, Bonner J. 2011. Pulmonary endpoints (lung carcinomas and asbestosis) following inhalation exposure to asbestos. J Toxicol Environ Health B Crit Rev. 14:76–121.
- Mossman BT, Bignon J, Corn M, Seaton A, Gee JB. 1990. Asbestos: scientific developments and implications for public policy. Science (New York, NY). 247:294–301.
- Mowat F, Bono M, Lee RJ, Tamburello S, Paustenbach D. 2005. Occupational exposure to airborne asbestos from phenolic molding material (Bakelite) during sanding, drilling, and related activities. J Occup Environ Hyg. 2:497–507.
- Muller J, Delos M, Panin N, Rabolli V, Huaux F, Lison D. 2009. Absence of carcinogenic response to multiwall carbon nanotubes in a 2-year bioassay in the peritoneal cavity of the rat. Toxicol Sci. 110:442–448.
- Muller KM, Schmitz I, Konstantinidis K. 2002. Black spots of the parietal pleura: morphology and formal pathogenesis. Respiration. 69:261–267.
- Murphy FA, Poland CA, Duffin R, Al-Jamal KT, Ali-Boucetta H, Nunes A, Byrne F, Prina-Mello A, Volkov Y, Li S, et al. 2011. Length-dependent retention of carbon nanotubes in the pleural space of mice initiates sustained inflammation and progressive fibrosis on the parietal pleura. Am J Pathol. 178:2587–2600.
- Musk B, Gordon L, Alfonso H, Reid A, Olsen N, Mina R, Franklin P, Peters S, Brims F, Hui J. 2017. Risk factors for malignant mesothelioma in people with no known exposure to asbestos. Am J Ind Med. 60:432–436.
- NIOSH. 1994. Asbestos by TEM: method 7402. Method no.
- NOHSC. 2005. Guidance note on the membrane filter method for estimating airborne fibres. NOHSC: Canberra; p. 3003.
- Oberdorster G, Graham U. 2018. Predicting EMP hazard: lessons from studies with inhaled fibrous and non-fibrous nano- and micro-particles. Toxicol Appl Pharmacol. 361:50–61.
- Ohara Y, Chew SH, Shibata T, Okazaki Y, Yamashita K, Toyokuni S. 2018. Phlebotomy as a preventive measure for crocidolite-induced mesothelioma in male rats. Cancer Sci. 109:330–339.
- Olsen JH, Jensen OM. 1987. Occupation and risk of cancer in Denmark. An analysis of 93,810 cancer cases, 1970–1979. Scand J Work Environ Health. 13 (Suppl.1):1–91.
- Ostermeyer GP, Müller M. 2008. New insights into the tribology of brake systems. Proc Inst Mech Eng D: J Automob Eng. 222:1167–1200.
- Pan XL, Day HW, Wang W, Beckett LA, Schenker MB. 2005. Residential proximity to naturally occurring asbestos and mesothelioma risk in California. Am J Respir Crit Care Med. 172:1019–1025.
- Panahi D, Kakooei H, Marioryad H, Mehrdad R, Golhosseini M. 2011. Evaluation of exposure to the airborne asbestos in an asbestos cement sheet manufacturing industry in Iran. Environ Monit Assess. 178:449–454.
- Pascolo L, Gianoncelli A, Schneider G, Salomé M, Schneider M, Calligaro C, Kiskinova M, Melato M, Rizzardi C. 2013. The interaction of asbestos and iron in lung tissue revealed by synchrotron-based scanning X-ray microscopy. Sci Rep. 3:1123.
- Paustenbach DJ, Finley BL, Lu ET, Brorby GP, Sheehan PJ. 2004. Environmental and occupational health hazards associated with the presence of asbestos in brake linings and pads (1900 to present): a "state-of-the-art" review. J Toxicol Environ Health B Crit Rev. 7:25–80.
- Paustenbach DJ, Richter RO, Finley BL, Sheehan PJ. 2003. An evaluation of the historical exposures of mechanics to asbestos in brake dust. Appl Occup Environ Hyg. 18:786–804.
- Peeters PM, Eurlings IM, Perkins TN, Wouters EF, Schins RP, Borm PJ, Drommer W, Reynaert NL, Albrecht C. 2014. Silica-induced NLRP3 inflammasome activation in vitro and in rat lungs. Part Fibre Toxicol. 11:58.
- Pierce JS, McKinley MA, Paustenbach DJ, Finley BL. 2008. An evaluation of reported no-effect chrysotile asbestos exposures for lung cancer and mesothelioma. Crit Rev Toxicol. 38:191–214.
- Pierce JS, Ruestow PS, Finley BL. 2016. An updated evaluation of reported no-observed adverse effect levels for chrysotile asbestos for lung cancer and mesothelioma. Crit Rev Toxicol. 46:561–586.
- Plato N, Tornling G, Hogstedt C, Krantz S. 1995. An index of past asbestos exposure as applied to car and bus mechanics. Ann Occup Hyg. 39:441–454.
- Poland CA, Byrne F, Cho W-S, Prina-Mello A, Murphy FA, Davies GL, Coey JMD, Gounko Y, Duffin R, Volkov Y, et al. 2012. Length-dependent pathogenic effects of nickel nanowires in the lungs and the peritoneal cavity. Nanotoxicology. 6:899–911.
- Poland CA, Duffin R, Kinloch I, Maynard A, Wallace WAH, Seaton A, Stone V, Brown S, MacNee W, Donaldson K. 2008. Carbon nanotubes introduced into the abdominal cavity of mice show asbestos-like pathogenicity in a pilot study. Nature Nanotechnol. 3:423–428.
- Poland CADR, Schinwald A, Donaldson K, Cherrie J. 2018. What makes a fibre hazardous to health? The asbestos origins of the fibre length threshold and its verification with nanotechnology. Occup Health. 15:21–26.
- Povey A. 2008. Molecular assessment of exposure, effect, and effect modification. Epidemiology of work related diseases. London, UK: BMJ Publishing Group; p. 463–484.
- Qi HS, Day AJ. 2007. Investigation of disc/pad interface temperatures in friction braking. Wear. 262:505–513.
- Rittinghausen S, Hackbarth A, Creutzenberg O, Ernst H, Heinrich U, Leonhardt A, Schaudien D. 2014. The carcinogenic effect of various multi-walled carbon nanotubes (MWCNTs) after intraperitoneal injection in rats. Part Fibre Toxicol. 11:59.
- Rodelsperger K, Jahn H, Bruckel B, Manke J, Paur R, Woitowitz HJ. 1986. Asbestos dust exposure during brake repair. Am J Ind Med. 10:63–72.
- Rodelsperger K, Woitowitz HJ, Bruckel B, Arhelger R, Pohlabeln H, Jockel KH. 1999. Dose-response relationship between amphibole fiber lung burden and mesothelioma. Cancer Detect Prev. 23:183–193.
- Roggli VL, George MH, Brody AR. 1987. Clearance and dimensional changes of crocidolite asbestos fibers isolated from lungs of rats following short-term exposure. Environ Res. 42:94–105.
- Roggli VL, Vollmer RT, Butnor KJ, Sporn TA. 2002. Tremolite and mesothelioma. Ann Occup Hyg. 46:447–453.
- Rohl AN, Langer AM, Klimentidis R, Wolff MS, Seilikoff IJ. 1977. Asbestos content of dust encountered in brake maintenance and repair. Proc R Soc Med. 70:32–37.
- Rohl AN, Langer AM, Wolff MS, Weisman I. 1976. Asbestos exposure during brake lining maintenance and repair. Environ Res. 12:110–128.
- Saber AT, Mortensen A, Szarek J, Koponen IK, Levin M, Jacobsen NR, Pozzebon ME, Mucelli SP, Rickerby DG, Kling K, et al. 2016. Epoxy composite dusts with and without carbon nanotubes cause similar pulmonary responses, but differences in liver histology in mice following pulmonary deposition. Part Fibre Toxicol. 13:37.
- Sahu SC, Casciano D. 2009. Nanotoxicity: from in vivo and in vitro models to health risks. Chichester: John Wiley.
- Salazar N, Cely-Garcia MF, Breysse PN, Ramos-Bonilla JP. 2015. Asbestos exposure among transmission mechanics in automotive repair shops. Ann Occup Hyg. 59:292–306.
- Schinwald A, Chernova T, Donaldson K. 2012a. Use of silver nanowires to determine thresholds for fibre length-dependent pulmonary inflammation and inhibition of macrophage migration in vitro. Part Fibre Toxicol. 9:47.
- Schinwald A, Murphy FA, Prina-Mello A, Poland CA, Byrne F, Movia D, Glass JR, Dickerson JC, Schultz DA, Jeffree CE, et al. 2012b. The threshold length for fiber-induced acute pleural inflammation: shedding light on the early events in asbestos-induced mesothelioma. Toxicol Sci. 128:461–470.
- Searl A, Buchanan D, Cullen R, Jones A, Miller B, Soutar C. 1999. Biopersistence and durability of nine mineral fibre types in rat lungs over 12 months. Ann Occup Hyg. 43:143–153.
- Searl A. 1997. A comparative study of the clearance of respirable para-aramid, chrysotile and glass fibres from rat lungs. Ann Occup Hyg. 41:217–233.
- Service RF. 1998. Nanotubes: the next asbestos? Science (New York, NY). 281:941.
- Spirtas R, Keehn R, Wright W, Stark A, Beebe G, Dickson E. 1985. Mesothelioma risk related to occupational or other asbestos exposure – preliminary results from a case-control study. Am J Epidemiol. 122:518–518.
- Stanton M, Layard M, Tegeris A, Miller E, May M, Morgan E. 1981. Relation of particle dimension to carcinogenicity in amphibole asbestoses and other fibrous minerals. JNCI. 67:965–975.
- Stanton MF, Wrench C. 1972. Mechanisms of mesothelioma induction with asbestos and fibrous glass. J Natl Cancer Inst. 48:797–821.
- Stayner LT, Dankovic DA, Lemen RA. 1996. Occupational exposure to chrysotile asbestos and cancer risk: a review of the amphibole hypothesis. Am J Public Health. 86:179–186.
- Suzuki Y, Yuen SR, Ashley R. 2005. Short, thin asbestos fibers contribute to the development of human malignant mesothelioma: pathological evidence. Int J Hyg Environ Health. 208:201–210.
- Takata A, Yamauchi H, Toya T, Aminaka M, Shinohara Y, Kohyama N, Yoshida K. 2009. Forsterite exposure causes less oxidative DNA damage and lung injury than chrysotile exposure in rats. Inhal Toxicol. 21:739–746.
- Teschke K, Morgan MS, Checkoway H, Franklin G, Spinelli JJ, van Belle G, Weiss NS. 1997. Mesothelioma surveillance to locate sources of exposure to asbestos. Can J Public Health. 88:163–168.
- Teschke K. 2016. Thinking about occupation-response and exposure–response relationships: vehicle mechanics, chrysotile, and mesothelioma. Ann Occup Hyg. 60:528–530.
- Teta MJ, Lewinsohn HC, Meigs JW, Vidone RA, Mowad LZ, Flannery JT. 1983. Mesothelioma in Connecticut, 1955–1977. Occupational and geographic associations. J Occup Med. 25:749–756.
- The Royal Society, Royal Academy of Engineering. 2004. Nanoscience and nanotechnologies: opportunities and uncertainties. London: The Royal Society.
- Trittschack R, Grobéty B. 2013. The dehydroxylation of chrysotile: a combined in situ micro-Raman and micro-FTIR study. Am Mineral. 98:1133–1145.
- Turci F, Tomatis M, Lesci IG, Roveri N, Fubini B. 2011. The iron-related molecular toxicity mechanism of synthetic asbestos nanofibres: a model study for high-aspect-ratio nanoparticles. Chem Eur J. 17:350–358.
- Turi JL, Yang F, Garrick MD, Piantadosi CA, Ghio AJ. 2004. The iron cycle and oxidative stress in the lung. Free Radic Biol Med. 36:850–857.
- Vainio H, Boffetta P. 1994. Mechanisms of the combined effect of asbestos and smoking in the etiology of lung cancer. Scand J Work Environ Health. 20:235–242.
- Valavanidis A, Vlachogianni T, Fiotakis K, Loridas S. 2013. Pulmonary oxidative stress, inflammation and cancer: respirable particulate matter, fibrous dusts and ozone as major causes of lung carcinogenesis through reactive oxygen species mechanisms. IJERPH. 10:3886–3907.
- Valentine R, Chang MJ, Hart RW, Finch GL, Fisher GL. 1983. Thermal modification of chrysotile asbestos: evidence for decreased cytotoxicity. Environ Health Perspect. 51:357–368.
- Virta RL. 2002. Asbestos geology, mineralogy, mining, and uses. Vol. Version 1.0. Reston, VA: U.S. Dept. of the Interior, U.S. Geological Survey (US Geological Survey Open-File Report).
- Virta RL. 2006. Worldwide asbestos supply and consumption trends from 1900 through 2003. Vol. 1298. Reston (VA): U.S. Geological Survey. (US Geological Survey Circular; iv, 80 p).
- Wagner JC, Sleggs CA, Marchand P. 1960. Diffuse pleural mesothelioma and asbestos exposure in the North Western Cape Province. Br J Ind Med. 17:260–271.
- Wang J, Schlagenhauf L, Setyan A. 2017. Transformation of the released asbestos, carbon fibers and carbon nanotubes from composite materials and the changes of their potential health impacts. J Nanobiotechnol. 15:15.
- Wang NS. 1975. The preformed stomas connecting the pleural cavity and the lymphatics in the parietal pleura. Am Rev Respir Dis. 111:12–20.
- Wang NS. 1985. Anatomy and physiology of the pleural space. Clin Chest Med. 6:3–16.
- Weir FW, Meraz LB. 2001. Morphological characteristics of asbestos fibers released during grinding and drilling of friction products. Appl Occup Environ Hyg. 16:1147–1149.
- Welch LS, Acherman YIZ, Haile E, Sokas RK, Sugarbaker PH. 2005. Asbestos and peritoneal mesothelioma among college-educated men. Int J Occup Environ Health. 11:254–258.
- Welch LS. 2007. Asbestos exposure causes mesothelioma, but not this asbestos exposure: an amicus brief to the Michigan Supreme Court. Int J Occup Environ Health. 13:318–327.
- WHO. 1997. Determination of airborne fibre number concentrations: a recommended method, by phase-contrast optical microscopy (membrane filter method).Geneva: World Health Organization.http://www.who.int/iris/handle/10665/41904
- Wohlleben W, Brill S, Meier MW, Mertler M, Cox G, Hirth S, von Vacano B, Strauss V, Treumann S, Wiench K. 2011. On the lifecycle of nanocomposites: comparing released fragments and their in-vivo hazards from three release mechanisms and four nanocomposites. Small. 7(16):2384–2395.
- Woitowitz HJ, Rodelsperger K. 1994. Mesothelioma among car mechanics? Ann Occup Hyg. 38:635–638.
- Wong O. 2001. Malignant mesothelioma and asbestos exposure among auto mechanics: appraisal of scientific evidence. Regul Toxicol Pharmacol. 34:170–177.
- Wong O. 2006. The interpretation of occupational epidemiologic data in regulation and litigation: studies of auto mechanics and petroleum workers. Regul Toxicol Pharmacol: RTP. 44:191–197.
- Woźniak H, Wiecek E, Bielichowska-Cybula G. 1991. The fibrogenic activity and neurotoxicity of heat-treated chrysotile. Pol J Occup Med Environ Health. 4:21–31.
- Yoshikawa N, Kashimura K, Hashiguchi M, Sato M, Horikoshi S, Mitani T, Shinohara N. 2015. Detoxification mechanism of asbestos materials by microwave treatment. J Hazard Mater. 284:201–206.
- Zaremba T, Krząkała A, Piotrowski J, Garczorz D. 2010. Study on the thermal decomposition of chrysotile asbestos. J Therm Anal Calorim. 101:479–485.
- Zhang H, Ji Z, Xia T, Meng H, Low-Kam C, Liu R, Pokhrel S, Lin S, Wang X, Liao Y-P, et al. 2012. Use of metal oxide nanoparticle band gap to develop a predictive paradigm for oxidative stress and acute pulmonary inflammation. ACS Nano. 6:4349–4368.