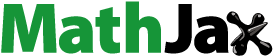
Abstract
Internal transport barriers (ITBs) observed in magnetically confined toroidal plasmas are promising for achieving high-performance burning plasmas, which are established from low-confinement L-mode plasmas dominated by anomalous transport exhibiting strong profile constraints. We studied the origin causing such L-mode plasmas using flux-driven gyro-kinetic based global toroidal simulations covering from core to edge. The simulations are incorporated with real space based statistical probability density function (PDF) approach, which directly measures the heat flux eddy distribution across the entire torus to identify the non-diffusive nature of turbulent transport with different spatio-temporal scales, such as micro-scale avalanches, macro-scale bursts, meso-scale shear layers, etc., as the origin causing the constraints. Based on studies of such L-mode plasmas, we present a methodology to overcome such constraints and demonstrate ITB formations by the control of quasi-stationary global radial
shear through the toroidal plasma rotation driven by either externally using momentum injection and intrinsically using both ions and electron simultaneous heating.
Introduction
Internal transport barriers (ITBs) have attracted attention as a promising operation scenario for realising high-performance plasmas with steep pressure gradients inside cores and have been intensively studied since the 1990s (Citation1–3). In the scenario, the structure of ambient magnetic field, characterised by the safety factor and the magnetic shear
, plays a key role in establishing ITBs. The structure with weak and/or nearly zero magnetic shear as well as reversed magnetic shear are considered appropriate to initiate ITB, while the characteristics, such as radial location, spatial scale length, especially its stability, are sensitively influenced by them (Citation4). Therefore, not only the trigger mechanism of ITB but also its stable sustainment is of particular importance (Citation4).
In has been generally found that a strong reversed magnetic shear configuration, which is established by applying strong central heating during the current rump-up phase to delay the current penetration time, leads to a box-type ITB with a steep pressure gradient (Citation3,Citation4), while those of weak and/or nearly zero magnetic shear leads to a parabolic-type ITB with a gentle pressure gradient (Citation4–7). In the former case, ITB shows higher performance capability (Citation3), but it is frequently collapsed due to the appearance of MHD instabilities, such as infernal type MHD mode (Citation8,Citation9), double tearing mode (Citation10), etc. In the latter case, performance is moderate, but can be sustained over longer time scales, which is referred to as ‘optimum shear’ (Citation6). It has been found that ion ITBs are formed in plasmas with nearly zero magnetic shear around surface, which is initiated by MHD events such as long-lasting mode (LLM) and also electron fish-bone mode (Citation11,Citation12). Recently, ITBs with higher fast ion fraction in the weak magnetic shear and lower density regime are sustained stably over 30 sec keeping central ion temperature around 10 keV (Citation13).
On the other hand, ITBs are established from a low-confinement L-mode plasmas with auxiliary heating which are dominated by turbulent transport and exhibits strong constraints during profile formation and relaxation. The present authors have studied such L-mode plasmas based on global gyro-kinetic toroidal simulations using GKNET covering from core to edge as a flux-driven system with heat source and sink (Citation14). Non-diffusive processes with different spatio-temporal scales are found to play an important role as the origin causing such constraints, such as (I) radially localised fast time scale avalanches, (II) radially extended global bursts, (III) meso-scale shear layers coupled with pressure corrugations which evolve radially with a slower time scale (Citation15–18). So far, they have been studied by measuring correction length and correlation time incorporated with Fourier spectrum analyses. However, since such qualitatively different non-diffusive processes take place in a mixed manner, resultant heat flux exhibits highly complex features. In order to find recipes for initiating ITB from such complex L-mode plasmas, new methodologies need to be introduced to clearly identify them not only qualitatively but also quantitatively.
Study for hat flux PDF (15–18)
For this purpose, here we explore the real space (not Fourier space) based statistical approach which directory measures the size distribution of heat flux eddied in whole torus and construct probability density function (PDF) with
the eddy size (area) with respect to the average ion gyro-radius
. Figure illustrates the results of the PDF analyses employing the data from flux-driven simulation incorporated incorporated with adiabatic electron response at a quiescent phase (a1) and a bursting phase (a2), and corresponding heat flux contribution (right bottom) for positive (red) and negative (green) components and associated net heat flux
at the bursting phase (b2), where
, and
represents the heat flux-driven by
-th eddy.
Figure 1. Heat flux PDF for (a1) quiescent phase and (a2) bursting phase, and corresponding heat flux contribution (right bottom) for both positive (red) and negative (green) components and associated net heat flux for bursting phase (a2). [modified from ref. (Citation16)].
![Figure 1. Heat flux PDF P(S) for (a1) quiescent phase and (a2) bursting phase, and corresponding heat flux contribution (right bottom) for both positive (red) and negative (green) components and associated net heat flux for bursting phase (a2). [modified from ref. (Citation16)].](/cms/asset/ad45cfe5-d304-44e4-ace9-66d775842c35/grad_a_2292727_f0001_oc.jpg)
In the quiescent phase (a1), the size-PDF is approximately fitted by three piecewise power laws as (a) in
, (b)
in
and (c)
in
, respectively. Note that the linear ion temperature gradient (ITG) modes, which are the dominant instability free energy source in the present system, locates around
. On the other hand, at the bursting phase (a2), the PDF exhibits non-power law irregular humps in the region
, which maximum size reaches
. Such humps are ascribed to the radially extend global structures which are spontaneously induced due the phase alignment of smaller scale eddies in the radial direction resulting from the toroidal coupling of poloidal Fourier harmonics. The mechanism only works in plasmas with finite magnetic shear
, while the effect becomes weaker as
becomes smaller (Citation16).
From the property that the size-PDF shows a different power law with respect to the eddy size , we assume that each region of eddy size
in Figure is assigned to each mechanism discussed above, i.e. (I), (II), and (III) (Citation18). Namely, we assigned region (a) as corresponding to a diffusive process dominated by smaller size eddies, while regions (b) and (c) are assigned to non-diffusive processes, i.e. (I) and (II). Furthermore, since the PDF is enhanced in region (c) during the bursting phase (a2) as seen in Figure , it is considered that region (c) corresponds to the bursting dynamics (II). Considering that region (b) is not influenced even if bursts take place, region (b) corresponds to the radially localised avalanches (I). The
shear layers, i.e., mechanism (III), is considered to be included in region (b), since it tends to be scrambled and/or diminished when global bursts take place (see Ref. (Citation15)). As can also be seen in heat flux contribution for each eddy size (right bottom in Figure ), a large amount of free energy is extracted through larger bursting events in region (b), which is responsible for causing constrains in the profiles formation and relaxation. To initiate ITBs by breaking such constrains, mechanism (I) or (II) must be suppressed. However, the mechanism (II) is likely to be suppressed which can be achieved by reducing the magnetic shear and preventing spontaneous phase alignment. This is consistent to the tendency for ITBs to formed in plasmas with weak and/or zero magnetic shear, or those with reversed magnetic shear.
Flux-driven simulation for ITB formation (19)
Based on the above idea, we present methodologies to overcome the L-mode constraints leading to ITBs through controlling the quasi-stationary global radial electric field shear, i.e., , by externally driving the toroidal rotation shear via direct momentum input (A) or intrinsically vis selective excitation of ITG modes and trapped electron mode (TEM) modes in different radial locations (B), which rotate different poloidal directions. These procedures are incorporated with the control of the magnetic field structure, i.e., those with weak and/or nearly zero magnetic shear, and revered magnetic shear, to suppress the instantaneous phase alignment as discussed above.
In Figure , we show the results of flux-driven simulations using GKNET with gyro-kinetic ion and hybrid electrons where trapped electrons are treated kinetically while passing electrons are adiabatically. Here, two cases are demonstrated, i.e., case (A) with nearly flat -profile with momentum injection assuming NBI to co-current direction around the half minor radius incorporated with the dominant heat source near the centre, case (B) with moderately revered
-profile using simultaneous ion and electron heating in both core region without momentum input. ITBs are found to be initiated in both cases as seen in (A1) and (B1) where ion thermal diffusivities
are reduced locally at the neo-classical level around the half minor radius as seen in (A2) and (B2) as the result of strong radial electric field shears
, which are regulated by radial force balance relation.
Figure 2. Results of flux-driven simulation in the case with (A) momentum input modelling NBI and (B) no-momentum input with applying both ion and electron heating simultaneously. (A1) (B1) show temperature and q profiles and (A2) (B2) show ion heat diffusivity. [from ref. 00]. [from (Citation19)].
![Figure 2. Results of flux-driven simulation in the case with (A) momentum input modelling NBI and (B) no-momentum input with applying both ion and electron heating simultaneously. (A1) (B1) show temperature and q profiles and (A2) (B2) show ion heat diffusivity. [from ref. 00]. [from (Citation19)].](/cms/asset/e5c083de-a814-42b6-904b-d65b376e7ff3/grad_a_2292727_f0002_oc.jpg)
Conclusion
Recently, strong revered magnetic shear plasmas in JT60U was studied, where both ion and electron temperature profiles exhibit strong profile constraints with keeping exponential type function form even when input power was increased (Citation20). Large scale avalanches are considered to be responsible for casing such constraints, which are overcome leading to the initiation of ITBs by increasing input power (Citation21). Here, we studied turbulent transport in normal magnetic shear plasmas and the role of global bursts as the origin of profile constraints. It is worthwhile to study the similarity of avalanches observed in above experiments and those in simulations and specifically the role of heating power for initiating ITBs, which are now under investigation (Citation22).
References
- Koide, Y.; Kikuchi, M., et al. Phys. Rev. Lett. 1994, 72, 3662.
- Levinton, F.M.; Zarnstrorff, M.C., et al. Phys. Rev. Lett. 1995, 75, 4417.
- Ishida, S.; Fujita, T., et al. Phys. Rev. Lett. 1997, 79, 3917.
- Shirai Kikuch, H., et al. Nucl. Fusion 1999, 39, 1713.
- Joffrin, E.; Gorini, G., et al. Plasma Phys. Control. Fusion 2002, 44, 1739.
- Becoulet, A.; Eriksson, L.-G., et al., Nucl. Fusion 2000, 40, 1113.
- Oyama, N., et al. Nucl. Fusion 2007, 47, 689. Nucl. Fusion 49, 065026 (2009).
- Manickam, J.; Pomphrey, N., et al. Nucl. Fusion 1989, 27, 1461.
- Ozeki, T.; Azumi, M., et al. Nucl. Fusion 1995, 35, 861.
- Miho, J.; Kishimoto, Y.; Li, J.Q. Phys. Rev. Lett. 2011, 107, 195001.
- Yu, D.L.; Wei, Y.L., et al. Nucl. Fusion 2016, 56, 056003.
- Wei, D.; Yi, L., et al. Phys. Plasmas 2022, 29, 102106.
- Han, H., et al. Nature 2022, 699, 269. W.H. Ko et al., “Overview of The KSTAR Experiments”, 29th IAEA Fusion Energy Conference (FEC 2023), Nov. 9-14, London.
- K. Imadera, Y. Kishimoto, et al., Proceedings of 26th IAEA-FEC, TH/P3-3 (2016).
- Wang, W.; Kishimoto, Y.; Imadera, K., et al. Nucl. Fusion 2018, 58, 056005.
- Wang, W.; Kishimoto, Y.; Imadera, K., et al. Nucl. Fusion 2020, 60, 066010.
- Y. Kishimoto and K. Imadera, Radiat. Effect. Defect. Solids, 175, 2020 -issue 11-12, p. 1021-1025 (abstracts presented at the NIA annual meeting held at Hampton, VA on August 12, 2020). doi:10.1080/10420150.2020.1845694
- Kishimoto, Y.; Imadera, K., et al. Phil. Trans. R. Soc. A 2023, 381, 20210231.
- Imadera, K.; Kishimoto, Y. Plasma Phys. Control. Fusion 2023, 65, 024003.
- Kin, F.; Itoh, K., et al. Nucl. Fusion 2023, 63, 016015.
- F. Kin, K. Itoh, et al. Sci. Rep..
- Y. Kishimoto, K. Imadera, et al., Proceedings of 29th IAEA-FEC 2023.