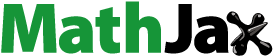
ABSTRACT
In this study, we designed and synthesised MTX and PEG‒grafted chitosan copolymer nanoparticles (PsCM) to load MAG. We generated MAG@PsCM nanoparticles according to the effective ratio of dual drugs of 1:2.5, achieving 3.73% and 8.95% drug loading for MTX and MAG, respectively. Drug efficiency experiments were conducted on MDA-MB-231 breast cancer cells, and the results showed that when MTX was used alone, the tumour cell survival rate was 43.66 ± 1.77%and when low-dose MAG and MTX were used in combination, the survival rate of tumour cells was significantly reduced to 29.82% ± 2.22%. The nanoparticles enhanced the synergistic tumour therapeutic effect of the two drugs with the cell survival rate was 21.94 ± 1.43%, significantly lower compared with the free drugs. MAG@PsCM nanoparticles were significantly taken up by cells at 3, 6 and 12 h, and the total amount taken up increased significantly with time.
Introduction
Triple-negative breast cancer (TNBC) has a high degree of malignancy, and few drugs can be selected for its treatment [Citation1–3]. Moreover, triple-negative breast cancer is prone to metastasis and relapse, leading to poor prognosis [Citation4,Citation5]. Marked by pronounced heterogeneity, specific mutations, and aberrant activation of signalling pathways, TNBC poses a formidable clinical challenge. Consequently, prospective treatment strategies for TNBC encompass targeted therapies directed at critical elements such as DNA repair pathways, androgen receptor signalling pathways, and kinases [Citation6]. Chemotherapy still has a pivotal role in current breast cancer treatment guidelines, especially in the adjuvant therapy and palliative treatment. The clinical treatment of triple-negative breast cancer mainly involves traditional chemotherapy drugs in combination with other small molecule drugs. Nevertheless, monotherapy will always have a limited effect on TNBC treatment. When using traditional chemotherapeutic drugs clinically, the drug resistance developed by the tumour will have a notable effect on patient prognosis, so effectively reducing the tumour drug resistance is the key to improving the prognosis. The main mechanism of action of methotrexate is the inhibition of tumour nucleic acid synthesis through inhibition of dihydrofolate reductase. Patients treated with methotrexate alone are prone to drug resistance and poor prognosis. However, the most classic clinical three-drug regimen of cyclophosphamide, methotrexate and 5-fluorouracil (CMF), has a certain effect on triple-negative breast cancer [Citation7–9].
Previous studies have concluded that the acquisition of stemness by TNBC cells will cause drug resistance [Citation10–12]. Those cells acquire stemness after epithelial-mesenchymal transition (EMT), resulting in increased expressions of certain membrane transport proteins, enzymes and cellular repair-related proteins, which cause drug resistance or recurrence. Moreover, the enhanced tumour cell stemness will reduce the communication proteins between the tumour cells, leading to easier tumour cell spreading and tumour metastasis [Citation13]. Magnolol (MAG) is the main extract from Magnolia officinalis as the traditional Chinese medicine, which contains isolated allyl-substituted biphenolics and has anti-inflammatory, antibacterial, antitumor and antioxidant effects [Citation14–19]. In previous studies, magnolol was considered not only to influence EMT-related pathways to reduce tumour stemness but also to achieve anticancer effects in other ways, such as affecting autophagy [Citation20]. Related studies showed that magnolol can induce the death of HepG2 cells and H460 cells by inducing the cellular autophagy pathway [Citation21,Citation22]. To improve the treatment efficacy while reducing the dose of MTX, in this project, we used the combination of MTX and MAG to increase the applicability.
The in vivo efficacy and metabolism of a variety of drugs are difficult to control, and drugs that lack targeting effects often have greater biological toxicity. To achieve efficient dual-drug delivery and reduce side effects, we constructed a new kind of nanoparticle for drug delivery in this study. Unmodified nanoparticles are easily removed through plasma, but modifying the nanoparticle surface with polyethylene glycol monomethyl ether (mPEG) can reduce the plasma clearance and achieve a long circulation effect [Citation23,Citation24]. Additionally, the attached pH-sensitive linker, which have achieved faster drug release in acidic environments, can also enhance the therapeutic effectiveness [Citation25,Citation26]. Chitosan is a natural polysaccharide with high biocompatibility. Chitosan was connected with methotrexate to form a hydrophobic core to wrap MAG, and the cationic properties of chitosan will increase the uptake of the nanomedicine by tumour cells [Citation27,Citation28]. Surface modification with mPEG will cover this charge and affect cellular uptake. Therefore, in this study we connect mPEG with chitosan via disulphide bond, which can be cleaved and lead to efficient cellular endocytosis via catalysis due to the high tumour concentrations of GSH. After exposing the cationic nanocore, the nanomaterial can more easily enter the cell through endocytosis and absorb protons in the cell, which will result in the destruction of the structure of the nanoparticles in the cell and therefore escaping the immune reaction during entering the body, thus realising pH-responsive drug delivery. In this study, we prepared a PsCM nanoparticle, characterised its synthesis and studied its structure and function in vitro and in vivo to obtain a nanocarrier for the treatment of triple-negative breast cancer ().
Materials and methods
Experimental reagent
Chitosan (5000 Da), 3,3′-dithiodipropionic acid (DA) and 1-(3-dimethylaminopropyl)-3-ethyl carbodiimide hydrochloride (EDCI) were purchased from Macklin Biochemical Technology Co., Ltd. (Shanghai, China). Polyethylene glycol monomethyl ether 5000 (mPEG5000) and 4-dimethylpyridine (DMAP) were purchased from Aladdin Reagent Co., Ltd. (Shanghai, China). Methotrexate (MTX) was purchased from Yuanyuan Biotechnology Co., Ltd. (Shanghai, China).
Synthesis and characterization
In the first step, polyethylene glycol monomethyl-disulphide-carboxylic acid (mPEG-SS-COOH) was synthesised. For the synthesis, 0.1 g of 3,3′-dithiodipropionic acid (DA), 0.1 g of 4-dimethylpyridine (DMAP), 0.2 g of 1-(3-dimethylaminopropyl)-3-ethyl carbodiimide hydrochloride (EDCI), and 2.0 g of polyethylene glycol 5000 (mPEG5000) were stirred and dissolved in dimethyl sulphoxide (DMSO). Mixed system then reacted on a heated thermostatic magnetic stirrer at 40°C for 1 day. After the reaction was complete, the solution was dialysed and dried in a vacuum freezer to yield the mPEG-SS-COOH solid.
Polyethylene glycol monomethyl ether-disulphide-chitosan-methotrexate (mPEG-ss-CS-MTX, PsCM) was then synthesised. First, 1.5 g of mPEG-SS-COOH, 0.1 g of DMAP and 0.2 g of EDCI were dissolved in DMSO. Then, 0.25 g of oligomeric chitosan was added to react at 40°C for 5 hours. Thereafter, 0.4 g, 0.2 g, 0.15 g, 0.1 g and 0.075 g of methotrexate were added to the above system in separate reaction vessels, and the reactions were continued for another day. After each reaction was complete, the solution was dialysed and vacuum freeze-dried to yield the PsCM solid ().
Screening and characterization
Five milligrams of PsCM nanoparticles prepared in five different proportions were dissolved in 5 mL of DMSO, and the solutions were loaded into a common dialysis bag with a molecular weight cut-off of 7000 Da for dialysis. The dialysis bag was placed in a beaker containing 2000 mL of pure water for dialysis for a total of 6 hours, during which the water was replaced with pure water every 30 minutes. Afterwards, the prepared nanoparticles were taken to detect their size with a dynamic light scattering instrument, and the PsCM material with an appropriate size was selected. Ten milligrams of mPEG, mPEG-SS-COOH, MTX, and the PsCM material were dissolved in 0.5 mL of deuterated DMSO, and then a proton nuclear magnetic resonance (1H-NMR) spectrometer was used to acquire the 1H-NMR spectra of the nanomaterials.
Determination of the synergy between MTX and MAG
The purchased MDA-MB-231 cells (Procell Life Science & Technology Co., Ltd.) were grown in DMEM-H containing 10% foetal bovine serum and 1% penicillin/streptomycin in an environment containing 5% CO2 at 37°C. MDA-MB-231 cells were digested and seeded into a 96-well plate (5,000 cells per well). MTX at concentrations of 1 μg/mL, 0.5 μg/mL, 0.25 μg/mL and 0.125 μg/mL, and MAG at concentrations of 20 μg/mL, 15 μg/mL, 10 μg/mL and 5 μg/mL, were separately dispersed in complete medium, and then the cells were treated with the above solutions for 1 day. After that, the medium was replaced with fresh complete medium containing 10% CCK-8 reagent, and then the samples were placed in the dark in a 37°C incubator for 1 hour before the absorbance was measured at 490 nm. Similarly, combinations of different concentrations of MTX (0 μg/mL, 0.1 μg/mL, and 0.2 μg/mL) and MAG (0 μg/mL, 5 μg/mL, and 10 μg/mL) were added to complete medium, and then the cells were treated with these solutions for 1 day. In the same way as described above, after treatment with CCK-8 reagent, the absorbance of the samples in the 96-well plate wells was measured, and cell activity was calculated. After that, the calculated cell activity data were imported into SynergyFinder 2.0 software to calculate the synergistic effect of MTX and MAG.
Hydrodynamic particle size, zeta potential and TEM
Ten milligrams of PsCM material was dissolved in 5 mL of DMSO, and the above solution was loaded into a common dialysis bag with a molecular weight cut-off of 7000 Da. The dialysis bag was placed in a beaker containing 2000 mL of pure water for dialysis for a total of 6 hours, during which the water was replaced with pure water every 30 minutes to yield PsCM nanoparticles (PsCM Nanoparticles). MAG-loaded nanoparticles (MAG@PsCM Nanoparticles) were prepared using the optimum concentrations of MTX and MAG that produced synergistic effects, and the method was similar to that for the PsCM Nanoparticles. The hydrodynamic particle sizes and zeta potentials of the two Nanoparticles were measured with a dynamic light scattering instrument with the following settings. Hydrodynamic particle size: wavelength 658 nm, temperature 25 ± 0.1°C, and angle 90°. Zeta potential: 1.4 V/cm, 13.0 mA, and 25 ± 0.1°C. Moreover, the two prepared nanoparticles were dropped onto a copper net, air-dried, and then scanned and photographed by transmission electron microscopy (TEM) microscope.
Drug loading and drug release
The MTX and MAG solutions were prepared at concentrations of 0 μg/mL, 2 μg/mL, 4 μg/mL, 6 μg/mL, 8 μg/mL, and 10 μg/mL. Thereafter, the above solutions were placed in an UV‒Vis spectrophotometer, and the absorption peaks of the two drugs were detected at 284 nm and 350 nm, respectively. The prepared MAG@PsCM nanoparticles were diluted 10-fold and placed in an UV‒Vis spectrophotometer to detect the absorption peaks of the PsCM material and MAG@PsCM nanoparticles at 284 nm and 350 nm and to calculate the contents of MTX and MAG in the PsCM material and MAG@PsCM nanoparticles according to the dual-wavelength method.
To detect drug release, 5 mL of the prepared nanoparticles was placed in a dialysis bag (7000 Da) and dialysed on a 37°C constant temperature shaker (75 rpm) containing 30 mL of PBS (pH = 7.4 or pH = 5.5, containing 10 mM GSH). At 0, 0.5, 2, 4, 8, 16, 24, and 48 hours, 30 mL of fresh PBS was used to replace the PBS in the system. PBS was collected at specific time points (t), the volume of PBS was determined (Vt), the absorption at 284 nm and 350 nm was measured, and the concentration of the drug was calculated as Ct.
Specifically, Qt is the drug release rate at time t (hours) (t = 0, 0.5, 1, … n … 48; V0 and C0 are equal to 0); Vdb is the volume of PBS in the dialysis bag; and Cdb (drug) is the initial concentration of nanomaterials.
Cytotoxicity of nanoparticles
MDA-MB-231 cells were inoculated into 96-well plates (5000 cells per well). Different concentrations of MTX, MTX+MAG and MAG@PsCM nanoparticles in complete medium (MTX:MAG = 1:2.42) were prepared and cultured with the cells in the 96-well plates for one day. After that, the culture medium was replaced with fresh complete culture medium containing 10% CCK-8 reagent for incubation in a 37°C incubator for 1 hour. The absorbance at 490 nm was detected, and the cell activity was calculated.
Cellular uptake
MDA-MB-231 cells were seeded into 24-well plates (20,000 cells per well). Cy5@PsCM nanoparticles containing 2 μg/mL Cy5 were added to the cells when they reached 50% confluence. The cells were then stained with DAPI (1 μg/mL), and photographs were taken at 2, 4, and 6 hours. Finally, cellular uptake was calculated using ImageJ software.
Cell migration experiment
MDA-MB-231 cells were digested and seeded into a 24-well plate (50,000 cells per well). When the cells had grown to 80% confluence, different concentrations of MTX, MTX + MAG and MAG@PsCM nanoparticles were added for culture. Cells were photographed at 0, 6 and 12 hours, and cell migration was calculated using ImageJ software.
Statistical analysis
All the experiments were repeated for three times, and the data were represented as mean ± standard deviation (SD). One-way ANOVA and t tests were employed to study the differences between experimental groups by using GraphPad 7.0. and Origin 2017. * p < 0.05 and ** p < 0.01 were considered to be significant, while *** p < 0.001 and **** p < 0.0001 were considered to be highly significant.
Results
Material ratio of the PsCM nanoparticles
First, we calculated the molar masses of the chitosan monomer and mPEG-SS-COOH. To achieve a positively charged surface and connect the amino carboxyl group with MTX, we designed the compound mPEG-SS-COOH, which was generated in a mass ratio of approximately 6:1 of mPEG to DA. Accordingly, we adjusted the feed ratio of mPEG-SS-COOH to MTX to produce a variety of nanomaterials with different MTX substitution degrees. These materials were used to prepare different nanoparticles, and the particle size of each nanoparticle was detected (). The data in show that the polydispersity index (PDI) of each type of nanoparticle was good, and the size of nanoparticle notably increased with an increase in the degree of MTX substitution. When the ratio of mPEG-SS-COOH to MTX was 20:1, the PsCM nanoparticles had a smaller size (280.57 ± 2.77 nm) and could be used in follow-up experiments.
Figure 3. Dimensions of the nanoparticles prepared from the mPEG-ss-CS-MTX materials with different feeding ratios. The legends A, B, C, D, E correspond to PsCM nanoparticles with mPEG:MTX ratios of 20:1, 15:1, 10:1, 7.5:1, and 3.5:1, respectively.

Table 1. Average particle sizes and PDI values of PsCM nanoparticles prepared from PsCM polymers with different hydrophobic substitutions.
Characterization of the PsCM nanomaterials
To demonstrate that the synthesis of the nanomaterials was successful, we examined the 1H-NMR spectra of the nanomaterials. As shown in , mPEG has a characteristic peak at 3.50 ppm, while methylene peaks also appeared in the spectra of the mPEG-SS-COOH and PsCM materials. The methylene peak next to the disulphide bond of mPEG-SS-COOH appeared at 2.88 ppm after modification with 3-methylene-dithiodipropionic acid, which indicated that the incorporation of 3-methylene-dithiodipropionic acid was successful. This characteristic peak also appeared in PsCM, which indicated that mPEG-SS-COOH was grafted onto chitosan. Methotrexate has the characteristic peak from the pteridine ring at 8.60 ppm and a characteristic peak from methyl hydrogen on the pteridine ring at 2.32 ppm. The appearance of the above characteristic peaks in the PsCM materials indicated that methotrexate was grafted onto PsCM. The characteristic peak of the amino group of chitosan appeared between 1–2 ppm in the 1H-NMR spectrum of PsCM. Because we designed the syntheses to include an excess of some raw materials and used dialysis bags of an appropriate size to remove unreacted substances or side product, we can assume that relatively pure products were obtained, and the above data showed the success of the synthesis of PsCM materials.
Synergistic effect of MTX with MAG
First, we used two single agents individually to treat triple-negative breast cancer MDA-MB-231 cells. As shown in , the survival rate of the tumour cells was 40.46 ± 4.29% when the concentration of MTX was 1 μg/mL, and the survival rate of the tumour cells was 9.05 ± 2.82% when the concentration of MAG was 20 μg/mL. Thus, both of these concentrations have inhibitory effects on MDA-MB-231 cells. The IC50 values of MTX and MAG were 0.49 ± 0.06 μg/mL and 11.72 ± 0.70 μg/mL, respectively. Next, we treated the tumour cells with a combination of drugs at different concentrations. As shown in , the survival rate of the tumour cells was 67.19 ± 1.85% when the concentration of MTX was 0.1 μg/mL and the concentration of MAG was 5 μg/mL, and the survival rate of the tumour cells was 44.45 ± 3.33% when the concentration of MTX was 0.2 μg/mL and the concentration of MAG was 10 μg/mL. Moreover, the synergy score of MTX and MAG was 14.836 within the experimental concentration range, which shows that this combination of drugs has an obvious synergistic effect.
Figure 5. Inhibition of MDA-MB-231 triple-negative breast cancer cell proliferation by MTX and MAG and their synergistic effects. a and b show the proliferation of MDA-MB-231 cells treated with MTX and MAG at different concentrations. c: the proliferation of tumor cells treated with a combination of both drugs. d: the synergistic effect of MTX and MAG calculated by SynergyFinder 2.0 software. * P<0.05 and * * P<0.01 indicate significant differences, * * * P<0.001 and * * * * P<0.0001 indicate highly significant differences.

Preparation and morphological characterization of mAG@PsCM nanoparticles
Due to the large difference in the effective concentrations of MTX and MAG, excessive MAG loading affects the size and dispersion of the nanoparticles, therefore an MTX:MAG mass ratio of 1 to 2.5 was used to prepare the nanoparticles. According to the results of UV‒visible absorption spectrophotometry, the content of MTX in the PsCM materials was calculated to be approximately 4.06 ± 0.18%, so the ratio of MAG to PsCM material used in the preparation of the dual-drug nanoparticles was approximately 10.15%, which is within the maximum drug loading range of most polymer nanoparticles. The PsCM nanoparticles and MAG@PsCM nanoparticles were prepared by dialysis. As shown in , the hydrodynamic particle sizes of the two nanoparticles were 276.8 ± 2.6 nm and 341.0 ± 1.6 nm, respectively, and their sizes increased after drug loading. Moreover, the nanoparticles were regularly spherical in shape, as observed under the electron microscope. Because the outermost layer of the nanoparticles is a thick PEG layer, the hydrated particle size of the nanoparticles was significantly larger than that determined by transmission electron microscopy. Moreover, the surface potentials of the two nanoparticles were 21.7 ± 0.5 mV and 20.7 ± 0.3 mV, which may be because MAG is electrically neutral, causing these two types of nanoparticles to have similar positive surface potentials.
Figure 6. Hydrodynamic sizes (a, b), zeta potentials (c, d) and transmission electron microscopy images (e, f) of the blank nanoparticles (a, c, e) and drug-loaded nanoparticles (b, d, f).

Afterwards, we measured the drug load into the nanoparticles and drug release from the nanoparticles (). The drug load of MAG into the MAG@PsCM nanoparticles was calculated to be approximately 8.95% by UV‒Vis spectroscopy. Next, we conducted drug release experiments to evaluate the sustained release of the drugs from the nanoparticles and their tumour microenvironment-endosome responsiveness. The results showed that the release of free MAG in a neutral environment was faster, and 59.76 ± 2.79% was released within 8 hours. Moreover, in the simulated tumour-endosome microenvironment (high GSH concentration, low pH), the release of free MAG increased significantly, and 74.24 ± 2.43% was released within 8 hours. Compared with the free drug, the release rate from the nanoparticles in a neutral environment was significantly lower over 8 hours (22.96 ± 2.11%), while the MAG release rate from the nanoparticles was 49.95 ± 2.80% over 48 hours, which indicated that the drug was released from the nanoparticles slowly. Compared with the neutral environment, in the simulated tumour-endosome microenvironment, the MAG release rate from the nanoparticles was 68.05 ± 3.27%, which was a significantly increase. The release rate of free MTX was 68.87 ± 2.65% in a neutral environment over 8 hours, which increased to 79.01 ± 3.77% in the simulated tumour-endosome microenvironment. Moreover, compared with the release of MAG, the release of the MTX grafted onto the nanoparticles was slower. The MTX release rate from the nanoparticles in the neutral environment over 48 hours was 28.76 ± 0.85%, while the MTX release rate in the tumour-endosome microenvironment was 35.86 ± 2.99%, showing a significant difference. This also means that in the tumour-endosome microenvironment, the structure of the nanoparticles will be destroyed, resulting in relatively rapid drug release.
In vitro antitumor activity of mAG@PsCM nanoparticles
Next, we investigated whether MAG@PsCM nanoparticles inhibited the proliferation of triple-negative breast cancer cells in vitro. We used the CCK-8 assay to detect MDA-MB-231 cell proliferation after drug treatment (). Free methotrexate mainly inhibited the folate pathway. When the concentration was high (above 1 μg/mL), the inhibitory effect of free methotrexate on cells increased slowly and caused side effects in vivo.
Figure 8. Toxicity of free MTX, free MTX with MAG and MAG@PsCM nanoparticles to MDA-MB-231 cells. * P <0.05 and * * P <0.01 indicate significant differences, and * * * P <0.001 and * * * * P <0.0001 indicate highly significant differences.

The tumour cell survival rate was 43.66 ± 1.77% when treated with MTX alone, and significantly decreased to 29.82 ± 2.22% when using low doses of MAG in synergy with MTX. Moreover, the nanoparticles further improved the synergistic tumour treatment efficacy of the two drugs compared with the free drugs (cell survival rate was 21.94 ± 1.43%). We further assessed the cytotoxicity of MAG@PsCM nanoparticles on NIH3T3, RAW264.7, and 4T1 cells across varying concentrations. Supplementary Figure S1 illustrates that, within the dose range, NIH3T3 cells showed no significant cytotoxicity, indicating minimal toxicity to fibroblasts. RAW264.7 cells exhibited a cytotoxic effect at the highest nanoparticle concentration (MTX concentration of 2.00 μg/ml, MAG concentration of 4.84 μg/ml), suggesting potential toxicity to macrophages at elevated doses. Notably, 4T1 cells displayed a dose-dependent cytotoxicity, mirroring the effect observed on MDA-MB-231 cells.
To further explore the mechanism by which MAG@PsCM nanoparticles enhance the cytotoxicity of both drugs, we performed cellular uptake experiments. As shown in , MAG@PsCM nanoparticles was significantly ingested by cells at 3, 6, and 12 hours, and the total amount taken up increased significantly over time. Nanoparticles were continuously be taken up by cells during our experiment, which will significantly increase the intracellular drug concentration and thus improve drug efficiency. To investigate the role of the nanoparticles in tumour migration, scratch experiments were performed (). In the control group, the tumour cells significantly migrated to the scratches from 0 to 12 hours compared with the certain reduction in the migration of tumour cells treated with free MTX. However, the migration rate of tumour cells treated with nanoparticles was significantly reduced compared with the control and free MTX groups. This indicates that MAG-loaded MAG@PsCM nanoparticles can effectively prevent MDA-MB-231 cell migration. In conclusion, the MAG@PsCM nanoparticles we prepared can be continuously absorbed by cells in vitro and inhibit tumour growth while reducing tumour migration.
Discussion
Intelligent nanodelivery systems responding to the tumour microenvironment have gradually become a research hotspot. Intelligent nanodelivery systems mainly encompass three distinct approaches: active-targeting nanocarriers, micro- or cytoplasmic-environment responsive nanocomposites, and external stimuli-triggered nanoplatforms. Among these, micro- or cytoplasmic-environment responsive nanocomposites primarily leverage the characteristics of low pH, high ROS, and elevated GSH in the tumour microenvironment to achieve smart nanodrug delivery [Citation29]. Polymer nanoparticles can be synthesised from monomers or prepared nano-polymers, and particles with different sizes and drug-carrying capabilities can be obtained by controlling the ratio of the ingredients [Citation30,Citation31]. Polymeric nanoparticles usually have polymersomal, micellular, or dendritic structures [Citation32,Citation33]. In recent years, researchers have designed a variety of nano-delivery systems for the treatment of TNBC. Among them, MTX-PEG-modified CG/DMMA polymeric micelles were designed for targeted delivery of doxorubicin [Citation34]; biotin receptor‒targeting nanogels were designed to load methotrexate [Citation35]; cholesterol biguanide hydrochloride nanoparticles were designed to load magnolol [Citation16]; self-assembling lecithin-based mixed polymeric micelles were designed to load honokiol (Akt inhibitor) [Citation36]. The PsCM nanoparticles prepared in this work are polymersomes made using amphiphilic block copolymers, which are comparable to liposomes and are usually locally reactive but have higher stability and cargo retention efficiency, making them effective for delivering therapeutic drugs to cells. Similar nano-delivery systems have previously found successful applications in the treatment of osteosarcoma, demonstrating promising therapeutic outcomes [Citation37]. Active cellular targeting of nano-drug delivery systems is achieved by modifying high-affinity ligands or aptamers to target tumour cells and deliver antitumor drugs [Citation38].
In this study, we first synthesised GSH-responsive mPEG and cografted it onto chitosan with the small molecule drug methotrexate. Next, we adjusted the feeding ratio of mPEG-SS-COOH and MTX to obtain a variety of nanomaterials with different substitution degrees of MTX. After preparing different nanoparticles, we detected the particle size of each. The hydrodynamic dimension of the nanoparticles have increased noticeably with the increase of MTX substitution degree, for the larger steric hindrance of MTX and the relative decrease in the hydrophobic group substitution [Citation39,Citation40]. When the feeding ratio of mPEG-SS-COOH and MTX was 20:1, the PsCM Nanoparticles had a smaller size (~280 nm). We further acquired 1H-NMR spectra of the nanomaterials, which showed that the synthesis of the PsCM material was successful. Because the drug was grafted outside the material, the nanoparticles might have simpler structures but less applicability. Due to the large positive charge of chitosan, it produces nanoparticles with a small size and positive zeta potential. There are disulphide bonds in the nanoparticles, which can be broken in an environment containing GSH. Exposed cationic nanopores more easily enter the endosome through endocytosis and absorb protons in the endosome, resulting in the destruction of the nanoparticle structure and escape from the endosome for pH-responsive drug release. This enabled the PsCM nanoparticles to achieve GSH and acid dual-responsive release in the simulated tumour-endosome microenvironment.
We used the CCK-8 method to detect the proliferation of MDA-MB-231 cells after drug treatment and performed scratch experiments to study the effect of the nanoparticles on tumour migration. Compared with the free drugs, the nanoparticles further improved the curative effect of the two drugs to synergistically treat tumours. The uptake experiments showed that the nanoparticles were continuously taken up by the cells during our experiment, which will significantly increase the concentrations of the drugs in cells, thereby improving drug efficiency. Scratch experiments showed that MAG-loaded MAG@PsCM nanoparticles could effectively prevent the migration of MDA-MB-231 cells. MAG@PsCM nanoparticles exhibit low toxicity towards NIH3T3 cells (fibroblasts), while high doses show some toxicity towards RAW264.7 cells (macrophages). However, MTX inherently induces M1 polarisation in macrophages. Additionally, MAG has the potential to induce M1 polarisation in macrophages through pathways such as PI3K. This dual action may contribute to improving the tumour immune microenvironment.
At present, there are many ways to achieve nanoparticle responsiveness to the tumour microenvironment, but there is still a large gap in their clinical application for the treatment of tumours. In the current study, tumour microenvironment response elements can be introduced as the most innovative elements, such as microRNA response elements [Citation41]. Moreover, the constructed microenvironment response system lacks a cascade design, which makes the complex nanoparticle-tumour response process appear to be complete in a single step. In addition, the current constructed nanodelivery system does not precisely locate tumour cells for tumour targeting, and the introduction of components, such as various tumour-targeted ligands, could further increase the efficiency of the nanodelivery system [Citation42]. In this study, we did not examine the in vivo function of the nanodelivery system, and we may perform these experiments in future studies. There are few nanodrugs that can be selected for clinical use, and nanotreatments are still in the laboratory research stage. However, because of this, the research potential of nanodelivery systems is great. In the future, studies on organic nanoparticles with high biocompatibility will effectively alter the delivery of different types of drugs to achieve efficient and personalised clinical treatment.
Conclusion
The prepared nanomaterials with disulphide bonds and chitosan were characterised by cleaved chemical bonds in GSH and increased solubility in acidic environments, thus conferring dual sensitivity. The nanoparticles could effectively reduce drug release in a neutral environment. Meanwhile, in the simulated tumour-endosome microenvironment, the drug release increased significantly under acidic and GSH conditions, significantly inhibited MDA-MB-231 cell viability, and exhibited a strong inhibitory effect on tumour migration.
Supplementary Figure 1.tif
Download TIFF Image (266.6 KB)Acknowledgments
The authors gratefully acknowledge the support of the Development Fund of Key Laboratory of Study and Discovery of Small Targeted Molecules of Hunan Province.
Disclosure statement
No potential conflict of interest was reported by the author(s).
Supplementary material
Supplemental data for this article can be accessed online at https://doi.org/10.1080/10667857.2023.2288780.
Additional information
Funding
References
- Medina MA, Oza G, Sharma A, et al. Triple-negative breast cancer: a review of conventional and advanced therapeutic strategies. Int J Environ Res Public Health. 2020;17(6):2078. doi: 10.3390/ijerph17062078
- Won KA, Spruck C. Triple‑negative breast cancer therapy: Current and future perspectives. Int J Oncol. 2020;57(6):1245–12. doi: 10.3892/ijo.2020.5135
- Deepak K, Vempati R, Nagaraju GP, et al. Tumor microenvironment: challenges and opportunities in targeting metastasis of triple negative breast cancer. Pharmacol Res. 2020;153:104683. doi: 10.1016/j.phrs.2020.104683
- Derakhshan F, Reis-Filho JS. Pathogenesis of triple-negative breast cancer. Annu Rev Pathol. 2022;17(1):181–204. doi: 10.1146/annurev-pathol-042420-093238
- Kudelova E, Smolar M, Holubekova V, et al. Genetic heterogeneity, tumor microenvironment and immunotherapy in triple-negative breast cancer. Int J Mol Sci. 2022;23(23):14937. doi: 10.3390/ijms232314937
- Li Y, Zhang H, Merkher Y, et al. Recent advances in therapeutic strategies for triple-negative breast cancer. J Hematol Oncol. 2022;15(1):121. doi: 10.1186/s13045-022-01341-0
- Colleoni M, Gray KP, Gelber S, et al. Low-dose oral cyclophosphamide and methotrexate maintenance for hormone receptor–negative early breast cancer: international breast cancer study group trial 22-00. J Clin Oncol. 2016;34(28):3400. doi: 10.1200/JCO.2015.65.6595
- Wei CW, Yu YL, Chen YH, et al. Anticancer effects of methotrexate in combination with α‑tocopherol and α‑tocopherol succinate on triple‑negative breast cancer. Oncol Rep. 2019;41(3):2060–2066. doi: 10.3892/or.2019.6958
- Yang V, Gouveia MJ, Santos J, et al. Breast cancer: insights in disease and influence of drug methotrexate. RSC Med Chem. 2020;11(6):646–664. doi: 10.1039/D0MD00051E
- Bai X, Ni J, Beretov J, et al. Cancer stem cell in breast cancer therapeutic resistance. Cancer Treat Rev. 2018;69:152–163. doi: 10.1016/j.ctrv.2018.07.004
- Mehraj U, Ganai RA, Macha MA, et al. The tumor microenvironment as driver of stemness and therapeutic resistance in breast cancer: new challenges and therapeutic opportunities. Cell Oncol. 2021;44(6):1–21. doi: 10.1007/s13402-021-00634-9
- Pan G, Liu Y, Shang L, et al. EMT‐associated microRnas and their roles in cancer stemness and drug resistance. Cancer Commun. 2021;41(3):199–217. doi: 10.1002/cac2.12138
- Lim B, Woodward WA, Wang X, et al. Inflammatory breast cancer biology: the tumour microenvironment is key. Nat Rev Cancer. 2018;18(8):485–499. doi: 10.1038/s41568-018-0010-y
- Peng C-Y, Yu C-C, Huang C-C, et al. Magnolol inhibits cancer stemness and IL-6/Stat3 signaling in oral carcinomas. J Formosan Med Assoc. 2022;121(1):51–57. doi: 10.1016/j.jfma.2021.01.009
- Tang H, Zhang Y, Li D, et al. Discovery and synthesis of novel magnolol derivatives with potent anticancer activity in non-small cell lung cancer. Eur J Med Chem. 2018;156:190–205. doi: 10.1016/j.ejmech.2018.06.048
- Wang Y, Sun C, Huang L, et al. Magnolol-loaded cholesteryl biguanide conjugate hydrochloride nanoparticles for triple-negative breast cancer therapy. Int J Pharmaceut. 2022;615:121509. doi: 10.1016/j.ijpharm.2022.121509
- Zhao M, Zheng Y-H, Zhao Q-Y, et al. Synthesis and evaluation of new compounds bearing 3-(4-aminopiperidin-1-yl) methyl magnolol scaffold as anticancer agents for the treatment of non-small cell lung cancer via targeting autophagy. Eur J Med Chem. 2021;209:112922. doi: 10.1016/j.ejmech.2020.112922
- Li Y-C, Wong C-N, Hsu F-T, et al. Accessing apoptosis induction and metastasis inhibition effect of magnolol on triple negative breast cancer in vitro. In Vivo. 2023;37(3):1028–1036. doi: 10.21873/invivo.13177
- Liu Y, Cao W, Zhang B, et al. The natural compound magnolol inhibits invasion and exhibits potential in human breast cancer therapy. Sci Rep. 2013;3(1):3098. doi: 10.1038/srep03098
- Kundu M, Das S, Das CK, et al. Magnolol induces cytotoxic autophagy in glioma by inhibiting PI3K/AKT/mTOR signaling. Exp Cell Res. 2023;424(1):113488. doi: 10.1016/j.yexcr.2023.113488
- Wang Y-D, Sun X-J, Yang W-J, et al. Magnolol exerts anticancer activity in hepatocellular carcinoma cells through regulating endoplasmic reticulum stress-mediated apoptotic signaling. Onco Targets Ther. 2018;Volume 11:5219–5226. doi: 10.2147/OTT.S168887
- H-B L, Yi X, J-M G, et al. Magnolol-lnduced H460 cells death via autophagy but not apoptosis. Arch Pharm Res. 2007;30(12):1566–1574. doi: 10.1007/BF02977326
- Tan L, Ma B, Chen L, et al. Toxicity evaluation and anti-tumor study of docetaxel loaded mPEG-polyester micelles for breast cancer therapy. J Biomed Nanotechnol. 2017;13(4):393–408. doi: 10.1166/jbn.2017.2356
- Kurd M, Sadegh Malvajerd S, Rezaee S, et al. Oral delivery of indinavir using mPEG-PCL nanoparticles: preparation, optimization, cellular uptake, transport and pharmacokinetic evaluation. Artific Cells Nanomed Biotechnol. 2019;47(1):2123–2133. doi: 10.1080/21691401.2019.1616553
- Chen B, He X-Y, Yi X-Q, et al. Dual-peptide-functionalized albumin-based nanoparticles with ph-dependent self-assembly behavior for drug delivery. ACS Appl Mater Inter. 2015;7(28):15148–15153. doi: 10.1021/acsami.5b03866
- Tian X, He X, Yao A, et al. The properties of cholesterol-modified pullulan nanoparticles with different PEG coatings and their anti-hepatoblastoma cell effects. Mater Technol. 2022;37(12):2276–2288. doi: 10.1080/10667857.2022.2029288
- Saeednia L, Yao L, Cluff K, et al. Sustained releasing of methotrexate from injectable and thermosensitive chitosan–carbon nanotube hybrid hydrogels effectively controls tumor cell growth. ACS Omega. 2019;4(2):4040–4048. doi: 10.1021/acsomega.8b03212
- Fathi M, Barar J, Erfan-Niya H, et al. Methotrexate-conjugated chitosan-grafted pH-and thermo-responsive magnetic nanoparticles for targeted therapy of ovarian cancer. Int j biol macromol. 2020;154:1175–1184. doi: 10.1016/j.ijbiomac.2019.10.272
- Chen C, Zhong W, Du S, et al. Intelligent nanotherapeutic strategies for the delivery of CRISPR system. Acta Pharm Sin B. 2022;13(6):2510–2543. doi: 10.1016/j.apsb.2022.12.013
- Mohanty A, Uthaman S, Park I-K. Utilization of polymer-lipid hybrid nanoparticles for targeted anti-cancer therapy. Molecules. 2020;25(19):4377. doi: 10.3390/molecules25194377
- Zhang N-N, Shen X, Liu K, et al. Polymer-Tethered nanoparticles: from surface engineering to directional self-assembly. Acc Chem Res. 2022;55(11):1503–1513. doi: 10.1021/acs.accounts.2c00066
- Tuncel D, Demir HV. Conjugated polymer nanoparticles. Nanoscale. 2010;2(4):484–494. doi: 10.1039/b9nr00374f
- Soares DCF, Domingues SC, Viana DB, et al. Polymer-hybrid nanoparticles: Current advances in biomedical applications. Biomed Pharmacother. 2020;131:110695. doi: 10.1016/j.biopha.2020.110695
- Cao Z, Liu R, Li Y, et al. MTX-PEG-modified CG/DMMA polymeric micelles for targeted delivery of doxorubicin to induce synergistic autophagic death against triple-negative breast cancer. Breast Cancer Res. 2023;25(1):1–15. doi: 10.1186/s13058-022-01599-9
- Abolmaali SS, Zarenejad S, Mohebi Y, et al. Biotin receptor-targeting nanogels loaded with methotrexate for enhanced antitumor efficacy in triple-negative breast cancer in vitro and in vivo models. Int J Pharmaceut. 2022;624:122049. doi: 10.1016/j.ijpharm.2022.122049
- Lin H-L, Cheng W-T, Chen L-C, et al. Honokiol/magnolol-loaded self-assembling Lecithin-based mixed polymeric micelles (lb MPMs) for improving solubility to enhance oral bioavailability. Int J Nanomed. 2021;16:651–665. doi: 10.2147/IJN.S290444
- Zheng Y, Ye J, Zhang Z, et al. A new type of glutathione-responsive anti-osteosarcoma prodrug nanoparticles. Mater Technol. 2022;37(9):953–961. doi: 10.1080/10667857.2021.1908769
- Shi P, Cheng Z, Zhao K, et al. Active targeting schemes for nano-drug delivery systems in osteosarcoma therapeutics. J Nanobiotechnol. 2023;21(1):1–27. doi: 10.1186/s12951-023-01826-1
- Kang H, Kim J-D, Han S-H, et al. Self-aggregates of poly (2-hydroxyethyl aspartamide) copolymers loaded with methotrexate by physical and chemical entrapments. JControlled Release. 2002;81(1–2):135–144. doi: 10.1016/S0168-3659(02)00058-5
- Wu Y, He L, Zhou H. Preparation of hydrophobically modified carboxylated pullulan nanoparticles for evaluating the effect of hydrophobic substitution on the properties and functions of nanoparticles. Mater Technol. 2022;37(10):1467–1477. doi: 10.1080/10667857.2021.1956230
- Shi J, Yang X, Li Y, et al. MicroRNA-responsive release of Cas9/sgRNA from DNA nanoflower for cytosolic protein delivery and enhanced genome editing. Biomaterials. 2020;256:120221. doi: 10.1016/j.biomaterials.2020.120221
- Kumari P, Ghosh B, Biswas S. Nanocarriers for cancer-targeted drug delivery. J Drug Targeting. 2016;24(3):179–191. doi: 10.3109/1061186X.2015.1051049