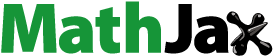
ABSTRACT
To enhance the efficiency of painless targeted nanotherapies, integrating optical imaging capabilities into nanoplatforms is gaining momentum. Our study presents a cost-effective synthesis of MoS2 nanoflower (MNF) and Indocyanine green (ICG) composites (MNF-ICG) using a physical method based on van der Waals interactions. MNF, prepared via the hydrothermal method, underwent characterization through UV-vis, FTIR, and fluorescence spectroscopy, while FESEM and XRD techniques were employed to analyse morphology and structural phase. Through electrostatic interaction, ICG was absorbed onto the MNF surface. The resulting MNF-ICG demonstrated exceptionally high optical absorbance across a broad NIR spectra, facilitating longer-wavelength photothermic imaging with increased penetration depth. In comparison to MNF alone, the composite significantly enhanced the photothermal imaging sensitivity of nanoplatforms, exhibiting notable bathochromic and hyperchromic shifts in fluorescence intensity. This innovative approach holds promise for advancing nanotherapeutics with improved optical imaging capabilities.
Introduction
The work on fluorescent and near-infrared (NIR) imaging has been of high interest in imaging methods and in vivo diagnostics. The optical agents having emission and excitation wavelengths in the range of 700 to 1450 nm match the optical imaging window, within which minimum absorbance and autofluorescence from blood and tissue are found [Citation1,Citation2]. Indocyanine green (ICG), also called Cardio Green, is an a clinical imaging dye approved by the FDA and clinicians for use in therapeutic applications as a photosensitizer and in the treatment and diagnosis of theranostic agents [Citation3]. Most frequently, nanocarriers (NCs) in biomedical applications are used as delivery agents by functionalizing the surface of the NC with a fluorescent dye [Citation4,Citation5,Citation6,Citation7,Citation8,Citation9,Citation10]. Thus, there is a significant interest in integrating ICG into nanoscale transporters or nanoplatforms.
Transition metal dichalcogenides (TMDs) have huge potential in biomedical applications because of their unique physical and chemical properties. Molybdenum disulfide (MoS2) is one of the most widely explored TMDs, having a large surface area due to its layered structure and high NIR light adsorption efficiency. The above properties make MoS2 an effective platform for incorporating NIR-sensitive photothermal contrast specialists by adsorbing fluorescent dyes onto its surface [Citation11,Citation12]. In our previous study, we synthesized MoS2 nanostructures via hydrothermal and liquid-phase exfoliation methods using different organic solvents [Citation13]. Herein, we report the synthesis of Molybdenum disulfide nanoflowers (MNF) using the hydrothermal route and then foster an a non-covalent system for the development of a MNF-ICG nanoplatform.
Materials &Methods
Ammonium heptamolybdate tetrahydrate (NH4)6Mo7O24.4 H2O and thiourea (NH2)2CS were purchased from Merck Chemicals. ICG (Cardiogreen) was purchased from Sigma-Aldrich (I2633). Citric acid monohydrate was purchased from Merck Chemicals and was used as a surfactant. Hydrothermal treatment was carried out in a high temperature furnace (model NO. AI-175). We used REMI R-8C PLUS for centrifugation. Bath sonication was performed through an a bath sonicator with an ultrasonic power of 180 W at a frequency of 40 kHz. A thin film coating was formed with the use of an a spin coater, spin NXG-P1. Millipore water was used during the entire synthesis and characterization process.
For characterization, an X-ray diffractometer (model: SMARTLAB), RIGAKU, Japan, with a source of Cu-Kα, generating the X-rays having a wavelength of 1.54 and diffraction angle (2θ) ranging from 0–80◦ was used to investigate the as-prepared MNF crystal structure. A UV-vis spectrophotometer was used to examine the optical absorbance (Shimazu UV-2600). Various bond formations, stretching, and vibrations of characteristic peaks of MNF, free ICG, and MNF-ICG composite were studied and examined using FTIR spectroscopy (Fourier Transform Infra-Red spectrometer, Bruker ALPHA-II) with an a spectral range of (0–3000) cm−1. The fluorescence properties of the material were measured using a Horiba Fluoro Max-4 fluorescence spectrometer. The morphology and element analysis of MNF and MNF-ICG surfaces were examined using a JEOL-JSM-7610FPlus FESEM and an EDAX Energy Dispersive X-ray (EDS) detector combined with a FESEM chamber. The Origin software (version 22) was used to plot various graphs used in the analyses.
Synthesis of MNF
For hydrothermal synthesis of MoS2, we used thiourea as a source of sulphur and ammonium molybdate tetrahydrate as a source of molybdenum. 1.639 g of ammonium heptamolybdate tetrahydrate (NH4)6Mo7O24.4 H2O was mixed in 50 ml of millipore water. To this solution, 0.6125 g of citric acid monohydrate powder was added and stirred for 30 min at 450 rpm and at a constant temperature of 60◦ °C. Subsequently, 1.58 g of thiourea, (NH2)2CS, was dissolved in 20 ml of water and mixed with the prepared (NH4)6Mo7O24.4 H2O solution. This solution was again stirred for 30 minutes. A teflon-lined stainless-steel vessel was filled with the prepared transparent homogeneous solution and closed by tightening the bolts of the autoclave. This autoclave was then placed in a high temperature furnace at a constant temperature of 180◦ C for 24 hours. After 24 hours, the autoclave was taken out of the furnace and allowed to cool naturally. The dark grey solution was collected and centrifuged at 5000 rpm for 15 min. It was then washed with ethanol multiple times to filter out the residual ions and impurities. Finally, it was dried at 80°C in a hot air oven for 12 hours. 0.80 g of MNF powder was collected and stored for further characterization and conjugation with ICG [Citation14].
Synthesis of MNF-ICG composite
5 mg of MNF and 0.5 mg of ICG (C43H47N2NaO6S2) was mixed with millipore water and made up to a final volume of 5 ml. The mixture went through continuous stirring for 24 h at room temperature at 550 rpm, for the highest possible loading of ICG into MNF via induced dipole interaction. The ICG loaded MNF (MNF-ICG composite) was collected through centrifugation (30 min/8000rpm) and was subsequently dried and stored at 4◦C. The residual containing unbound ICG was also collected to evaluate the loading efficiency of ICG. The ICG loading was carried out entirely in the absence of any direct illumination ().
ICG loading efficiency
UV-vis spectroscopy was used to evaluate the loading efficacy of ICG. The optical absorbance of the MNF-ICG solutions (residual after centrifugation) with different feeding ratios (1:10, 2:10, and 3:10) was observed with a UV – vis spectrometer at the characteristic wavelength of 780 nm for ICG. On comparing with the calibration curve, the amount of unbound ICG was calculated. After subtracting the amount of unbound ICG from the total amount of ICG taken initially, we got the amount of loaded ICG. The percentage loading efficiency was calculated as W1/W2 *100, where W1 represents the weight of the loaded ICG with MNF and W2 represents the weight of MNF initially taken.
Result & discussion
A combination of crystallography, mechanisms, and data analysis from different characterization techniques can be used to explain how the different parts of the synthesis process work together in the formation of MNF and MNF-ICG. Here are four possible chemical reactions that could happen during the hydrothermal treatment at high temperature and pressure.
At first, thiourea produced hydrogen sulphide (H2S) and other gases. On the other hand, oxidation of Mo in the form of MoO3 (molybdenum trioxide) happened due to the reduction of H24Mo7N6O24.4 H2O (ammonium molybdate). On reacting MoO3 with Hydrogen sulphide, it converts into molybdenum dioxide first and then finally reduces to molybdenum sulphide. The tiny MoS2 nanoparticles act as nucleation sites, and on growing they produce the familiar molybdenum disulphide nanosheets. The 3D nanoflowers (MNF) are then created by the self-assembly of these two-dimensional sheets. As prepared, MNF crystals were loaded with ICG via physical adsorption.
The ICG loading efficiency was calculated at its maximum (77.7%) for the ICG: MNF concentration ratio (1:10) as seen in . Subsequently, a ratio of 1:10 (ICG: MNF) was preferred for further experiments.
The XRD pattern of MNF shown in and was recorded using the X-ray diffractometer (model: SMARTLAB), RIGAKU, Japan) with Cu-Kα source. In accordance with the reference JCPDS file (PDF: 00-037-1492), four diffraction peaks at 2θ values of 14.00◦, 32.69◦, 35.59◦ and 57.91◦ were found corresponding to the (002), (100), (102), and (110) lattice planes, respectively. This confirms the hexagonal structure of MNF, having lattice constants as a = b = 0.3161 nm and c = 1.2299 nm [Citation15–17]. The absence of any additional intense peaks confirms the purity of 2 H phase in the hydrothermally prepared MNF. The high intensity of the peaks also confirms the crystallinity of the material. Interestingly, in the XRD pattern, the peak corresponding to the (002) lattice planes displayed the highest diffraction strength, confirming that the long-range stacking of the (002) layers along the c-axis dominates the structure. To quantitatively assess the number of stacked MoS2 layers, the intensity ratio between the (002) and (100) peaks was used [Citation18]. The measured ratio in this case was 1.32, which is consistent with the stacking of MoS2 layers. The creation of MoS2 nanoscale crystallites is indicated by the broad diffraction peaks. The Scherrer’s equation was used to determine the crystal dimension (D) of MNF, and the results are shown in . The measured crystallite size was between 2.56 nm and 5.78 nm, with an average crystallite size of about 3.80 nm. These MoS2 planes are layered over one another to form a single crystallite.
Table 1. XRD parameters and crystal dimensions of MNF powder.
For MNF, the typical adsorption peaks were seen at 605, 660, and 750 nm (). However, after the loading of ICG, the optical characteristics of MNF underwent a significant change [Citation19]. The MNF-ICG has a significantly higher optical absorbance than MNF at the peak wavelength (780 nm) due to the absorbance of ICG by MNF [Citation20]. The absorbance intensity of MNF-ICG was 1.9 times more than of MNF with the same concentration. The hybrid also had a larger NIR adsorption spectrum than MNF, with the absorbance peak being redshifted by 30 nm (from 750 to 780 nm). The redshift of the absorbance peak and the broadening of NIR spectrum confirms the adsorption of ICG onto the MNF surface, which is probably the result of electrostatic interaction between MNF and ICG.
The formation of different chemical bonds and the presence of functional groups can be detected using FTIR spectroscopy. shows the FTIR transmittance spectra of MNF, which reveals the distinctive peaks of transmission at 570 cm−1,592 cm−1 907 cm−1, 1045 cm−1, 1720 cm−1, and 3000 cm−1. The weak transmission band at 570 cm−1 is a result of Mo-S bond stretching vibrations, which indicate the development of MNF crystals. The strong transmission band 592 cm−1 is due to the bridging of two ligands of S22- . The S-S stretching vibrational mode is characterized by the strong band that appears at 907 cm−1. The substantial transmission bands at 1045 cm−1 and 1622 cm−1 are attributable to the presence of carbonyl (C=O) groups and C-O groups, respectively. The existence of water molecules absorbed on the surface of MNF shows the transmission peak of hydroxyl (−OH) group at 3000 cm−1. The FTIR spectra of free ICG shows that C-H is out of plane at 600–1000 cm−1, aromatic C=O stretches at 1400–1500 cm−1. The C-O-H bond on benzene ring gives two peaks at 1150 cm−1 and 1390 cm−1 due to the bending and tensile vibrations, respectively. After the adsorption of ICG on MNF, the Mo-S bond shows vibration peaks at 657 cm−1 and ethylene stretching of ICG at 2300 cm−1. In addition, the vinyl lengths of ICG could be responsible for the presence of a distinctive peak at 1093 cm−1.
The fluorescence spectra (FL)of the synthesized MNF, MNF-ICG and free ICG are shown in . To analyse the changes in fluorescence property, spectra were observed at excitation wavelengths according to characteristics adsorption wavelengths. The FL spectrum of MNF and MNF-ICG at excitation wavelength 700 nm represents almost 44% enhancement in fluorescence of MNF due to the adsorption of ICG. On the other hand, the FL spectrum of free ICG and MNF-ICG at excitation wavelength 780 nm represents the fluorescence quenching in free ICG of almost 45% (4d). The FL quenching is due to the aggregation of ICG with MNF through FRET (Fluorescence/Förster resonance energy transfer) photothermal effect [Citation21]. Fluorescence resonance energy transfer occurs when an excited molecule fluorophore (ICG-the donor) transfers energy non-radiatively to another fluorophore (MNF-the acceptor) via an intermolecular long-range dipole-dipole coupling phenomenon. When a specimen (MNF-ICG) containing both donor (ICG) and acceptor (MNF) molecules is excited with light at wavelengths that match the donor fluorophore’s adsorption maximum (780 nm in the case of donor ICG), the FRET phenomena can be expected by detecting light that is emitted at wavelengths close to the acceptor’s emission maximum (820 nm in the case of acceptor MNF) [Citation22–24].
The FESEM images of the surface of the as prepared material demonstrate the effective fabrication of thin nanosheets self-assembled MoS2 nanoflowers. The low and highly resolved FESEM images of the MNF structures are displayed individually in . The less-resolved image () depicts a homogeneous flower-shaped morphology produced by the aggravation of thin nanosheets. The high-magnification FESEM image () shows a relatively close-up view of nanoflowers consisting of several thin, plate-like nanosheets that are well-separated and the thickness ranges from 5 to 20 nm. It was observed that the nanosheets have an intriguing tendency to self-assemble, resulting in the flower-like MoS2 nanostructures (MNF) shown (). The EDS spectrum (not shown here) of MNF structures confirms the presence of Mo and S thereby confirming the phase purity and correlating with the findings of the XRD. The atomic content ratio of molybdenum to sulphur, which is 9.1/20.8 or approximately 1:2, confirms the stoichiometry of MoS2. The FESEM images of the surface morphology of MNF-ICG composite were also analysed. Physical adsorption of ICG on the MNF surface was confirmed by uniform thick layers of ICG in the shape of grids (). The existence of plentiful mesoporous structures enables the adsorption of ICG on MNF through physical forces. A good amount of ICG can also be absorbed through the pores of the nanoflower ( ).
Figure 7. a) the less resolved FESEM image of homogeneous flower-shaped MoS2 nanosheets. b,c) the close-up views of nanoflowers consisting of several well-separated nanosheets. d) FESEM image of MNF-ICG hybrid represents uniform thick layers of ICG on MNF.E,f) physically absorbed ICG between MoS2 nanosheets through the pores of the nanoflower’s structure. g,h) EDS spectrum represents percentage and graphical elements content.

Conclusions
Herein, we report the synthesis and analysis of a MoS2 nanoplatform-based MNF-ICG composite. We investigated the crystallographic, morphological, structural, optical, and fluorescent properties of the MNF-ICG composite. Hydrothermal technique was used to successfully produce flower-like nanostructures. The surface morphology of MoS2 nanoflowers (MNF) constructed from thin layer nanosheets was studied at the nanoscale, by FESEM micrographs with typical thickness of around 5 to 20 nm. From XRD spectrum analysis, we geo the hexagonal form of the MNF crystals with an average crystallite size of about 4 nm. The formation of MoS2 was confirmed by EDS analysis as the Mo/S ratio of about 1:2 was close to the Mo/S stoichiometric ratio. In the FTIR spectrum, stretching vibrations of S-S and Mo-S bonds were found to be responsible for the formation of crystalline MoS2. Indocyanine green (ICG), an optical dye, was loaded onto MNF to give fluorescent properties to the nanoplatform. The negatively charged ICG was then adsorbed on the surface of the positively charged MNF through an electrostatic interaction. The vibration peaks of the Mo-S bond at 657 cm−1, the ethylene stretching peak at 2300 cm−1, and the characteristic peak at 1093 cm−1 due to vinyl stretches of ICG in the FTIR spectrum confirm the successful adsorption of optical dye on MNF. FESEM images show the presence of grid-type thick layers of ICG on MNF, which confirms the presence of Van der-Wall force in the formation of the MNF-ICG composite. Broad absorbance was seen in the case of MNF-ICG at 770–900 nm, and typical peaks were found around 780 nm. The redshift phenomena result from the development of ICG aggregates. The FL-enhancement of MNF-ICG and FL quenching of optical dye (ICG) via fluorescence resonance energy transfer (FRET) also confirms ICG adsorption into MNF.
Future scope
The well-defined nanoscale crystalline structure of MoS2 nanoflowers have potential uses in a number of fields, including electronic devices [Citation21,Citation25] solar cells [Citation26], water filtration [Citation27] lubricants, sensors [Citation28–30] and electromagnetic wave adsorption [Citation31] Electromagnetic wave shielding is an advanced application of such two-dimensional nanostructures with large specific surface areas and strong carrier suppleness [Citation32]. Non-covalent physical adsorption method used in MNF-ICG hybrid formation would be advantageous in photothermic mechanism as the connection force between ICG and MNF would easily disturb on rising the surrounding temperature. As ICG is a high NIR termination coefficient optical dye, so when ICG is absorbed with MNF, the optical adsorption of MNF-ICG showed red-shifting towards NIR region which is significantly longer than MNF alone. This allows photothermic imaging at a longer wavelength to get larger entrance profundity and reduce back noise. Consistent with previous research in targeted drug delivery applications, a photothermal effect could effectively trigger the accelerated release of a loaded drug, which were loaded on NIR active nanoplatforms [Citation33–37]. Fluorescence enhancement in MNF-ICG would play an important role while using the nanoplatforms in optical imaging and targeted drug delivery applications.
Acknowledgments
Authors are grateful to the CAIF and SAIF facility at Manipal University Jaipur, for characterization of the samples.
Disclosure statement
No potential conflict of interest was reported by the author(s).
References
- Pansare VJ, Faenza WJ, Hoang L, et al. Formulation of long-wavelength indocyanine green nanocarriers. J Biomed Opt. 2017;22(9):096007. doi: 10.1117/1.JBO.22.9.096007
- Pansare VJ, Hejazi S, Faenza WJ, et al. Review of long-wavelength optical and NIR imaging materials: contrast agents, fluorophores, and multifunctional nano carriers. Chem Mater. 2012;24(5):812–8. doi: 10.1021/cm2028367
- Landsman M, Kwant G, Mook GA, et al. Light-absorbing properties, stability, and spectral stabilization of indocyanine green. J Appl Physiol. 1976;40(4):575–583. doi: 10.1152/jappl.1976.40.4.575
- Gunn J, Park SI, Veiseh O, et al. A pretargeted nanoparticle system for tumor cell labeling. Mol Biosyst. 2011;7(3):742–748. doi: 10.1039/C005154C
- Gunn J, Wallen H, Veiseh O, et al. A multimodal targeting nanoparticle for selectively labeling T cells. Small. 2008;4(6):712–715. doi: 10.1002/smll.200701103
- Jarzyna PA, Skajaa T, Gianella A, et al. Iron oxide core oil-in-water emulsions as a multifunctional nanoparticle platform for tumor targeting and imaging. Biomaterials. 2009;30(36):6947–6954. doi: 10.1016/j.biomaterials.2009.09.004
- Jun L, Li J, Rosol TJ, et al. Biodegradable nanoparticles for targeted ultrasound imaging of breast cancer cells in vitro. Phys Med Biol. 2007;52(16):4739–4747. doi: 10.1088/0031-9155/52/16/002
- Koch AM, Reynolds F, Kircher MF, et al. Uptake and metabolism of a dual fluorochrome Tat-nanoparticle in HeLa cells. Bioconjugate Chem. 2003;14(6):1115–1121. doi: 10.1021/bc034123v
- Parikh T, Bommana MM, Squillante E. Efficacy of surface charge in targeting pegylated nanoparticles of sulpiride to the brain. Eur J Pharm Biopharm. 2010;74(3):442–450. doi: 10.1016/j.ejpb.2009.11.001
- Reddy GR, Bhojani MS, McConville P, et al. “Vascular targeted nanoparticles for imaging and treatment of brain tumors,” Clin. Cancer Res. 2006;12(22):6677–6686. doi: 10.1158/1078-0432.CCR-06-0946
- Zhang N, Chittasupho C, Duangrat C, et al. PLGA nanoparticle-peptide conjugate effectively targets intercellular cell-adhesion molecule-1. Bioconjugate Chem. 2008;19(1):145–152.
- Liu B, Li C, Chen G, et al. Synthesis and optimization of MoS2@Fe3O4-ICG/Pt(IV) nanoflowers for MR/IR/PA bioimaging and combined PTT/PDT/Chemotherapy triggered by 808 nm laser. Adv Sci. 2017;4(8):1600540. doi: 10.1002/advs.201600540
- Wang B, Iocozzia J, Zhang M, et al. The charge carrier dynamics, efficiency and stability of two-dimensional material-based perovskite solar cells. Chem Soc Rev. 2019;48(18):4854–4891. doi: 10.1039/C9CS00254E
- Sharma A, Bagaria A. Morphology of MoS2 Nanostructures: Insights into the Solvent Assisted Exfoliation. AIP conference Proceedings. Recent Advances in Material Science and Computational Techniques (RAMSACT-1); 2021 Nov 24–26; Manipal University Jaipur, Rajasthan, India. AIP Publishing; 2023.
- Liu Y, Kang N, Lv J, et al. Deep photothermic/luminescence/magnetic resonance multimodal imaging in living subjects using high-efficiency upconversion nanocomposites. Adv Mater. 2016;28(30):6411–6419. doi: 10.1002/adma.201506460
- Wang XH, Peng HS, Yang W, et al. Indocyanine green-platinum porphyrins integrated conjugated polymer hybrid nanoparticles for near-infrared-triggered photothermal and two-photon photodynamic therapy. J Mater Chem B. 2017;5(9):1856–1862. doi: 10.1039/C6TB03215J
- Kumar N, Siroha P, Sharma Y, et al. Probing on crystallographic structural and surface morphology of hydrothermally synthesized MoS2 nanoflowers consisting of nanosheets. Appl Surf Sci. 2021;6:100167. doi: 10.1016/j.apsadv.2021.100167
- Aljarb A, Fu JH, Hsu CC, et al. Ledge-directed epitaxy of continuously self-aligned single-crystalline nano ribbons of transition metal dichalcogenides. Nat Mater. 2020;19(12):1300–1306. doi: 10.1038/s41563-020-0795-4
- Chengbo Liu1. Jingqin Chen1,2. Ying Zhu1. Xiaojing Gong1. Rongqin Zheng3. Ningbo Chen1. Dong Chen1. Huixiang Yan1. Peng Zhang5. Hairong Zheng4. Zonghai Sheng4. Liang Song1, Highly Sensitive MoS2–Indocyanine Green Hybrid for Photothermic Imaging of Orthotopic Brain Glioma at Deep Site. Nano-Micro Lett. 2018;10:48.
- Chen J, Liu C, Zeng G, et al. Indocyanine green loaded reduced graphene oxide for in vivo photothermic/fluorescence dual-modality tumor imaging. Nanoscale Res Lett. 2016;11(1):85. doi: 10.1186/s11671-016-1288-x
- Marega GM, Zhao Y, Avsar A, et al. Logicin-memory based on an atomically thin semiconductor. Nature. 2020;587(7832):72–77. doi: 10.1038/s41586-020-2861-0
- Hu D, Zhang J, Gao G, et al. Indocyanine green-loaded polydopamine-reduced graphene oxide nanocomposites with amplifying photothermic and photothermal effects for cancer theranostics. Theranostics. 2016;6(7):1043–1052. doi: 10.7150/thno.14566
- Cayre F, Mura S, Andreiuk B, et al. In vivo FRET imaging to predict the risk associated with hepatic accumulation of squalenebased prodrug nanoparticles. Adv Healthc Mater. 2018;7(3):1700830. doi: 10.1002/adhm.201700830
- Vattikuti SVP, Byon C. Synthesis and characterization of molybdenum disulfide nano flowers and nanosheets: nanotribology. J Nanomater. 2015;2015:1–11. doi: 10.1155/2015/710462
- Lin Z, Liu Y, Halim U, et al. Solution-processable 2D semiconductors for high performance large-area electronics. Nature. 2018;562(7726):254–258. doi: 10.1038/s41586-018-0574-4
- Liang M, Ali A, Belaidi A, et al. Improving stability of organometallic-halide perovskite solar cells using exfoliation two-dimensional molybdenumchalcogenides. npj 2D Mater Appl. 2020;4(1):40. doi: 10.1038/s41699-020-00173-1
- Gopal R, Chinnapan MM, Bojarajan AK, et al. Facile synthesis and defect optimization of 2D-layered MoS2 on TiO2 heterostructure for industrial effluent, wastewater treatments. Sci Rep. 2020;10(1):21625. doi: 10.1038/s41598-020-78268-4
- Cao MS, Wang XX, Zhang M, et al. Electromagnetic response and energy conversion for functions and devices in lowdimensional materials. Adv Funct Materials. 2019;29(25):1807398. doi: 10.1002/adfm.201807398
- Lei Y, Butler D, Lucking MC, et al. Single-atom doping of MoS2 with manganese enable sultra sensitive detection of dopamine: experimental and computational approach. Sci Adv. 2020;6(32):eabc4250. doi: 10.1126/sciadv.abc4250
- Surya S, Yuvaraja S, Varrla E, et al. An infield integrated capacitive sensor for rapid detection and quantification of soil moisture, Sens. Actuators B Chem. 2020;321:128542. doi: 10.1016/j.snb.2020.128542
- Chai J, Cheng J, Zhang D, et al. Enhancing electromagnetic wave absorption performance of Co3O4 N. Kumar et al. Applied surface science advances 6 (2021) 100167 8 nanoparticles functionalized MoS2 nanosheets. J Alloy Compd. 2020;829:154531. doi: 10.1016/j.jallcom.2020.154531
- Zhang M, Cao MS, Shu JC, et al. Electromagnetic absorber converting radiation for multifunction, Mater. Sci Eng R. 2021;145:100627. doi: 10.1016/j.mser.2021.100627
- Ding T, Xing YX, Wang ZQ, et al. Structural complementarity from DNA for directing two-dimensional polydopamine nanomaterials with biomedical applications. Nanoscale Horiz. 2019;4:652–657. doi: 10.1039/C8NH00351C
- Gao G, Jiang YW, Jia HR, et al. Near-Infrared LightControllable on-Demand Antibiotics Release using ThermoSensitive Hydrogel-based drug Reservoir for combating bacterial infection. Biomaterials. 2019;188:83–95. doi: 10.1016/j.biomaterials.2018.09.045
- . Huiling Jianga C, Yilin D, Leilei C, et al. Multimodal theranostics augmented by transmembrane polymer-sealed nano-enzymatic porous MoS2 nanoflowers. doi: 10.1016/j.ijpharm.2020.119606
- Wan MM, Chen H, Wang Q, et al. Bio-Inspired Nitric-Oxide-Driven Nanomotor. Nat Commun. 2019;10(1):966. doi: 10.1038/s41467-019-08670-8
- Younis MR, Wang C, An R, et al. Low power single laser activated synergistic cancer phototherapy using photosensitizer functionalized dual plasmonic photothermal nanoagents. ACS Nano. 2019;13:2544–2557. doi: 10.1021/acsnano.8b09552