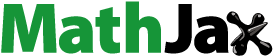
ABSTRACT
In this paper, the atomic model of high-entropy alloy is established by molecular dynamics simulation. By applying nano-indentation, the influence of Cu atomic ratio and temperature on the deformation of FeCoCrCuNi high-entropy alloy was studied, and the micro-scale dislocation movement evolution mechanism was obtained. The results show that, with the increase of temperature, the load of FeCoCrCuNi high-entropy alloy under nano indentation decreases. The total length of dislocations in each stage of nanoindentation decreases monotonically, and the types of dislocation reactions decrease. Under the action of high temperature, the number of perfect dislocations decomposed into Shockley partial dislocations decreases, resulting in more Frank dislocations and Stair-rod dislocations, which makes the extended dislocations recombine into perfect dislocations, and the length of dislocations decreases sharply. The dislocation defect is replaced by a cavity at high temperature, which makes the high-entropy alloy lose elastoplastic mechanical properties.
Introduction
In recent years, the appearance of high-entropy alloys [Citation1,Citation2] provides a new way to solve the constraint between strength and toughness of metal materials. The multi principal component alloy design concept and high mixing entropy effect of high-entropy alloy [Citation3–5] breakthrough the traditional design idea of single principal component alloy. Researchers can meet different application needs by changing the element type, composition ratio, heat treatment and other processes. High-entropy alloys show excellent mechanical properties, such as high strength and toughness, which are different from traditional alloys [Citation6–8], so they have been paid more attention in the industrial field [Citation9–11]. Among them, FeCoCrCuNi high-entropy alloy has been proven by experiments to have excellent mechanical properties [Citation12–14] and has potential and application value in the industrial field. For example, Gong and Feng et al. [Citation15,Citation16] designed coatings for high-entropy alloys, Maldonado et al. [Citation17] studied grain boundary segregation in high-entropy alloys, Tan et al. [Citation18] prepared high-entropy alloy thin films using magnetron sputtering method. However, the dynamic mechanical properties and microstructure evolution mechanism of FeCoCrCuNi high-entropy alloy under plastic deformation are still unclear, which seriously restricts its application in the industrial field.
The mechanical properties of high-entropy alloys can be analysed by nanoindentation experiments. Nano indentation technology [Citation19–21] is to control the continuous change of load through a computer program and measure the indentation depth in real time. Nano indentation technology is mainly used to quantitatively characterise the mechanical properties of different materials on the nano scales, such as load–displacement curve, elastic modulus, hardness, fracture toughness, strain hardening effect, creep behaviour, etc.
Although the macroscopic mechanical properties of materials can be obtained by nanoindentation experiments, the internal microstructure of atoms is the internal fundamental factor that determines the mechanical properties of metal materials. Therefore, it is necessary to reveal the deformation response mechanism of high-entropy alloy under loading from the aspects of element atomic effect, phase transformation and defect evolution at the micro scale. However, the evolution of atomic microstructure is very difficult to be observed directly by experimental means, which seriously restricts the development of the research on the mechanical response mechanism of high-entropy alloy materials. Molecular dynamics simulation can be used as an effective tool to make up for the shortcomings of experiments due to its intrinsic atomic tracking ability [Citation22]. Therefore, the nanoindentation process can be simulated by molecular dynamics simulation method, which is helpful to understand the plastic behaviour in such cases [Citation23,Citation24].
Unfortunately, up to now, there has been little research on the simulation of the microstructure evolution of high-entropy alloy nanoindentation, and microscale research on the effect of temperature on high-entropy alloys is even rarer. Li et al. [Citation25] simulated the indentation of FeCrCuAlNi alloy and found that its hardness was high, which was attributed to the low stacking fault energy (SFE) and the dense atomic arrangement in the slip plane. Wang et al. [Citation26] studied the nano scratches of AlCrCuFeNi alloy and found that the high dislocation density obtained under the scratches led to significant plastic activity. Huang [Citation27] studied the tribological behaviour of FeCoCrCuNi alloy and found work hardening and stick slip behaviour. Iyad et al. [Citation28] studied the nano indentation of CoCrFeMnNi high-entropy alloy and found that the plastic zone of the high-entropy alloy is much smaller than that of nickel. However, due to the high dislocation density, the fracture of the twin interface is stronger than that of nickel.
The research on nano-indentation of FeCoCrCuNi high-entropy alloy material mainly includes Mu et al. [Citation29] evaluated the effect of copper segregation and precipitation on the indentation behaviour of FeCoCrCuNi from the aspects of force displacement relationship, dislocation propagation and shear strain distribution. Tian et al. [Citation30] compared the nano indentation behaviour of single crystal and polycrystalline FeCoCrCuNi high-entropy alloys by simulation method and revealed the important role of twin boundary on the plastic deformation of high-entropy alloys from the atomic scale. Feng et al. [Citation31] studied the effect of interface and layer number on the indentation response of nano copper/FeCoCrCuNi high-entropy alloy nano laminates observed in the experiment by molecular dynamics simulation. It is found that the stacking fault strengthening is very obvious, and a large number of Shockley partial dislocations are generated, which makes the material have good plasticity. Zhang et al. [Citation32] Studied the mechanical response of FeCoCrCuNi high-entropy alloy with single crystal fcc structure through in-situ SEM nanoindenter system, and found that the yield strength of the material under elastic axial compression is related to the size, but the sensitivity is lower than that of typical metal micro nanostructures. The influence of temperature on the nanoindentation process is not considered in the above documents.
In order to explore the influence of microscale structural evolution on macroscopic mechanical properties, molecular dynamics simulation method has been used to apply nanoindentation process to the high-entropy alloy atomic model. The plastic deformation law of FeCoCrCuNi high-entropy alloy has been simulated at different temperatures, and the dislocation motion evolution mechanism of FeCoCrCuNi high-entropy alloy has been analysed.
Method
The nanoindentation atomic model of FeCoCrCuNi high-entropy alloy was established as shown in . The atoms of the five elements are randomly distributed on the lattice points of the fcc structure at an atomic ratio of 20%. The maximum value of lattice constant in five single crystals of Fe, Ni, Co, Cr and Cu is selected as the lattice constant of FeCoCrCuNi high-entropy alloy, and the [100], [010] and [001] directions of the crystal are selected as the x, y and z directions of the simulation box. The simulation box has a lateral dimension of 15.1 nm, a depth of 12.3 nm and contains 220,342 atoms. The simulation time step is 1 fs. Embedded atom method (EAM) potential function [Citation33] is selected to describe the interaction force between five elements. The atomic layer of the substrate at the bottom of the model has been fixed to prevent any translational or rotational movement of the entire substrate.
In order to more realistically simulate the physical and chemical properties of high-entropy alloys, the atomic model is pretreated as follows according to the preparation process of high-entropy alloys with high-temperature melting, cooling and annealing [Citation34,Citation35]: First, the model was heated and melted. All atoms in the system were heated to 1500k at a rate of 0.06k/fs, and the relaxation reached equilibrium under the NVT ensemble. Then the quenching process was simulated. the temperature was reduced from 1500k to 300K at the rate of 0.024k/fs, and the relaxation was carried out under the NVT ensemble.
The interaction potential between the virtual spherical indenter and the matrix atom is limited within the range of distance r, as shown in formula (1). Where R is the radius of the indenter, r is the distance from the model atom to the spherical centre of the indenter, and K can be regarded as the stiffness of the indenter. In this paper, the radius of the indenter is fixed at 15 Å, and the stiffness of the indenter is taken as 10 ev/Å3. The previous work [Citation36] confirmed that the value of K is reliable in calculating the repulsive force of the indenter. The indenter interacts with the matrix atom through potential. The indenter is initially located directly above the model and moves in the negative direction of the z-axis perpendicular to the target at a speed of 1 Å/ps, reaching the deepest depth of 15 Å after 15ps. The indenter was fixed for 50ps, and then the indenter was unloaded at a speed of 1 Å/ps. When the deformation law of FeCoCrCuNi hihigh-entropylloy with different Cu atomic ratio is studied, the whole indentation process is kept at constant temperature T = 300K; when the influence of temperature on deformation response of FeCoCrCuNi hihigh-entropylloy with equal atomic ratio was studied, the indentation process was kept at constant temperature T = 300, 650, 1000, 1350, 1700 and 2000K, respectively. The X- and Y-directions are set as periodic boundaries, and the Z direction is set as nonon-periodicoundaries.
The molecular dynamics software LAMMPS [Citation37] was used to simulate the above process and calculate the force change law of the atom in the Z direction. Ovito [Citation38] was used to visually analyse the atomic morphology, identify the dislocation type and measure the dislocation length.
Results and discussion
shows the dislocation movement and reaction law under nano indentation. When the indentation depth reached 5 Å, a few particles began to transform from FCC phase to HCP phase, and no dislocation occurred. When the indentation depth reaches 7.5 Å, more FCC phase particles turn into HCP phase, and dislocation slip is the main form in the initial stage of plastic deformation. Some total dislocations slip on the main slip plane and decompose to form Shockley partial dislocations. With the deepening of the indenter, the transformed HCP phase continues to increase, and more total dislocations decompose into Shockley partial dislocations. When the indentation depth reaches 15 Å, all the full perfect dislocations are decomposed into Shockley partial dislocations, and the extended dislocation cross slip phenomenon occurs. When the original slip plane and the
cross-slip plane intersect at an obtuse angle, the leading part of the extended dislocation first decomposes in the original slip plane, forming a small number of Hirth dislocations. In the maintan stage, the force generated by the indenter is continuously loaded on the material, so that the number of HCP phase particles reaches a peak. The cross-slip process continues and the number of Hirth dislocations increases. When two Shockley partial dislocations meet on the intersection line on the slip plane, dislocation reaction occurs to generate Stair-rod dislocation, which hinders the further slip of Shockley partial dislocations, reduces the proportion of Shockley partial dislocations, and improves the strength of the material. When the maintain time exceeds 15ps, the number of HCP phase particles begins to decrease. The percentage of Hirth dislocations is higher than that of the indent stage, and the dislocation movement basically stagnated during the subsequent maintain stage (as shown in )), indicating that the material properties will not be affected after the loading lasts for a period of time. With the retraction of the indenter, the number of HCP phase particles continued to decrease, and the extended dislocations re-entered the cross-slip stage. Some Shockley partial dislocations are decomposed into Hirth dislocations, and some Hirth dislocations and other Shockley partial dislocations recombine Shockley partial dislocations. The two types of dislocations are in local dynamic equilibrium. However, with the retraction of the indenter, the total dislocation length continues to decrease, and the plastic deformation part gradually recovers until the stress is completely unloaded. Finally, the indentation cannot completely return to the original state (as shown in )). In the whole indentation process of the high-entropy alloy, the dislocation ring cannot be found, indicating that the potential barrier of dislocation movement in the high-entropy alloy is higher than that of FCC crystal.
Figure 2. Transformation process of high entropy alloy at different indentation depths.

The load time curve of FeCoCrCuNi high-entropy alloy with equal atomic ratio at different temperatures is shown in . It can be seen that the load on FeCoCrCuNi high-entropy alloy gradually decreases with the increase of temperature during the intent stage. In the maintain stage, the load platform is presented, and the overall load decreases with the increase of temperature. In the retract stage, the load at different temperatures decreased significantly.
The curve of load displacement curve of FeCoCrCuNi high-entropy alloy at different temperatures is shown in . As the temperature rises, the load- depth curve shows increasingly drastic fluctuations, which is consistent with the phenomenon observed in the experiment [Citation39,Citation40]. It is speculated that as the temperature rises, the bonding force between atoms weakens, making the atoms deviate from the original position, intensifying the vibration, and making the curve show greater volatility as a whole. When the temperature exceeds 1350K, it can be found that the load of FeCoCrCuNi high-entropy alloy no longer keeps the elastic-plastic law, but floats up and down around a specific load. With the continuous increase of temperature, the load value decreases.
shows the total dislocation length time curve at different temperatures. The time history curves of total dislocation length at different temperatures show the same law: there are two peaks and one platform. With the increase of temperature, the dislocation length of different stages decreases sharply, and the dislocation length is the largest when the nanoindentation reaches the deepest point. In the maintain stage, the dislocation length decreases rapidly with time, then rises abruptly, and then drops to a certain platform again. At the stage of retraction, the dislocation length decreases greatly. With the increase of temperature, the dislocation plateau value in the maintain stage decreases significantly.
shows the change law of dislocation length in various stages of high-entropy alloy at different temperatures, and shows the change law of dislocation type in high-entropy alloy at different temperatures in indent, maintain and retract stages. With the increase of temperature, the total dislocation length of each stage decreases monotonically, and the dislocation length of the maintain stage is slightly lower than that of the indent stage. When the temperature is lower than 1000 K, the dislocation length decreases greatly when the indenter retracts. When the temperature is higher than 1000 K, the dislocation lengths of the three stages are similar.
Figure 6. Dislocation length variation in different stages of high entropy alloys at different temperatures.

Figure 8. Dislocation type changes of high entropy alloys in maintain stage at different temperatures.

In the indent stage, the main dislocation type is the Shockley partial dislocation. The Shockley partial dislocation length decreases with the increase of temperature. It is worth noting that different dislocation types are generated by dislocation reaction at different temperatures, which will affect the properties of materials.
In the maintain stage, the main dislocation type is still Shockley partial dislocation. With the increase of temperature, the length of Shockley partial dislocation decreases greatly. When the temperature is 300 K, some Hirth dislocations are generated in the holding stage. The higher the temperature is, the fewer new dislocation types are generated by dislocation reaction.
At the stage of retract, the length of each type of dislocation decreases rapidly. There are a few Hirth dislocations at low temperature. When the temperature reaches 1350 K, the dislocation length increases abnormally and some Stair-rod dislocations are formed. Compared with the normal temperature state, the dislocation length and the dislocation reaction type of the high-entropy alloy decrease greatly at high temperature. The reason for the above phenomenon will be explored by observing the dislocation movement law and defect morphology at high temperature.
The dislocation movement law at the indent stage at 650k is shown in , and the dislocation movement law at the maintain stage is shown in . In the indent stage, with the depth of the indenter, the strain of the atomic model increases and the dislocation length increases. At low strain, there are a large number of perfect dislocations. However, perfect dislocation slip needs to overcome the continuous energy barrier brought by the high point array friction. The slow moving perfect dislocation slip band will hinder the partial dislocations, and the partial dislocations need additional force to overcome the obstacles. Therefore, the number of partial dislocations induced slip increases gradually during the pressing process of the indenter. The end of No. 1 perfect dislocation is decomposed into two Shockley partial dislocations, as shown in the black circle in . At the same time, No. 1 dislocation enters the conjugate slip plane through cross slip and is decomposed into Frank dislocation and Shockley partial dislocation, as shown in the red circle in . The Frank dislocation is an immovable dislocation, which is fixed on the boundary line between the main slip plane and the conjugate slip plane. The Shockley partial dislocation slides downward to the right on the conjugate slip plane, and a stacking fault is generated between two partial dislocations under the action of stress, as shown by the arrow in . It shows that the deformation of FeCoCrCuNi high-entropy alloy is coordinated by dislocation slip when the deformation is low.
With the increase of deformation, the stacking fault length increases, and along the same direction, which should be caused by only a small number of slip systems participating in the deformation process at the initial stage of plastic deformation [Citation41]. Along with the deformation process, similar cross slip and decomposition behaviours may occur in the subsequent stacking faults. Three Frank dislocations will form a dislocation with a Berber vector of < 111> and leave no defects. Based on the above mechanism, perfect dislocations can effectively form stacking faults through cross slip and decomposition.
By observing the dislocation movement law of the high-entropy alloy at the temperature of 650k in the maintain stage, it can be seen that the stacking faults formed in the indent stage meet at the conjugate plane to form the Stair-rod dislocation, as shown by the arrow in , which hinders the movement of the surrounding dislocations. Therefore, the dislocations under the Stair-rod dislocation disappear at 15.8ps, as shown in . Partial dislocations No. 2 and No. 3 in and stacking fault No. 4 sandwiched in the middle form extended dislocations. Due to the presence of the front Stair-rod dislocations, the dislocations are prevented from sliding to the left, and the stacking fault No. 4 in is temporarily extended. However, due to the stress concentration at the head of the dislocation train during the maintain stage, the extended dislocations recombine with the dislocations on the lateral slip plane through cross slip ()), and rapidly decompose into stacking faults 6 ()) while sliding downward. Until 17.9ps, the extended dislocations recombine the perfect dislocations 7 ()), and the stacking faults basically disappear. Since then, no extended dislocations have been captured, only the repeated synthesis ()) and decomposition ()) of all dislocations. In the deformation area, there are active stacking faults repeatedly occurring and disappearing, which is consistent with the active stacking fault movement observed at the crack tip of the high-entropy alloy studied by Zhang [Citation42].
The evolution of the defect grid when the indenter reaches the deepest point at different temperatures is shown in . The atomic type is shown in . The white block in the figure is the defect grid. The defect grid is a two-dimensional fluid interface grid, which separates the good crystal region and the bad crystal region of the material. The outside is the good crystal region such as dislocation, stacking fault and grain boundary, and the surrounding area is the bad crystal region with non dislocation defects. It can be seen that with the increase of temperature, the type, number and length of dislocations near the deformation region are significantly decreased, the FCC phase atoms are greatly reduced, and the proportion of amorphous phase is gradually increased. When the temperature is lower than 1000K, the defect morphology of the model is mainly dislocation, which is concentrated near the indenter. When the temperature rises to 1350K, the defect grid becomes extremely irregular, and the bad defect morphology spreads to the whole region. At this time, the dislocations basically disappear, which means that the atoms leave the original orbit and form many holes and other point defects at high temperature. When the temperature rises above 1700K, a large number of FCC phase atoms turn to amorphous state, and the defect grid begins to collapse. The model is filled with poor defect motion, making it completely impossible to characterise crystal motion with dislocation defects, indicating that FeCoCrCuNi high-entropy alloys no longer follow the laws of elastic-plastic mechanics at high temperatures.
To sum up the evolution law of dislocation at different temperatures, it can be found that the dislocation reaction is dominated by the decomposition of perfect dislocation into Shockley partial dislocation. When the temperature is low (T < 1000K), the plastic deformation mechanism of FeCoCrCuNi high-entropy alloy is dislocation slip. As the temperature gradually increases from room temperature, the number of total dislocations decomposed into Shockley partial dislocations decreases, and the number of Frank dislocations and Stair-rod dislocations generated by conjugate slip and cross slip of dislocations increases, which hinders the further movement of dislocations and enables the extended dislocations to recombine perfect dislocations. This also explains the phenomenon that the length of dislocations at the maintain stage is basically unchanged at room temperature, while the length of dislocations at the maintain stage decreases sharply when the temperature rises to 650k. As the temperature continues to rise, more atomic phases are transformed into amorphous state, and dislocation defects are replaced by defects such as voids, which show that the elastic-plastic mechanical properties are lost macroscopically.
Conclusion
In this paper, the mechanical properties and dislocation movement of FeCoCrCuNi high-entropy alloy during nanoindentation were studied by molecular dynamics simulation. The mechanism of the influence of temperature on dislocation evolution of FeCoCrCuNi high-entropy alloy was obtained. The following specific conclusions can be drawn:
Some FCC phase particles of FeCoCrCuNi high-entropy alloy are transformed into HCP phase during indentation at room temperature. At the initial stage of plastic deformation, dislocation movement is dominated by slip, and all dislocations slip and decompose on the main slip plane, forming Shockley partial dislocations. In the maintain stage, the extended dislocation cross slip phenomenon occurs, and the Hirth dislocation and the Stair-rod dislocation are formed successively, which makes the dislocation reaction stagnate in the later stage of the maintain stage. After entering the retract stage, the HCP phase particles continued to decrease, and the local dynamic equilibrium between the Hirth dislocation and the Shockley partial dislocation, but the total dislocation length continues to decrease.
With the increase of temperature, the load of FeCoCrCuNi high-entropy alloy decrease gradually. When the temperature exceeds 1350K, the elastic-plastic law of the material is no longer maintained.
With the increase of temperature, the total length of dislocation in each stage decreases monotonically, and the type of dislocation reaction decreases.
The mechanism of temperature affecting the properties of FeCoCrCuNi high-entropy alloy is as follows: With the temperature increasing from room temperature, the number of perfect dislocations decomposed into Shockley partial dislocations decreases, and Frank dislocations and Stair-rod dislocations generated by conjugated and cross slip increase, which hinders the further movement of dislocations. The extended dislocations recombine with perfect dislocations, and the length of dislocations decreases sharply. As the temperature continues to rise, more atomic phases are transformed into amorphous state, and dislocation defects are replaced by voids, which makes the high-entropy alloy lose elastic-plastic mechanical properties.
Author contribution statement
Ye Du – Primary author and performed most of the simulation and analysis in this manuscript.
Qiang Li – Collected data and provided proofreading the manuscript.
Acknowledgments
We acknowledge the guidance given by Key Laboratory of Science and Technology on Materials under Shock and Impact.
Disclosure statement
No potential conflict of interest was reported by the author(s).
Data availability statement
The raw/processed data required to reproduce these findings cannot be shared at this time due to legal and ethical reasons.
Additional information
Funding
References
- Chen HH, Zhang XF, Liu C, et al. Research progress on impact deformation behavior of high-entropy alloys. Explosion And Shock Waves. 2021;41:041402. doi: 10.11883/bzycj-2020-0414
- George EP, Curtin WA, Tasan CC. High entropy alloys: a focused review of mechanical properties and deformation mechanisms. Acta Materialia. 2020;188:435–12. doi: 10.1016/j.actamat.2019.12.015
- Li JG, Huang RR, Zhang Q, et al. Mechnical proerties and behaviors of high entropy alloys. Chinese J Theor Appl Mech. 2020;52:333–359. doi: 10.6052/0459-1879-20-009
- Li J, Feng H, Chen Y, et al. Progress in theoretical modeling and simulation on strengthening and toughening of high-entropy alloys. Chinese J Solid Mech. 2020;41:93–108. doi: 10.19636/j.cnki.cjsm42-1250/o3.2020.009
- Lu ZP, Lei ZF, Huang HL, et al. Deformation behavior and toughening of high-entropy alloys. Acta Metall Sin. 2018;54:1553–1566. doi: 10.11900/0412.1961.2018.00372
- Senkov ON, Wilks GB, Scott JM, et al. Mechanical properties of Nb25Mo25Ta25W25 and V20Nb20Mo20Ta20W20 refractory high entropy alloys. Intermetallics. 2011;19:698–706. doi: 10.1016/j.intermet.2011.01.004
- Chen H, Kauffmann A, Laube S, et al. Contribution of lattice distortion to solid solution strengthening in a series of refractory high entropy alloys. Metall Mater Trans A. 2018;49(3):772–781. doi: 10.1007/s11661017-4386-1
- Xie XC, Li N, Liu W, et al. Research progress of refractory high-entropy alloys: a review. Chin J Mech Eng. 2022;35(1). doi: 10.1186/s10033-022-00814-0
- Zhang ZR, Zhang H, Tang Y, et al. Microstructure, mechanical properties and energetic characteristics of a novel highentropy alloy HfZrTiTa0.53. Mater Design. 2017;133:435–443. doi: 10.1016/j.matdes.2017.08.022
- Zhang ZR. Microstructure and mechanical properties of Hf Zr Ti tax high-entropy alloys energetic structural materials. Changsha, Hunan, P.R.China: National University of Defense Technology; 2017.
- Chen HH, Zhang XF, Xiong W, et al. Dynamic mechanical behavior and pentration performance of WFeNiMo high-entropy alloy. Chinese J Theor Appl Mech. 2020;52:1443–1453. doi: 10.6052/0459-1879-20-166
- Liu Y, Chen M, Li YX, et al. Microstructure and mechanical performance of AlxCoCrCuFeNi high-entropy alloys. Rare Metal Mat Eng. 2009;38:1602.
- Li BY, Peng K, Hu AP, et al. Structure and properties of FeCoNiCrCu0. 5Alx high- entropy alloy. Trans Nonferrous Met Soc China. 2013;23:735. doi: 10.1016/S1003-6326(13)62523-6
- Dong B, Wang XM, Zhu ZL. Study on the mechanical performance and microstructure of FeCoCrCuNi high-entropy alloy with crack and void by molecular dynamics simulations. J Atomic Mol Phys. 2020;37:591–595.
- Gong N, Meng TL, Cao J, et al. Laser-cladding of high entropy alloy coatings: an overview. Mater Technol. 2023;38(1). doi: 10.1080/10667857.2022.2151696
- Feng L, Yang WJ, Ma K, et al. Microstructure and properties of cold spraying AlCoCrCuFeNix HEA coatings synthesized by induction remelting. Mater Technol. 2022;37:2567–2579. doi: 10.1080/10667857.2022.2046929
- Maldonado AJ, Misra KP, Misra RDK. Grain boundary segregation in a high entropy alloy. Mater Technol. 2023;38. doi: 10.1080/10667857.2023.2221959
- Tan SY, Liu XD, Wang ZZ. Nanoindentation mechanical properties of CoCrFeNi high entropy alloy films. Mater Technol. 2022;37:1097–1108. doi: 10.1080/10667857.2021.1921329
- Xu L, Sun DQ, Ma JM, et al. Applications of depth‑sensing indentation on asphalt materials: a review. Constr Build Mater. 2020;268:121195. doi: 10.1016/j.conbuildmat.2020.121195
- Fernandes JV, Antunes JM, Sakharova NA, et al. Young’s modulus of thin films using depth-sensing indentation. Philos Mag Lett. 2010;90(1):9–22. doi: 10.1080/09500830903334312
- Simões MI, Antunes JM, Fernandes JV, et al. Numerical simulation of the depth-sensing Indentation test with Knoop Indenter. Metals - Open Access Metall J. 2018;8(11):885. doi: 10.3390/met8110885
- Li Q, Jiang CL, Du Y. Molecular dynamics study on dynamic mechanical behaviour of FeCoCrCuNi high entropy alloy. Mater Technol. 2023;38. doi: 10.1080/10667857.2023.2200660
- Ruestes CJ, Alhafez IA, Urbassek HM. Atomistic studies of nanoindentation—a review of recent advances. Crystals. 2017;7(10):293. doi: 10.3390/cryst7100293
- Alhafez IA, Ruestes CJ, Bringa EM, et al. Indentation and scratching of iron by a rotating tool – a molecular dynamics study. Comput Mater Sci. 194. doi: 10.1016/j.commatsci.2021.110445
- Li J, Fang QH, Liu B, et al. The effects of pore and second-phase particle on the mechanical properties of machining copper matrix from molecular dynamic simulation. Appl Surface Sci. 2016;384:419–431. doi: 10.1016/j.apsusc.2016.05.051
- Wang ZN, Li J, Fang QH, et al. Investigation into nanoscratching mechanical response of AlCrCuFeNi high-entropy alloys using atomic simulations. Appl Surface Sci. 2017;416:470–481. doi: 10.1016/j.apsusc.2017.04.009
- Huang JC, Sheer TJ, Bailey-McEwan M. Heat transfer and pressure drop in plate heat exchanger refrigerant evaporators. Int J Refrig. 2012;35(2):325–335. doi: 10.1016/j.ijrefrig.2011.11.002
- Alhafez IA, Ruestes CJ, Bringa EM, et al. Nanoindentation into a high-entropy alloy-an atomistic study. J Alloys Compd. 2019;803:618–624. doi: 10.1016/j.jallcom.2019.06.277
- Mu AR, Han Y, Song XJ, et al. Nanoindentation into feconicrcu high-entropy alloy: an atomistic study. Mater Sci Technol. 2021;37:202–209. doi: 10.1080/02670836.2021.1885095
- Tian YY, Fang QH, Li J. Molecular dynamics simulations for nanoindentation response of nanotwinned FeNiCrCoCu high entropy alloy. Nanotechnology. 2020;31:465701. doi: 10.1088/1361-6528/ababcd
- Feng H, Tang JW, Chen HT, et al. Indentation-induced plastic behaviour of nanotwinned Cu/high entropy alloy FeCoCrNi nanolaminate: an atomic simulation. RSC Adv. 2020;10(16):9187–9192. doi: 10.1039/D0RA00518E
- Zhang HT, Siu KW, Liao WB, et al. In situ mechanical characterization of CoCrCuFeNi high-entropy alloy micro/nano-pillars for their size-dependent mechanical behavior. Mater Res Express. 2016;3(9):094002. doi: 10.1088/2053-1591/3/9/094002
- Farkas D, Caro A. Model interatomic potentials and lattice strain in a high- entropy alloy. J Mater Res. 2018;33(19):3218–3225. doi: 10.1557/jmr.2018.245
- Li J, Guo XX, Ma SG, et al. Mechanical properties of AlCrFeCuNi high entropy alloy: a molecular dynamics study. Chinese J High Press Phys. 2020;34:37–45. doi: 10.11858/gywlxb.20190762
- Pi JH, Pan Y, Zhang H, et al. Microstructure and properties of microstructure and properties of AlCrFeCuNix (0.6≤x≤1.4) high-entropy alloys. Mater Sci Eng A. 2012;534:228–233. doi: 10.1016/j.msea.2011.11.063
- Fang QH, Yi M, Li J, et al. Deformation behaviors of Cu29Zr32Ti15Al5Ni19 high entropy bulk metallic glass during nanoindentation. Appl Surface Sci. 2018;443:122–130. doi: 10.1016/j.apsusc.2018.02.245
- Plimpton S. Fast parallel algorithms for short-range molecular dynamics. J Comput Phys. 1995;117(1):1–19. doi: 10.1006/jcph.1995.1039
- Stukowski A. Visualization and analysis of atomistic simulation data with OVITO–the open visualization tool. Modelling Simul Mater Sci Eng. 2010;18(1):15012. doi: 10.1088/0965-0393/18/1/015012
- Lin CM, Tsai HL. Equilibrium phase of high-entropy FeCoNiCrCu0.5 alloy at elevated temperature. J Alloys Compd. 2010;489:30–35. doi: 10.1016/j.jallcom.2009.09.041
- Samaei AT, Mirsayar MM, Aliha MRM. The microstructure and mechanical behavior of modern high temperature alloys. Eng Solid Mech. 2015;3(1):1–20. doi: 10.5267/j.esm.2015.1.001
- Chen CX. Deformation mechanism of high-entropy alloys. Hangzhou: Zhejiang University; 6 2018.
- Zhang ZJ, Mao MM, Wang JW, et al. Nanoscale origins of the damage tolerance of the high-entropy alloy CrMnFeCoNi. Nat Commun. 2015;6(1):10143. doi: 10.1038/ncomms10143