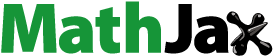
ABSTRACT
In this study, hydrothermal-calcination method was employed to synthesize pure vanadium oxide and zinc doped vanadium oxide powders. The crystal structure, morphology, chemical state and light absorption properties of the powders were also thoroughly analyzed. The antimicrobial effect against Candida albicans was evaluated by the circle of inhibition method and plate colony counting method. This study reveals the antibacterial mechanism of oxygen defects generating reactive oxygen species, leading to oxidative stress damage. This study not only demonstrates the potential of vanadium oxides as an efficient antimicrobial agent, but also provides a strong experimental basis and theoretical support for the design and optimization of novel antimicrobial materials by modulating the defect engineering of the materials. This finding provides a new perspective for the development of antimicrobial strategies against microorganisms and has important application prospects for the clinical treatment of Candida albicans infections.
Introduction
Candida albicans is a widespread human pathogen, and its infections result in diseases ranging from skin and mucosal infections to systemic infections, where the morbidity and mortality of systemic infections are quite high [Citation1–3]. Therefore, the development of effective antimicrobial strategies to combat Candida albicans infections is of great clinical importance [Citation4–6].
Vanadium oxides, as an important transition metal oxide, have shown great potential for applications in photocatalysis, electrochemistry and antimicrobials due to their unique physical and chemical properties [Citation7,Citation8]. In recent years, vanadium oxides have attracted much attention for research as antimicrobial materials [Citation9–11]. The key to the antimicrobial ability of vanadium oxides is their ability to generate large amounts of Reactive Oxygen Species (ROS) through the formation of oxygen defects [Citation12–16]. ROS are capable of triggering strong oxidative stress, affecting the integrity of microbial cell walls and cell membranes, and ultimately leading to the leakage of cellular contents and effective inhibition of bacteria [Citation17,Citation18]. The effectiveness of this antimicrobial mechanism demonstrates the advantages of vanadium oxides as an antimicrobial material. However, the antimicrobial effect of pure vanadium oxides has yet to be improved. In order to optimize their performance, attempts have been made to change their electronic structure by doping with other metallic elements, which in turn improves their antimicrobial activity [Citation19–22]. It has been found that elemental doping gives inorganic materials a higher concentration of oxygen defects, which can effectively enhance their antimicrobial properties [Citation23–25]. The element zinc has good biocompatibility and antimicrobial properties, so we chose zinc as the doping element, which is expected to enhance the antimicrobial effect of vanadium oxides [Citation26–28]. It has been shown that doping can improve the reactivity of a material by increasing its defect concentration. This is not only applicable to improve the antimicrobial effect, but may also have a positive impact on improving the performance of the materials in other applications such as photocatalysis and electrochemical energy storage.
In this study, pure vanadium oxide and zinc-doped vanadium oxide powders were prepared by hydrothermal-calcination method using ammonium metavanadate, anhydrous oxalic acid and zinc sulphate heptahydrate for in-depth material characterization. We will evaluate in detail the antimicrobial effect of these materials against Candida albicans and explore the possible antimicrobial mechanism, where the oxygen-deficient defects can trap oxygen molecules and enhance the activity of the powders, which in turn promotes the production of intracellular ROS and disrupts the morphology and membrane integrity of Candida albicans to achieve antimicrobial activity. We expect that this study will provide new perspectives and strategies for the antimicrobial treatment of Candida albicans, as well as useful reference information for researchers in related fields.
Experiment
Materials and synthesis
Ammonium metavanadate (NH4VO3), anhydrous oxalic acid (C2H2O4) and zinc sulphate heptahydrate (ZnSO4‧7 H2O) were purchased from Aladdin Co. Ltd. (Shanghai, China), and all the chemicals were analytically pure and unpurified. First, NH4VO3 and C2H2O4 were added to 80 mL of deionized water at a molar ratio of 2:3 and stirred for h at room temperature to obtain a blue liquid. The solution was then transferred to a00 mL reactor and kept in an oven at80°C for 6 h, respectively. After cooling to room temperature, it was washed several times with deionized water and ethanol. After vacuum drying at 65°C for2 h, it was calcined in high purity argon gas at 650°C for.5 h to obtain the final powder labelled as S1. Then, NH4VO3, C2H2O4 and ZnSO4‧7 H2O were added into 80 mL of deionized water in a molar ratio of 2:3:0.006, and stirred for hour at room temperature to obtain a blue liquid. The subsequent steps were the same as the previous process, and the resulting final sample was recorded as S2.
Materials characterization
The crystal structures of the synthesized samples were analysed by a Bruker D8 Advance powder X-ray diffractometer (XRD, Bruker AXS D8-Advance, Germany) and a Cμ Kα source. Surface morphology was observed by field emission scanning electron microscopy (FE-SEM, HITACHI Sμ8010, Japan) and (FE-SEM, ZEISS Gemini 300, Germany). The structure and valence of the samples were analysed by X-ray photoelectron spectroscopy (XPS, Thermo-Fischer, ESCALAB Xi+, U.S.A.). The oxygen defects of the samples were characterized using paramagnetic resonance spectroscopy (EPR, Bruker EMXPLUS, Germany). The light absorption properties of the powders in the wavelength range of 200–800 nm were investigated using a UV-visible spectrophotometer (Shimadzu UV-3600, Japan).
Antibacterial experiments
Strains, media and conditions
In this study, Candida albicans ATCC10231 was used to evaluate the potential antimicrobial effect of S1 and S2 powders.S1, S2 powders, and silica powders, Sabouraud Dextrose Medium (SDM), and deionized water were autoclaved for 20 min at21°C. SDM medium consisted of 40 g of dextrose,0 g of peptone, and deionized water, fixed to L. The solid medium was supplemented with an additional 20 g of agar. Candida albicans ATCC10231 seeds were added to 24-well plates containing 5 mL of SDM medium at a final concentration of approximately × 105 cells/mL, and S1 and S2 powders were added to the desired final concentration, and the control C1 group was without any powder. The 24-well plates were fixed on a shaker and incubated at a constant temperature of 30°C with shaking at 200 r/min. Samples were taken at certain times for relevant assays.
Antibacterial circle test
The inhibitory activity of S1 and S2 was determined by measuring the size of the diameter of the inhibition circle. Several small wells were punched in plates coated with SDM of Candida albicans ATCC10231, and 30 μl of a mixture of the powder at a final concentration of00 mg/ml and deionized water was added to the wells. The plates were incubated at 30°C for 24 h and the diameter of the zone of inhibition was measured.
Antibacterial rate test
S1 powder, S2 powder, and control C1 were each co-cultured in 24-well plates by adding SDM medium containing × 105 cells/ml of bacteria at a final concentration of 2.5 mg/ml of powder. The culture conditions were as described previously. At 48 h, 50 μl of the sample solution was taken, diluted to the appropriate concentration, and then00 μl was taken and evenly applied to the surface of the plates covered with SDM. The plates were incubated at 30°C for 24 hours. The number of bacteria in the diluted solution was calculated by counting the number of colonies and then converted to the number of bacteria in the original solution. The bacterial inhibition rate was defined:
where R is the percentage reduction of Candida albicans ATCC10231, A is the average number of viable bacteria in the control group, and B is the average number of viable bacteria in the sample. Each set of experiments was repeated three times.
Results and discussion
Characterisation of materials
The XRD spectra of S1 and S2 are shown in . From the analysis, it is known that S1 and S2 are a mixture of V6O13 (JCPDS card number 43–1050, space group: C2/m) phase and VO2 (JCPDS card number 43–1051, space group: P21/c) phase. According to previous literature [Citation29], high-temperature calcination leads to a phase transition of vanadium oxide from V6O13 to VO2. The phase transition mainly occurs at the same time of crystal nucleation and growth, which is confirmed by the narrower peaks of both the V6O13 and VO2 phases in the XRD results. In addition, the synergistic motion of VO6 octahedra in V6O13 plays an important role, and during the formation of VO2, some oxygen is released and transferred to the surface by diffusion, with a consequent decrease in the free energy of the system, and this phase transition contributes to the formation of vanadium oxide oxygen defects [Citation30]. From S2, it can be seen that Zn doping did not cause the production of other oxides and did not change the physical phase.
show the FESEM images of S1 and S2, respectively. It can be seen that the morphology of S1 is a mixture of rod-like and granular morphology. Compared with S1, S2 has the same morphology and smaller size. In fact, the Zn2+ ions acted as passivators to inhibit the growth of the crystals, leading to the formation of smaller sizes. The experimental composition of the samples is consistent with the given using energy dispersive x-ray spectroscopy (EDS) localized quantitative analysis as shown in . The elemental mapping of the samples shows that oxygen, vanadium and zinc atoms are uniformly distributed on the sample surface (). The results also show that Zn2+ is doped into the crystal structure of the material.
Figure 2. SEM images of the synthesized samples: (a) S1; (b) S2; (c-e) mapping diagram of S2; (f) EDS diagram of S2.

The elemental types and states present on the material surface were characterized by XPS, as shown in . The high-resolution V2p spectra of samples S1 () and S2 () indicate the presence of V ions in two different oxidation states, a result that agrees well with the chemical state of vanadium as reported in the literature. To confirm rich oxygen defects exist in S1 and S2, high resolution O1s spectrum was also studied. For S1, two independent peaks emerge [Citation31]. The first peak at 529.8 eV matches with lattice O (Olatt). The second one located at 530.7 eV can be attributed to oxygen defect (Od) (). For S2, two independent peaks, 530.0 eV and 531.0 eV, corresponding to lattice O (Olatt) and oxygen defect (Od), were also observed (). The high oxygen defect content between them originates from calcination, and Zn doping simultaneously increases the oxygen defects of S2. The high-resolution spectra of V2p spectra () and O1s spectra () of S1 and S2 indicate the presence of V4+ and V5+ and oxygen defects in all samples. From the XPS spectra for Zn2p (), Zn peaks were identified with the two main characteristic peaks corresponding to 2p1/2 and 2p3/2 of Zn2+ with BE values at033.7 eV and019.3 eV, respectively [Citation29,Citation32,Citation33]. The room-temperature Electron Paramagnetic Resonance (EPR) spectra of S1 and S2 samples are shown in . The signals of S2 are stronger than that of S1, which suggests that more oxygen defects have been introduced. This is consistent with the XPS findings.
Figure 3. UV-Vis absorption spectra and EPR spectra of S1 and S2 samples: (a-b) high-resolution V2p spectra and O1s spectra of S1; (c-d) high-resolution V2p spectra and O1s spectra of S2; (e) high-resolution Z2p spectra of S2; (f) EPR spectra of S1 and S2 samples.

The UV-visible absorption spectra of S1 and S2 are shown in . For direct bandgap semiconductors, according to the Kubelka-Munk formula
where R is the reflectance, E=hν, h is Planck’s constant. The plot of (F(R)hν)2 versus hν for the samples is shown in . The optical band gap of the sample is determined through extrapolation of the linearly fitted data along the energy axis. As shown in , the band gaps of S1 and S2 are approximately 2.17 eV and.95 eV, respectively. These variations in the band gaps are attributed to the presence of oxygen defects, as well as other defects on the particle surface.
Antibacterial properties
In this study, we evaluated the antimicrobial activity of the samples using the circle of inhibition assay and the reduction in the number of Candida albicans colonies on agar plates.
Firstly, the antimicrobial activity against Candida albicans was examined for samples S1 and S2.C1 was prepared without any powder and served as a control. The diameter of the circle of inhibition for S1 and S2 was 0.69 ± 0.01 cm and.07 ± 0.02 cm, respectively, which shows that the diameter of the circle of inhibition of the sample S2 was larger than that of the sample S1, which indicates that S2 is more capable of inhibiting the bacterial growth of Candida albicans than S1 ().
Figure 5. Antimicrobial effect of S1 and S2 samples against Candida albicans after 24 h. (a): inhibition circles of S1 and S2, C1 indicates the control group representing the absence of added powder; (b) image of Candida albicans colonies on the agar plate, as a control group, without the addition of any samples; (c) image of Candida albicans colonies on the agar plate with the addition of the S1 samples; (d) agar plate with Candida albicans colonies on the agar plate image, S2 sample added.

In order to further test the antimicrobial capacity of the powders, we tested the bactericidal rate of powders S1 and S2. Succinctly, Candida albicans ATCC10231 and the powder were co-cultured for 24 h. The fermentation broth diluted to a certain concentration was applied to the solid medium and incubated for 24 h. Colonies were counted and the bactericidal rate was calculated; the bacteriophage method is in the Experimental Methods section. The sterilization rate of powder S1 and S2 were 92.9 ± 0.34% and 99.4 ± 0.27%, respectively (). The results showed that both S1 and S2 samples caused strong oxidative stress damage to Candida albicans, inducing the production of more reactive oxygen species, and both of them possessed excellent antibacterial effects.
Probable antibacterial mechanism
Combining the results of the above material analysis and antimicrobial activity evaluation experiments, it can be concluded that the antimicrobial activity of zinc-doped vanadium oxides is superior to that of pure vanadium oxides. The possible antimicrobial mechanisms are as follows.
The addition of zinc-doped vanadium oxides to a solution containing Candida albicans releases Zn2+ with low toxicity and biological antimicrobial activity. These metal ions can come into direct contact with the bacteria and bind to the component proteins in the cell wall and cell membrane, denaturing and inactivating the component proteins. This causes the outer layer to lose its protection and the bacteria to rupture and die. In addition, the interaction between Zn2+ and the DNA structure prevents the bacteria from reproducing.
Zinc-doped vanadium oxides are rich in oxygen defects, which can have an important effect on the electronic structure of the material as well as properties such as surface adsorption. It has been found that oxygen defects can effectively trap dissolved oxygen and generate ROS, and when microorganisms are exposed to oxidative stress caused by oxygen defects, they protect themselves by producing antioxidant enzymes such as peroxidase, catalase and superoxide dismutase. These enzymes break down ROS and render them harmless. However, if these enzymes are unable to completely break down these ROS, they can damage the cell membranes and DNA of microorganisms, which can lead to their death. Single linear oxygen (1O2), superoxide anion radicals (‧O2−), peroxides (H2O2), and hydroxyl radicals (‧OH) are considered to be common ROS. Up to 25–30% of the oxygen vacancy-associated defects have been reported to be thermodynamically stable. By referring to other literature and experimental results, we propose that the oxygen defects on the surface of zinc-doped vanadium oxides are prone to promote the generation of ROS such as superoxide by the following mechanism:
In addition, zinc-doped vanadium oxides can work in dark conditions where Candida albicans tends to multiply, so they are not limited by light sources, and the application areas of antimicrobial agents will be further expanded. In summary, the high antimicrobial activity of zinc-doped vanadium oxides stems from the synergistic effect of Zn2+ ion release and oxygen-deficiency-mediated oxidative stress damage.
Conclusion
In summary, we prepared oxygen-deficient pure vanadium oxides and zinc-doped vanadium oxide powders enriched with oxygen defects by hydrothermal-calcination method, and all the samples showed good in vitro antimicrobial effects against Candida albicans. Pure vanadium oxides under specific external environments caused a crystallographic transition leading to a decrease in the valence state of vanadium ions (gradual transition from V5+ to V4+), and the detachment of oxygen from the lattice in the process led to the formation of oxygen defects due to oxygen deficiency. Zinc doping further enhances the oxygen defect defects in vanadium oxides and reduces their energy band width, which is more favourable for antimicrobial properties. One potential mechanism is that the oxygen defect defects in the material induce ROS production from dissolved oxygen and water in the environment, causing oxidative stress, disrupting the morphology and membrane integrity of Candida albicans, and enhancing the membrane permeability to cause leakage of intracellular material. Therefore, our study reveals the potential mechanism of zinc-doped vanadium oxides to enhance antimicrobial activity and provides a new research direction to further optimize the design and development of antimicrobial materials. The findings of this study are expected to promote the development of novel antimicrobial materials and provide theoretical support for antimicrobial treatment in practical applications.
Disclosure statement
No potential conflict of interest was reported by the author(s).
References
- Ye W, Chen Y, Zhang W, et al. Potential anti-Candida albicans mechanism of trichoderma acid from trichoderma spirale. Int J Mol Sci. 2023;24(6):5445. doi: 10.3390/ijms24065445
- Masoumizadeh M, Madani M, Fatahian S, et al. Effect of Silver Nanoparticles (AgNPs) on Candida albicans, Candida dubliniensis and Candida guilliermondii. Curr Drug Ther. 2022;17(1):50–8. doi: 10.2174/1574885517666220221093456
- Datta A, Das D, Nett JE, et al. Differential skin immune responses in mice intradermally infected with Candida auris and Candida albicans microbiology spectrum. Microbiol Spectr. 2023;11(6):e02215–23. doi: 10.1128/spectrum.02215-23
- Ma D-Y, Wang Z-J, Chen Y-C, et al. Antifungal compounds of Chinese prickly ash against drug-resistant Candida albicans. Food Chemistry: X. 2022;15:100400. doi: 10.1016/j.fochx.2022.100400
- Wang W, Zhao J, Zhang Z. Bacillus metabolites: compounds, identification and anti-Candida albicans mechanisms. Microbiol Res. 2022;13(4):972–984. doi: 10.3390/microbiolres13040070
- Khan A, Moni SS, Ali M, et al. Antifungal activity of plant secondary metabolites on Candida albicans: an updated review. Curr Mol Pharmacol. 2023;16(1):15–42. doi: 10.2174/1874467215666220304143332
- Hu P, Hu P, Vu TD, et al. Vanadium oxide: phase diagrams, structures, synthesis, and applications. Chem Rev. 2023;123(8):4353–4415. doi: 10.1021/acs.chemrev.2c00546
- Li P, Feng Y, Cheng D, et al. Self-template synthesis of mesoporous vanadium oxide nanospheres with intrinsic peroxidase-like activity and high antibacterial performance. J Colloid Interface Sci. 2022;625:435–445. doi: 10.1016/j.jcis.2022.06.049
- Huang L, Niu Y, Xu G, et al. Generation of vanadium oxide quantum dots with distinct fluorescence and antibacterial activity via a room-temperature agitation strategy. ChemNanomat. 2018;4(10):1048–1053. doi: 10.1002/cnma.201800257
- Menazea AA, El-Newehy MH, Thamer BM, et al. Preparation of antibacterial film-based biopolymer embedded with vanadium oxide nanoparticles using one-pot laser ablation. J Mol Struct. 2021;1225:129163. doi: 10.1016/j.molstruc.2020.129163
- Sun H, Yang Z, Pu Y, et al. Zinc oxide/vanadium pentoxide heterostructures with enhanced day-night antibacterial activities. J Colloid Interface Sci. 2019;547:40–49. doi: 10.1016/j.jcis.2019.03.061
- Liu L, Li S, Shi D, et al. Antibacterial activity of oxygen vacancy-mediated ROS production of V6O13 powder against Candida albicans. Inorganics. 2023;11(11):429. doi: 10.3390/inorganics11110429
- Zheng F, Du W, Yang M, et al. Constructing ROS-Responsive supramolecular gel with innate antibacterial properties. Pharmaceutics. 2023;15(8):2161. doi: 10.3390/pharmaceutics15082161
- Sá AS, de Lima IS, Honório LM, et al. ROS-mediated antibacterial response of ZnO and ZnO containing cerium under light. Chem Papers. 2022;76(11):7051–7060. doi: 10.1007/s11696-022-02390-y
- Chandrasekaran K, Kim S, Choi M-J, et al. Surface engineering of tin dioxide through chitosan: band-gap tuning of spherical structure with oxygen vacancies for enhanced antibacterial therapeutic effects. J Ind Eng Chem. 2024;130:255–265. doi: 10.1016/j.jiec.2023.09.029
- Hazarika R, Kalita B. Effect of oxygen vacancy defects on electronic and optical properties of MgO monolayers: first principles study. Mater Sci Eng B. 2022;286:115974. doi: 10.1016/j.mseb.2022.115974
- Song H, Zhang M, Tong W. Single-Atom Nanozymes: fabrication, characterization, surface modification and applications of ROS scavenging and antibacterial. Molecules. 2022;27(17):5426. doi: 10.3390/molecules27175426
- Paula CTB, Madeira AB, Pereira P, et al. ROS-degradable PEG-based wound dressing films with drug release and antibacterial properties. Eur Polym J. 2022;177:111447. doi: 10.1016/j.eurpolymj.2022.111447
- Simo A, Drah M, Sibuyi NRS, et al. Hydrothermal synthesis of cobalt-doped vanadium oxides: antimicrobial activity study. Ceram Int. 2018;44(7):7716–7722. doi: 10.1016/j.ceramint.2018.01.198
- Tousley ME, Wren AW, Towler MR, et al. Processing, characterization, and bactericidal activity of undoped and silver-doped vanadium oxides. Mater Chem Phys. 2012;137(2):596–603. doi: 10.1016/j.matchemphys.2012.10.008
- Tahir T, Chaudhary K, Warsi MF, et al. Synthesis of sponge like Gd3+ doped vanadium oxide/2D MXene composites for improved degradation of industrial effluents and pathogens. Ceram Int. 2022;48(2):1969–1980. doi: 10.1016/j.ceramint.2021.09.282
- Ragupathi C, Geetha VT, Narayanan S, et al. Study of Ce-doping effects on optical, morphological, magnetic, structural, and antibacterial properties of NiCr2O4 ceramics. Mater Sci Eng B. 2023;291:116358. doi: 10.1016/j.mseb.2023.116358
- Liu Y, Zhang Y, Jiang H, et al. Synergistic engineering of oxygen-defect and heterojunction boosts Zn2+ (De)intercalation kinetics in vanadium oxide for high-performance zinc-ion batteries. Chem Eng J. 2022;435:134949. doi: 10.1016/j.cej.2022.134949
- Singh J, Juneja S, Palsaniya S, et al. Evidence of oxygen defects mediated enhanced photocatalytic and antibacterial performance of ZnO nanorods. Colloids Surf B Biointerfaces. 2019;184:110541. doi: 10.1016/j.colsurfb.2019.110541
- Zhang J, Shi R, Zhang Z, et al. Modulating the large vacancy types of CuS ultrathin nanosheets via defect engineering to improve the photocatalytic antibacterial performance. Appl Surf Sci. 2023;639:158269. doi: 10.1016/j.apsusc.2023.158269
- Khalid A, Ahmad P, Alharthi AI, et al. Optical, and antibacterial efficacy of pure and zinc-doped copper oxide against pathogenic bacteria. Nanomaterials. 2021;11(2):451. doi: 10.3390/nano11020451
- Roesner M, Zankovic S, Kovacs A, et al. Biocompatibility assessment of zinc alloys as a new potential material for bioabsorbable implants for osteosynthesis. Materials. 2023;16(15):5224. doi: 10.3390/ma16155224
- Udhayakumar G, Muthukumarasamy N, Velauthapillai D, et al. Highly crystalline zinc incorporated hydroxyapatite nanorods’ synthesis, characterization, thermal, biocompatibility, and antibacterial study. Appl Phys A. 2017;123(10):655. doi: 10.1007/s00339-017-1248-z
- Meng J, Zhang S, Liu X, et al. Facile synthesis of 3D urchin-like V6O13 microflowers as cathode materials for high-capacity and high-rate lithium-ion batteries. J Electroanal Chem. 2021;900. doi: 10.1016/j.jelechem.2021.115742
- Horiuchi S, Saeki M, Matsui Y, et al. Transition of V6O13 to VO2 observed with a high-resolution electron-microscope. Acta Crystallogr A-Found Adv. 1975;31(5):660–664. doi: 10.1107/S0567739475001398
- Hou X, Jiang S, Wang X, et al. Anti-biofouling photothermal film for solar steam generation based on oxygen defects rich and haloperoxidase mimic active V6O13. J Solid State Chem. 2022;315. doi: 10.1016/j.jssc.2022.123443
- Goyal CP, Goyal D, Ganesh V, et al. Improvement of photocatalytic activity by zn doping in Cu2O. Phys Solid State. 2020;62(10):1796–1802. doi: 10.1134/S1063783420100091
- Nguyen XS, Pham TD, Tran TTT, et al. Photo-oxidation efficient as (III) under visible light using microporous Zn doped-Fe3O4 sphere. Mater Technol. 2020;35(6):335–348. doi: 10.1080/10667857.2019.1682857