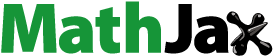
ABSTRACT
A multi-layer composite material consisting of 316 L steel and Al6061 aluminium was prepared via hot pressing. The mechanical properties of this heterogeneous layered composite material were studied under various reduction rates. The results revealed that the overall performance of the material initially improved and then declined as the reduction rates increased. Notably, at a hot pressing temperature of 400°C, the material exhibited optimal performance at a reduction rate of 32%. Furthermore, finite element simulations of the material processing process were performed using ABAQUS software, and the stress-strain state was analysed. The findings indicated that the overall performance of the material is influenced by both the presence of intermetallic compounds (IMCs) at the interface and the shear stress at the interface. In comparison to the aluminium layer, the performance of the steel layer undergoes more pronounced changes at the same reduction rate.
Introduction
With the growing societal demand for energy conservation and emission reduction, lightweight materials have emerged as a primary focus of materials science research. This is especially critical in the automotive industry, where there is an urgent need for lighter materials. Currently, the density of steel commonly used in the industry is about 7.85 g/cm3, which is difficult to meet the requirements of weight reduction. As a result, researchers have turned to lightweight alloys, such as aluminium and magnesium alloys, as alternatives to steel in manufacturing car body structures due to their lower density. However, these alloys often fail to simultaneously satisfy the demands for economic benefits and structural strength due to inherent drawbacks. Metal composites, in comparison to traditional materials, exhibit superior performance and offer significant potential in design and applications [Citation1,Citation2]. Fe/Al composite materials possess numerous advantages, as they combine the high strength, hardness, toughness, and corrosion resistance of steel with the low density and good ductility characteristics of aluminium [Citation3]. Currently, many researchers add aluminium elements to steel through the method of smelting [Citation4,Citation5]. The materials obtained by this method usually have higher strength, but the smelting process causes loss of aluminium elements, and the amount of added aluminium elements is limited [Citation6,Citation7].
Jawad et al. [Citation8] reported an ultimate tensile strength of 440 MPa for multilayer steel/aluminium composite plates manufactured using the ARB process. Taali et al. [Citation6] demonstrated a higher ultimate tensile strength of 519 MPa and a total elongation of 13.6% for cold-rolled multilayer steel/aluminium composite plates. These findings highlight the effectiveness of Fe/Al laminated composites in preparing lightweight materials. The performance of Fe/Al laminated composites not only depends on the properties of the constituent materials but also on the bonding interface. The principles governing this interface involve the mechanical bonding of adjacent metals under pressure and the diffusion between different elements, leading to the formation of diffusion layers at high temperatures [Citation9,Citation10].
Previous studies have mainly employed rolling processes, including hot rolling and cold rolling, to produce Fe/Al laminated composites. In the cold rolling process, mechanical bonding occurs between the steel and aluminium layers. Wang et al. [Citation11] found that when the cold rolling deformation is below 35%, a wavy interface primarily forms through mechanical bonding. As the deformation increases to 50%, a thin diffusion layer consisting of Al and Fe atoms forms at the interface. In contrast, the hot rolling process involves both mechanical and metallurgical bonding between the steel and aluminium. The increased heat activates greater diffusion behaviour between the metals, leading to the formation of IMCs at the interface [Citation12]. Deng et al. [Citation13] utilized hot rolling to prepare Fe/Al laminated composites and achieved different forms of IMCs at the interface through heat treatment. Their results showed that the layered IMCs could diminish the strength of the interface bonding. Mendes et al. [Citation14] investigated multilayer Fe/Al composites prepared by accumulative roll bonding (ARB) and asymmetrical accumulative roll bonding (AARB). Their findings revealed that the introduction of shear force through rolling strengthened the mechanical bonding, and a rolling temperature of 400°C yielded atomic diffusion layers at the interface. However, this conventional method often generates significant shear stress and makes it challenging to control the formation of IMCs, leading to potential fracture of the metal layers in the composite material [Citation8]or weakening the interfacial bonding [Citation15].
Metallurgical bonding of dissimilar metals can be achieved through the joint action of temperature and pressure in the process of hot pressing. Que et al. [Citation16] prepared Al/Al-TiB2 composite materials with different layers by hot pressing sintering method, and the test results showed that the Al/Al-TiB2 interface was well combined without obvious micropores, cracks and other defects. In addition, it is found that the soft Al layer has the function of transferring strain. Due to crack deflection, interfacial delamination and strain transfer in lamellar composites, the plasticity of the whole material increases. Wang et al. [Citation17] used vacuum isothermal heating to prepare steel/Al laminated composites under 655°C and 3.8MPa pressure. The results showed that the composite obtained by this method had dense microstructure and showed good compressive strength. The introduction of new interfaces during hot pressing deformation enhances diffusion behaviour but also leads to an increased formation of dislocations [Citation18,Citation19]. These dislocations can expedite the formation of IMCs. Therefore, it is important to investigate the control of material properties by regulating the degree of hot pressing deformation.
In this study, Fe/Al laminated composites with varying compression rates were fabricated using the hot press method to examine the impact of deformation on the performance of layered Fe/Al composites. The mechanical properties of the material were examined both at the macroscopic and microscopic levels, and the alterations in performance were elucidated by integrating microscopic morphology analysis. Additionally, finite element simulation using ABAQUS software was employed to gain a more comprehensive understanding of the stress-strain behaviour of the material post-deformation.
Experimental procedure
Materials and methods
In this study, 6061 aluminium alloy and 316 L stainless steel were employed as the raw materials. The chemical composition of the materials can be found in . The thickness of 6061 aluminium is 0.8 mm, while the thickness of 316 L stainless steel is 0.5 mm. Both materials were then shaped into circular discs with a 30 mm diameter. The physical parameters of the materials can be seen in .
Table 1. Main physical parameters of materials.
To ensure the cleanliness of the material contact interface, the steel plates underwent an acid-washing process using a 5% volume fraction of hydrochloric acid to eliminate the oxide layer. Subsequently, both the steel and aluminium plates were polished with 600 grit sandpaper. To remove any remaining moisture and dust particles, they were cleaned in anhydrous ethanol for 10 minutes using ultrasound. The pressing process was promptly conducted after the surface treatment to prevent the formation of new oxides. The normal direction (ND) thickness after stacking is 3.1 mm. The calculated theoretical density is 5.16 g·cm−3. A stack of aluminium and steel sheets, as shown in , was placed in a press and cold-pressed at room temperature with a force of 10t for 30 minutes. This ensured the compaction of the sample and the expulsion of any trapped air between the layers, effectively preventing the formation of oxides during subsequent processes.
Following that, a heating coil was employed to raise the sample’s temperature to 400°C. Hot pressing was performed with pressures of 21.6t, 28.8t, and 36t, corresponding to pressures of 300, 400, and 500 MPa, respectively, to achieve different levels of deformation. Once the process was completed, the sample was carefully removed. Measurements revealed that the average deformation amounts under different pressures were 17%, 32%, and 38%, respectively.
The tensile samples were cut along the rolling direction (RD) using wire electrical discharge machining (WEDM), as depicted in . For each condition, three samples were tested, and the standard deviation of the results was found to be less than 5%. Tensile experiments were conducted at room temperature with a strain rate of 0.5 mm/min.
Material characterization
The interface of the Fe/Al composite plate was characterized and analysed using a scanning electron microscope (SEM) equipped with an energy-dispersive spectroscopy (EDS) system. Metallographic samples, taken parallel to the rolling direction, were prepared for observation with the scanning electron microscope. The preparation involved a sequence of polishing steps, starting from coarse sandpaper to finer grit, followed by polishing with alumina suspension
The nanoindentation device was employed to concurrently measure the nanoscale Young’s modulus (EN) and nanoscale hardness (HN). The nanoindentation test employed a 3D mapping technique with a maximum test load of 50 mN. During the 3D mapping measurement process, a total of 225 points were tested at 10 µm intervals within a 150 µm x 150 µm area. This approach allowed for the determination of the variation in interface hardness and Young’s modulus at different positions, which were subsequently analysed.
Finite element simulation
Using the commercial software ABAQUS, we utilized the finite element method (FEM) to create a model of the hot pressing process for the material. This model effectively captures the comprehensive stress and strain experienced by the composite material throughout the manufacturing process. The constant parameters in the model have been established based on experimental findings. Through the analysis of the composite material’s production process, we investigate the impact of these procedures on its internal properties.
Results and discussion
Composite interface microstructure
depict the morphologies observed at the Fe/Al interface of samples subjected to various compression rates during hot pressing. It is evident that the shape of the interface transitions from flat to curved as the compression rate increases. The growth of the IMC layer is also observed. Specifically, at a compression rate of 17%, the sample interface appears to be flat, with scattered IMC present. In contrast, at a compression rate of 32%, the interface exhibits a slight curvature, and continuous IMC layers begin to appear. Notably, a distinct IMC layer is formed when the compression rate reaches 38%. Analysis of the interface scanning results reveals that the aluminium content at the interface increases with the compression rate, indicating a higher diffusion activity of aluminium atoms compared to iron atoms. This can be attributed to the higher diffusion rate of Al atoms in the IMC, allowing them to penetrate the initially formed IMC and form new IMC layers [Citation20]. Considering the compositional ratio, it is plausible to suggest that the IMC present in the sample with the highest compression rate is FeAl3. Amanollahi [Citation21] observed FeAl3 in cold-rolled samples after a 500°C heat treatment. In previous studies [Citation13,Citation20,Citation22], IMC formation was typically not observed under heat treatment conditions below 400°C. However, the pressure is maintained throughout the heating process, resulting in a reduction in interatomic distance and facilitating diffusion, thus promoting metallurgical bonding formation at the interface. Furthermore, as the compression rate during hot pressing increases, the volume of IMC also increases. This can be attributed to Assari’s assertion [Citation19] that an increased number of dislocations, caused by deformation, creates more favourable conditions for interdiffusion. Moreover, the higher compression rate leads to greater lateral extension, resulting in the rupture of the original oxide film on the metal surface. This, in turn, leads to the formation of additional fresh metal surfaces at the interface, providing more favourable regions for diffusion.
Tensile properties and fracture morphology
The tensile properties of samples subjected to different compression rates are illustrated in and . When the compression rate is set at 32%, the sample achieves the highest tensile strength of 467 MPa, which is comparable to that of the sample compressed at 38%. When the specific strength is about 91 MPa·cm3·g−1, the elongation reaches 2.5 times that of the steel/aluminium laminated composite prepared by Saeed et al. [Citation6]And the overall strength is greater than the results of Jawad et al. [Citation8]Initially, an increase in the compression rate leads to a rise in tensile strength, followed by a decline. Moreover, the yield strengths of these samples are essentially identical and greater than that of the sample compressed at 17%. As the compression rate increases, the elongation of the samples decreases, with the most significant reduction observed at a compression rate of 38%. This is attributed to the prominence of work hardening as a result of increased deformation caused by hot pressing, consequently enhancing strength [Citation23]. In addition, the change of mechanical properties may also be related to the change of the matrix microstructure and the interface morphology during machining. shows the grain change of the steel/aluminium composite after hot pressing. The steel has obvious rolling texture along the RD direction after deformation, which is due to the uneven plastic deformation of the metal during the rolling process, which leads to stress concentration and strain gradient inside the metal. These stress concentrations and strain gradients cause the grains inside the metal to deform and rearrange. Elongated grains in the RD direction can be observed in aluminium, but no other obvious changes occur due to excellent plasticity.These changes also have an effect on mechanical properties.However, once the compression rate is further increased, the formation of a distinct IMC occurs at the interface, with certain regions showing an enrichment of IMC (). Due to the weak bonding between IMC and aluminium, as well as the brittle nature of IMC, cracks are prone to forming and the material’s ability to deform is limited [Citation24]. During the plastic deformation stage, the interface experiences stress concentration, leading to cracking at vulnerable locations [Citation25], ultimately resulting in material fracture. Despite the fact that the sample compressed at 38% theoretically possesses higher strength, the presence of interface defects prevents any further improvement in strength and leads to premature fracture. Another notable observation is that the tensile strength of the entire stress-strain curve only experiences a decrease at the point of overall fracture, indicating that steel and aluminium undergo cooperative deformation without any single-layer fracture occurring prior to fracture. This demonstrates the strong bonding of the interface, which can withstand the shear stress generated during tensile deformation, highlighting their excellent bond.
Figure 6. (a) 316 L initial microstructure, (b) 316 L hot pressed after 32% reduction rate, (c) 6161 initial micro structure, (d) 6061 hot pressed after 32% reduction rate.

illustrates the fracture morphology of the samples. At lower compression rates, both Fe and Al exhibit numerous dimples on their fracture surfaces, indicating ductile fracture. However, in the samples subjected to higher compression rates, a mixed fracture comprising both ductile and brittle fracture is observed. Additionally, tongue-shaped depressions, which are characteristic of intergranular fracture, can be observed on the fracture surfaces of both metals. The number, size, and depth of the dimples vary due to the difference in ductility between the two metals. Notably, the size of the dimples decreases with increasing deformation. This reduction can be attributed to work hardening during plastic deformation, an occurrence that leads to an increase in dislocation density within the metal crystals. As a result, the dimples at the fracture surface undergo a refinement.
Figure 7. Fracture morphology of samples with different reduction rates(a1)Macroscopic fracture morphology of 17% reduction rate;(a2)Aluminum side(a3)Steel side,(b1)Macroscopic fracture morphology of 32% reduction rate;(b2) aluminum side(b3)Steel side,(c1) macroscopic fracture morphology of 38% reduction rate;(c2) aluminum side(c3)Steel side.

depicts the fracture process of the layered Fe/Al composite material. Initially, cracks emerge on the IMCs and subsequently expand rapidly under shear stress, ultimately resulting in fracture. The necking phenomenon is observed on both a global and local scale, which accounts for the presence of gaps between layers on the macroscopic tensile fracture surface. Interfacial delamination and the tortuousness of cracks can impede crack propagation, as evidenced by Liu et al.‘s study [Citation26]. Layered composite materials are particularly susceptible to delamination when the bonding strength is low. Therefore, the sample subjected to a compression rate of 17% may possess improved plasticity not only due to diminished work hardening effects, but also hindrance of crack propagation. Additionally, the IMCs observed in do not compromise interlayer bonding; instead, they function as potential crack initiators at the steel and aluminium interfaces. Consequently, enhanced bonding increases the probability of crack propagation between layers.
Finite element simulation
The method of finite element simulation can be used to monitor and predict the parameters in the plastic forming process. In this paper, a three-dimensional model is established using the ABAQUS finite element analysis software to observe the stress-strain changes in the steel-aluminium composite process. The established model is shown in , with the deep green part in the figure representing 316 L, the grey-white part representing 6061, set as plastic deformation bodies, with a hexahedral mesh type and a global size of 1 mm; the light green parts represent the punch and die, set as rigid bodies, with a tetrahedral mesh type and a global size of 6 mm. Constant forward loads of 21.6t, 28.8t, and 36t are applied to the punch. The thermal properties parameters of steel and aluminium are set as listed in .
Table 2. Material thermal property parameters.
presents theFEMresults depicting the distribution of Mises stress and true strain within samples possessing varying compression ratios. The Mises stress distributions for samples compressed at ratios of 17%, 32%, and 38% are denoted by ‘a’, ‘b’, and ‘c’, respectively. Similarly, the true strain distributions for samples compressed at ratios of 17%, 32%, and 38% are represented by ‘d’, ‘e’, and ‘f’, respectively. The Mises stress is determined by evaluating the principal stresses in three directions, as formulated in EquationEquation (1)(1)
(1) .
Figure 10. (a) Mises stress distribution with a reduction rate of 17%,(b) Mises stress distribution with a reduction rate of 32%,(c) Mises stress distribution with a reduction rate of 38%,(d) The true strain distribution with a reduction rate of 17%,(e) The true strain distribution with a reduction rate of 32%,(f) The true strain distribution with a reduction rate of 38%.

The Von Mises stress σand the first σ1, second σ2, and third σ3 principal stresses are considered in this study. It is observed that the deformation degrees of aluminium and steel are not consistent after hot pressing. Compared to steel, aluminium demonstrates better plasticity and undergoes more deformation at the same temperature, which is in line with experimental findings. This difference in deformation results in shear forces at the interface, which directly impact the bonding effect [Citation27,Citation28]. During the hot pressing stage, shear forces cause grain refinement near the interface, leading to an increase in dislocation density and favourable conditions for diffusion [Citation19]. However, in regions of peak stress, interface cracking may occur, highlighting the need to control the existence of shear stress. While the downward movement of the pressing head is uniform, the stress and deformation behaviour of different material parts vary [Citation29]. Along the unconstrained RD direction, tensile strain is uniformly distributed along the material edge. In the case of a compression ratio of 38%, there is also inward tensile strain in the steel layer on the surface of the sample. On the other hand, in the ND direction, compressive strain is not uniformly distributed on the surface. The material edge experiences significantly higher compressive strain compared to the central region, which may be attributed to the difference in deformation ease. The regions near the centre are constrained in all directions, making deformation more difficult, whereas the regions near the edge can freely extend outward, facilitating compressive deformation. This uneven distribution of stresses explains why the centre of the material and the material edge experience more pronounced stresses. The central region’s extension is consistently impeded by the surrounding area, while the high stress level in the edge region results from the shear stress caused by the deformation difference between aluminium and steel. The simulation results also indicate that although the soft aluminium layer deforms the most, it does not generate significant stress. The steel layer, on the other hand, experiences a greater impact from different compression ratios, including changes in internal stress levels and structural properties. Overall, the change in material performance is influenced not only by IMCs and shear stress at the interface but also by modifications in the steel matrix’s properties.
Nanoindentation test
) illustrates the distribution of the nano-Young’s modulus (EN) at the sample interface with reduction rates of 17%, 32%, and 38%. Slight variations are observed in the test regions due to microstructural defects, such as pores and cracks, which can lead to fluctuations in EN and hardness. However, overall, the EN results are relatively uniform for both the steel and aluminium sides. Interestingly, as deformation increases, the average EN of the steel side shows an increment of about 20 GPa, while the EN of the aluminium side exhibits a slight increase as well. These findings suggest an overall enhancement in performance compared to the original material as presented in . Notably, in the two control groups with reduction rates exceeding 30%, the overall EN remains similar. This implies that dislocation density and grain refinement play a significant role in influencing EN. This effect is particularly evident in the steel layer, indicating that performance changes on the steel side dominate the tensile properties of the entire material. On the other hand, Because of the excellent plasticity of aluminium, the change of ENis not obvious after the deformation of the overall composite material. Furthermore, there is a distinct transition layer between the aluminium layer and the steel layer, characterized by a difference in modulus, which suggests the occurrence of diffusion and the formation of IMCs between aluminium and steel. The presence of this diffusion layer demonstrates excellent interfacial bonding effects.
Figure 11. Contours of modulus distribution at Fe/Al interface of samples with different reduction rates,(a) 17%;(b) 32%;(c) 38%.

) depict the nano-hardness (HN) at the sample interface with reduction rates of 17%, 32%, and 38%. It is evident from that the HN value of the steel layer exceeds that of the aluminium layer. Furthermore, as the hot pressing deformation increases, the HN of both layers also shows an upward trend. The hot pressing compounding process of the metal composite plate introduces friction between the metal layers at the composite plate interface, exerting a shear force that enhances the dislocation density at each layer interface. This phenomenon results in strain hardening and enhances the HN value at the interface effectively. Although non-uniformity in deformation and stress exists at different positions within the material, overall, the deformation of each layer increases with the progression of hot pressing deformation. Additionally, as deformation increases, the increment in HN amplitude of the steel layer surpasses that of the aluminium layer significantly. Specifically, after increasing the deformation, the maximum HN value of the steel layer rose by 0.74 GPa, while the aluminium layer showed a relatively small change. These findings suggest that variation in reduction rate primarily influences the physical properties of the steel, while aluminium primarily affects the overall material performance through interfacial diffusion.
Conclusion
The exploration of Fe/Al composite materials through hot pressing is the primary focus of this study. Tensile strength, microphysical properties, and interface microstructure are thoroughly investigated. By incorporating the results of finite element simulations, the study reveals the variations in internal stress within the Fe/Al layered composite material under different reduction rates. Based on these findings, the following conclusions can be deduced:
Fe/Al layered composite materials, prepared through hot pressing, exhibit commendable mechanical properties. The sample obtained at 400°C with a reduction rate of 32% demonstrates a tensile strength of 467 MPa and an elongation of 34%.
Increased reduction rates facilitate the mutual diffusion of Fe and Al atoms, thereby effectively enhancing the interface bonding effect.
The presence of IMCs does not necessarily weaken the interface bonding; however, it does serve as a potential source for cracks at the interface, ultimately resulting in material fracture.
The overall improvement of the material’s performance is predominantly dictated by the changes in the performance of the steel matrix itself.
Disclosure statement
No potential conflict of interest was reported by the author(s).
References
- Czerwinski F. Current trends in automotive lightweighting strategies and materials. Materials. 2021;14(21):6631. doi: 10.3390/ma14216631
- Heggemann T, Homberg W. Deep drawing of fiber metal laminates for automotive lightweight structures. Compos Struct. 2019;216:53–10. doi: 10.1016/j.compstruct.2019.02.047
- Assari AH. Investigating the deformation behavior of hot-pressed Ti/Al/Ti laminated composite. J Manuf Processes. 2023;95:369–381. doi: 10.1016/j.jmapro.2023.04.026
- Wei LL, Gao GH, Kim J, et al. Ultrahigh strength-high ductility 1 GPa low density austenitic steel with ordered precipitation strengthening phase and dynamic slip band refinement. Mater Sci Eng A. 2022;838:142829. doi: 10.1016/j.msea.2022.142829
- Wei LL, Gao GH, Misra RDK. Phenomenological understanding of kappa-carbides on strengthening mechanism of low-density steel. Mater Sci Technol. 2023;39(12):1463–1474. doi: 10.1080/02670836.2023.2173430
- Taali S, Toroghinejad MR, Saeidi N. Architectured lightweight steel composite: evaluation of the effect of geometrical parameters and annealing treatments on deformation behavior. J Mater Res Technol. 2021;15:5414–5427. doi: 10.1016/j.jmrt.2021.10.121
- Song C, Wang H, Sun Z, et al. A new hot-rolled lightweight steel with ultra-high strength and good ductility designed by dislocation character and transformation strain. Scripta Materialia. 2022;212:114583. doi: 10.1016/j.scriptamat.2022.114583
- Jawad DH, Hosseinzadeh A, Yapici GG. Effect of layer architecture on the mechanical behavior of accumulative roll bonded interstitial free steel/aluminum composites. Mater Sci Eng A-Struct Mater Prop Microstruct Process. 2021;818:9. doi: 10.1016/j.msea.2021.141387
- Yang X, Weng H, Tang C-L. Interfacial Bonding Mechanism Ofaluminium and Steel Composites. Adv Compos Lett. 2018;27(2). 096369351802700. doi: 10.1177/096369351802700203
- Qin L, Fan M, Guo X, et al. Plastic deformation behaviors of Ti-al laminated composite fabricated by vacuum hot-pressing. Vacuum. 2018;155:96–107. doi: 10.1016/j.vacuum.2018.05.021
- Wang C, Jiang Y, Xie J, et al. Interface formation and bonding mechanism of embedded aluminum-steel composite sheet during cold roll bonding. Mater Sci Eng A. 2017;708:50–59. doi: 10.1016/j.msea.2017.09.111
- Wang Y, Du J, Xiao H. Interfacial evolution and coordinated deformation mechanism of Ti/Al laminated metal composites prepared by diffusion bonding. J Mater Res Technol. 2023;27:4541–4551. doi: 10.1016/j.jmrt.2023.10.264
- Deng Z, Xiao H, Yu C. Effect of intermetallic compounds distribution on bonding strength of steel–aluminum laminates. Mater Design. 2022;222:111106. doi: 10.1016/j.matdes.2022.111106
- Mendes A, Timokhina I, Molotnikov A, et al. Role of shear in interface formation of aluminium-steel multilayered composite sheets. Mater Sci Eng A. 2017;705:142–152. doi: 10.1016/j.msea.2017.08.025
- Dai G, Liu Y, Chen K, et al. Effect of cumulative deformation and interlayer mechanical properties on bonding strength of corrugated interface steel/aluminum composite plate. J Manuf Processes. 2023;103:78–89. doi: 10.1016/j.jmapro.2023.08.028
- Que B, Chen L, Chen Y, et al. Fabrication of Al/Al-TiB2 laminate composites via hot press sintering process: an insight into the mechanical properties and fracture behavior. J Manuf Processes. 2024;109:53–64. doi: 10.1016/j.jmapro.2023.11.040
- Wang Y, Liu Y, Pan H, et al. Phase formation and mechanical properties of iron-based intermetallic/steel laminate composites Adv Compos Hybrid Mater. 2021;5(3):2171–2183. doi: 10.1007/s42114-021-00264-7
- Assari AH, Eghbali B. Interfacial layers evolution during annealing in ti-al multi-laminated composite processed using hot press and roll bonding. Met Mater Int. 2016;22(5):915–923. doi: 10.1007/s12540-016-5647-z
- Assari AH, Eghbali B. Solid state diffusion bonding characteristics at the interfaces of Ti and Al layers. J Alloys Compd. 2019;773:50–58. doi: 10.1016/j.jallcom.2018.09.253
- Slapakova M, Fekete R, Kralik K, et al. The influence of surface on direction of diffusion in Al-fe clad material. Mater Charact. 2022;190:112005. doi: 10.1016/j.matchar.2022.112005
- Amanollahi A, Ebrahimzadeh I, Raeissi M, et al. Laminated steel/aluminum composites: improvement of mechanical properties by annealing treatment. Mater Today Commun. 2021;29:102866. doi: 10.1016/j.mtcomm.2021.102866
- Springer H, Kostka A, Payton EJ, et al. On the formation and growth of intermetallic phases during interdiffusion between low-carbon steel and aluminum alloys. Acta Materialia. 2011;59(4):1586–1600. doi: 10.1016/j.actamat.2010.11.023
- Eizadjou M, Kazemitalachi A, Daneshmanesh H, et al. Investigation of structure and mechanical properties of multi-layered Al/Cu composite produced by accumulative roll bonding (ARB) process Compos Sci Technol. 2008;68(9):2003–2009. doi: 10.1016/j.compscitech.2008.02.029
- Zhang X, Gao K, Wang Z, et al. Effect of intermetallic compounds on interfacial bonding of Al/Fe composites. Mater Lett. 2023;333:133597. doi: 10.1016/j.matlet.2022.133597
- Gao BX, Zou DK, Guo YC, et al. Numerical simulation and experimental study on the effect of bond strength on the formability of steel/aluminum composite panels. Eng Fract Mech. 2022;276:108919. doi: 10.1016/j.engfracmech.2022.108919
- Liu HS, Zhang B, Zhang GP. Enhanced toughness and fatigue strength of cold roll bonded Cu/Cu laminated composites with mechanical contrast. Scripta Materialia. 2011;65(10):891–894. doi: 10.1016/j.scriptamat.2011.08.001
- Tamimi S, Gracio JJ, Lopes AB, et al. Asymmetric rolling of interstitial free steel sheets: microstructural evolution and mechanical properties. J Manuf Processes. 2018;31:583–592. doi: 10.1016/j.jmapro.2017.12.014
- Koga N, Suzuki S, Jiang H, et al. Characterization of heterogeneous–nano structure in austenitic stainless steel: crystal orientations and hardness distribution. J Mater Sci. 2020;55(22):9299–9310. doi: 10.1007/s10853-020-04643-1
- Watanabe C, Kobayashi S, Aoyagi Y, et al. Heterogeneous nano-structure and its evolution in heavily cold-rolled SUS316LN stainless steels. ISIJ Inter. 2020;60(3):582–589. doi: 10.2355/isijinternational.ISIJINT-2019-445