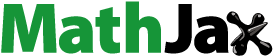
ABSTRACT
An innovative strategy was formulated to overcome the challenges in delivering doxorubicin (DOX) for breast cancer treatment. Transdermal patches were prepared using carbopol 971 (CP) and polyvinyl alcohol (PVA) loaded with starch-coated iron oxide nanoparticles conjugated to DOX (DOX@St-IONPs). The patches’ physicochemical properties, in vitro drug diffusion, alongside the physical properties of DOX@St-IONPs, and their impact on breast cancer cell lines’ viability were examined. The M1 patch, with a 1:1 CP:PVA ratio and DOX@St-IONPs, showed unique properties, indicating effective delivery. It maintained consistent thickness, weight, and 97% DOX loading. Demonstrated flexibility and acceptable surface pH. M1 displayed fivefold increased drug penetration compared to free DOX patches (flux of 14.85 µg/cm2/h and permeability of 0.95 cm/h), with higher cytotoxicity against triple-negative breast cancer. These results propose promising non-invasive DOX delivery through the developed patches.
Introduction
One of the most used chemotherapeutic drugs, doxorubicin (DOX), often requires invasive intravenous administration for the treatment of various malignancies [Citation1]. However, systemic DOX delivery presents several drawbacks, including dose-dependent cardiotoxicity, low bioavailability, and the need for high doses. To overcome these limitations, researchers have explored non-invasive transdermal delivery that offers many advantages such as bypassing first-pass metabolism, reducing gastric discomfort, and minimizing systemic toxicity [Citation2,Citation3]. Nonetheless, DOX is a hydrophilic drug with high molecular weight, posing challenges for effective skin penetration. To address this, many approaches have been employed to enhance DOX’s skin permeation and targeting abilities including liposomes [Citation4], nanoemulsions [Citation5], microneedle [Citation6], hydrogels [Citation7], iron oxide NPs [Citation8], gold NPs [Citation9], and zinc oxide NPs [Citation10]. Among all approaches, iron oxide nanoparticles (IONPs) have garnered significant attention due to their wide range of therapeutic applications, including vaccine delivery, target gene delivery, and magnetic resonance imaging [Citation11]. IONPs possess remarkable advantages making them an ideal option for delivering anticancer drugs [Citation12]. IONPs are biocompatible and have low toxicity, making them safer for medical applications compared to other magnetic compositions [Citation13]. IONPs have a high drug loading capacity, enabling efficient encapsulation and delivery of DOX [Citation14]. In addition, IONPs possess robust magnetic properties, enabling them to be directed by an external magnetic field to specific sites in vivo, thereby improving the targeted delivery of therapeutic agents to their intended locations. Moreover, the size and surface coating of NPs play pivotal roles in influencing the behaviour of those nanosystems, significantly affecting their effectiveness in therapy and diagnosis [Citation15,Citation16]. Surface modification techniques can be employed to make drug release responsive to different stimuli, including variations in temperature, redox state, or pH, enhancing the precision and control of drug administration. Furthermore, IONPs generate localized heating to selectively treat specific tumour tissues while minimizing damage to surrounding normal tissues, as it avoids significant overall temperature elevation [Citation17]. Moreover, targeted drug delivery can be achieved by conjugating IONPs with targeting agents that can interact specifically with disease markers, further enhancing the specificity and efficacy of treatment. Thus, IONPs can be functionalized to target specific cancer cells, enhancing the efficacy of DOX delivery while minimizing off-target effects [Citation14]. However, the synthesis of IONPs encounters several hurdles, such as the high cost involved in the process, the stability, and the challenge of controlling particle size. Ultrasmall IONPs (smaller than 10 nm, particularly those with sizes around 2.3 to 4.2 nm) face elimination through the kidneys, exhibit significant toxicity and can be lethal even at low dosages. Certain doses of IONPs can induce rapid haemolysis and lead to animal death. While those larger than 100 nm risk being engulfed by the reticular endothelial system [Citation18]. Additionally, coating NPs may result in a reduction of their magnetic properties. Moreover, the toxicity of metallic nanomaterials varies depending on factors like oxidation state, ligands, solubility, morphology, and individual health conditions. Thus, green strategies have gained momentum for IONPs synthesis with superiority over physical and chemical methods [Citation19]. Particularly, the biosynthesis of metallic and metallic oxide NPs from biowaste has captured attention by utilizing green waste and reducing reliance on expensive chemicals [Citation20]. These green-mediated NPs not only consume fewer resources but also exhibit exceptional biomedical properties, such as anticancer and antimicrobial activities, enhanced stability, cost-effectiveness, eco-friendliness, suitable particle size and longevity [Citation18]. By harnessing the power of biological molecules, green synthesis removes harmful compounds, resulting in environmentally sustainable and pollution-free methods [Citation21].
Banana peels, often discarded after consuming the pulp, have emerged as a straightforward green technique for synthesizing various metallic nanoparticles [Citation22]. Researchers have successfully utilized banana peel extract to create gold NPs [Citation23], silver NPs [Citation24], and iron oxide NPs [Citation25].
Therefore, the primary goal of this research is to fabricate a non-invasive transdermal patch integrating environmentally friendly synthesized IONPs loaded with DOX, with the specific aim of improving the delivery of DOX for breast cancer treatment. The envisioned outcome of this approach is to synthesis novel stable IONPs with ideal particle size of (10–100 nm) to enhance the skin penetration and cytotoxic effectiveness of DOX delivery for individuals undergoing breast cancer treatment.
Method
Materials
Starch and ferric chloride (FeCl3) were purchased from Aldhaher Company (Amman, Jordan). Doxorubicin hydrochloride from Biosynth Carbosynth (San Diego, U.S.A.) was purchased from AL Sabbagh Drug Store (Amman, Jordan). Polyvinyl alcohol (PVA) and Poly(ethylene-co-vinyl acetate) (EVA) were purchased from Sigma-Aldrich (St. Louis, U.S.A.). Carbopol 971P (CP) was purchased from B.B.C. Chemicals (China), Glycerol; Purified from Lobachemie (India), and dichloromethane from Laboratory Chemicals (Amman, Jordan). Distilled water (DW) was utilized through the experiments.
Bananas peels collection and extract preparation
Unripe green bananas were selected, cleaned, and their peels were removed. These peels underwent a cleaning process using distilled water (DW) and were subsequently subjected to drying in an oven at 140°C for a duration of two hours, as described by [Citation26]. Following this, the dried peels were finely ground into a powder using a blender.
To prepare the aqueous peel extract, 4 grams of the powdered peels were combined with 400 mL of distilled water and placed on a hot plate at a temperature of 90°C for 30 min. After the heating process, the extract was filtered and then subjected to centrifugation at a temperature of 6°C for 10 min, at a speed of 5000 revolutions per minute (rpm). The resulting supernatant was carefully separated and underwent subsequent drying in an oven at 80°C for a duration of 12 hours.
Chemical characterization of banana peel extract
Existence of tannins in the banana peel extract was revealed by the presence of a dirty-green precipitate upon adding two drops of 5% FeCl3 to 1 mL of the aqueous peel extract [Citation27]. At the same time, flavonoids were detected by the pale brown colouration upon adding three drops of ammonia solution, followed by 0.5 mL concentrated hydrochloric acid and ethanol to 1 mL of the aqueous extract [Citation27].
Preparation of IONPs
IONPs were prepared using banana peels extract and varying concentrations of FeCl3 concentrations (0.01, 0.1, 0.6, and 0.9 M). Five volume ratios (1:1, 2:1. 3:1, 4:1, and 6:1) of banana peels extract and FeCl3 were prepared. The mixtures were left at room temperature for half an hour to react. Then, 2% of starch dispersion was added to prepare starch-coated IONPs (St-IONPs). They were mixed for 20 min using a magnetic stirrer at room temperature, followed by sonication for 40 min and 10 min of centrifugation at 5000 rpm and 5°C. The percentage yield was estimated using Equationequation (1)(1)
(1) :
Preparation of DOX conjugated to starch-coated IONPs (Dox@st-IONPs)
To prepare DOX@St-IONPs), 2 mg of DOX was introduced into a solution containing 20 mL of IONPs and 2.5 mL of a 2% starch dispersion. This blend underwent 35 minutes of agitation at room temperature using a magnetic stirrer, followed by a 40-minute sonication process. Afterward, centrifugation was carried out at 5°C, spinning at 5000 rpm for 20 minutes. The resulting supernatant underwent drying at 80°C for 4 hours, resulting in the formation of DOX@St-IONPs.
The loading capacity was determined by measuring the UV absorbance at 480 nm of the unconjugated free DOX found in the supernatant. This measurement was performed following a 20-minute centrifugation at 5°C and 5000 rpm of the dissolved DOX@St-IONPs in phosphate-buffered saline (PBS) at a pH of 7.4, as reported by [Citation28]. EquationEquation (2)(2)
(2) is applied to determine the loading capacity alongside constructed calibration curve for DOX in PBS at pH 7.4. The plot was created employing six calibration standards with concentrations ranging from 4.8 to 12.8 µg/mL, derived from diluting the initial stock solution with a concentration of 40 µg/mL.
Characterization of IONPs
The particle size, polydispersity index (PDI), and zeta potential of IONPs, St-IONPs, and DOX@St-IONPs were assessed using zeta sizer analyser (NANO ZS-001, Malvern, UK). Specific volume ratios were selected to attain optimal particle size with the lowest PDI values for further investigation.
Additionally, a UV-visible spectrophotometer (UV/VIS-002, Shimadzu, Japan) was employed to determine the characteristic absorption peak. Alongside, the crystallinity of IONPs was examined by subjecting them to diffracted electromagnetic X-ray waves with frequencies ranging from 10 to 0.01 nm and energies spanning from 120 eV to 120 KeV, with the screening process conducted at a rotation rate of 3° per minute.
Furthermore, a Fourier transform infrared spectrophotometer (FTIR) was utilized to identify the chemical functional groups present in the components used, shedding light on the proposed mechanisms of interactions between them.
Anticancer activity
The anticancer activity of the banana peels extract, IONPs, St-IONPs, and DOX@St-IONPs against triple-negative breast cancer cells (TNBC-MDA-mb 231) was investigated. The Dulbecco’s modified eagle medium (DMEM) with and without cancerous cells, Free DOX (0.1 mg/20 mL DMEM media) and FeCL3 solution were used as controls. To ensure that all traces of old media were removed, tubes were washed with 5 mL Dulbecco’s-PBS. The cells were then detached from the flask surface with 2 mL of trypsin-EDTA applied to the flask surface. The flask was incubated in CO2 incubator for 2 min. To avoid the trypsin reaction during dilution, about 10 mL of fresh DMEM media was immediately added to the flask. The cells were transferred from the fresh media to a centrifuge tube designed to spin for 10 min at 2000 rpm in a centrifugation system. Cancerous cells were adsorbed on the tube wall, and the media was removed. Then, 10 mL of fresh DMEM media was added to the test tube to form a stock solution, which was mixed with 100 mL Trypan blue dye solution, shaken well, and applied to a particular slide covered with a coverslip for counting viable cancerous cells (colourless dots) under an inverted microscope. Subsequently, the cells were seeded in three culture plates (96 wells) and allowed to grow for 24 h in a CO2 incubator to get about (1800) cells in each well. All wells were treated in triplicate and added in a CO2 incubator for three hours. After that, solubilization solution/stop mix (G4101, Promega) was added, and the mixture was allowed to sit at room temperature for about an hour. Using a BioTek ELx800 absorbance microplate reader, the cell viability at two wavelengths (590 and 630 nm) was lastly assessed. The cytotoxicity half-maximal inhibitory concentration (IC50) was assessed from the sigmoidal curve fitting using Graph Pad, Prism 8 (San Diego, CA, U.S.A.). The IC50 was estimated using different serial concentrations of banana peel extract, IONPs, St-IONPs, DOX@St-IONPs, and DOX.
Preparation of transdermal patches
The transdermal patches were produced using the solvent evaporation method. Initially, polymers were dissolved in a chosen solvent to create a clear and thick solution, which was then evaporated to generate transparent films [Citation29]. The backing layer was formed by pouring 10 mL of 3% EVA solution in dichloromethane onto a Plexiglass Petri dish, with subsequent drying at room temperature. Meanwhile, the adhesive gel layer was prepared by combining 0.5% PVA and 1.5% CP aqueous solutions. The PVA solution involved dissolving 0.5 g of PVA in 100 mL of distilled water on a hot plate, while the CP solution was made by dissolving 1.5 g of CP in 100 mL of distilled water over 24 hours, including 0.45 g of glycerol. The CP and PVA solutions were mixed in different ratios (1:1 and 1:5, PVA: CP) to create four distinct gel mixtures (M1-M4). DOX@St-IONPs were incorporated into M1 and M2, whereas free DOX was loaded into M3 and M4 (). Each gel mixture was applied to the dried EVA, followed by oven drying at 38°C for 3 hours and an additional 5-day air drying period at room temperature.
Table 1. Composition of the medicated gelling layer of the patches (surface area = 24.62 cm2).
Characterization of the transdermal patches
Thickness, weight, and drug content uniformity
The thickness of ten patches was measured at five different positions using a digital micrometre, while the weight of each patch was individually determined using the ME204 Mettler Toledo balance from Switzerland. Subsequently, the averages of both the patch thicknesses and weights, along with their standard deviations, were recorded. Additionally, each patch was submerged in 10 mL of phosphate buffer (PB) at pH 7.4, held at 25°C, and stirred for 24 hours at 100 rpm. This process aimed to assess the drug content by subjecting the patches to UV-visible spectrophotometric analysis at a wavelength of 480 nm.
Folding endurance
The patch underwent repeated folding at a consistent point to assess its flexibility. The folding endurance value corresponds to the number of times the film could be folded before breaking [Citation29].
Surface pH of the patch
Ten patches were randomly chosen and placed into a beaker filled with 150 mL of phosphate buffer (PB) at pH 7.4. The surface pH of each patch was measured using a pH metre (pH-001, Eutec, Germany).
Swelling study
For the swelling analysis, another set of four randomly selected patches were weighed, attached to pre-weighed stainless-steel supports with specific sieve gaps, and submerged in a beaker containing 150 mL of PB (pH 7.4). This beaker was then placed in a water bath set at 32°C. At 10-minute intervals from 0 to 90 minutes, the supports were removed from the solution, placed on filter paper to eliminate excess solvent, and immediately re-weighed. The change in weight was used to calculate the percentage of swelling (hydration capacity) of the patches using Equationequation (3)(3)
(3) .
The weight at a specific time ‘t’ is labelled as Wt, with the initial weight denoted as W0. All measurements were performed three times, and the outcomes were presented as the mean value along with the standard deviation (SD).
In vitro diffusion studies
The in vitro analysis of DOX diffusion through a cellulose membrane was conducted employing Franz diffusion cells (Hanson, United States) that featured an orifice surface area of 1.77 cm2, as described by [Citation30]. The cellulose membrane employed had a molecular weight cut-off (MWCO) ranging from 12 to 14,000 Dalton and had a size of 7 Inf Dia, featuring a diameter of 23.8 mm. The diffusion trials were carried out at a temperature of 32°C, wherein the patches were positioned on the cellulose membrane so that the backing layer faced the donor compartment while the adhesive film faced the receiver compartment. The donor compartment consisted of various components such as the cell ring, glass cell disk, and dosage wafer. In contrast, the receiver compartment contained 8.3 mL of phosphate buffer (PB) with a pH value of 7.4, and a helix magnetic stirrer was used.
During the experiments, samples were automatically collected at specific time intervals (0.15, 1, 2, 4, 8, 12, 16, 20, and 24 hours). Each time, 2.4 mL of the sample was withdrawn and replaced with an equal volume of fresh receptor solution. A spectrophotometer, operating at a wavelength of 480 nm, was employed to determine the drug content of the collected samples. To ensure reliable results, the diffusion experiments were conducted in triplicate, assessing the influence of different polymer combinations and forms of drug loading on the release profile. Sink conditions were maintained to guarantee accurate measurements by dissolving the maximum amount of DOX loaded into the patch (with a surface area of 1.77 cm2) in the total volume of the solvent used in the receiver compartment at 32°C. Sink conditions were confirmed by adding 1.43 mg of DOX to 8 mL of PB (pH 7.4) at 32°C and verifying complete dissolution.
The study also investigated the unidirectional diffusion of DOX across EVA. One sample was extracted from the receiver compartment after a 24-hour interval, during which the patch was administered in the reverse orientation. In this configuration, the patches were affixed to the cellulose membrane with the adhesive layer facing the donor compartment and the backing layer oriented towards the receiver compartment. The percentage of drug that permeated the cellulose membrane was determined by dividing the total amount of diffused drug by the initial quantity of loaded drug.
Subsequently, the flux (Jss) was computed by analysing the gradient of the linear segment in the cumulative amount of drug that passed per unit surface area over time through the cellulose membrane. Moreover, the permeability coefficient (P) was calculated by dividing Jss by the initial drug concentration in the donor compartment (C0), using Equationequation (4)(4)
(4) as reported by [Citation30].
Results and discussion
Preparation of banana peel extract
The banana peel extract initially had a cloudy brown-yellow appearance, which became a transparent light yellow after filtration. The alteration in colour confirmed the presence of tannic acid and flavonoids in the extract [Citation27].
Preparation of IONPs
The addition of FeCl3 to the banana peel extract resulted in the immediate formation of a black colour. It is hypothesized that the interaction between flavonoids, phenolic compounds, and FeCl3 facilitated a redox reaction, leading to the formation of black-coloured IONPs [Citation31]. The combination of phenols and ferric ions led to the creation of a black complex [Citation32]. Moreover, increasing the ratio of peel extract to FeCl3 concentration led to a reduction in the particle size of IONPs. The optimal concentration of FeCl3 for producing IONPs with a size of <100 nm was found to be 0.9 M, with a volume ratio of banana peel extract to FeCl3 of 4:1, resulting in a yield of 73.19% [Citation31]. Similar observations were reported by [Citation33] who observed the formation of IONPs after a colour change from translucent red to black in Trigonella extract and from bright green to black in tomato extract.
Characterization of IONPs
Upon starch coating, the mean size of IONPs significantly reduced from 64.2 ± 0.9 nm to 18.2 ± 2.4 nm, with a similar PDI of 0.5. This size decrease rendered the St-IONPs smaller than those previously reported, ranging from 100 to 180 nm [Citation34]. Furthermore, the zeta potential of St-IONPs (−17.6 mV) surpassed that of uncoated IONPs (−16.9 mV). This has sparked considerable interest in starch due to its unique properties compared to traditional nanomaterials [Citation35]. It is hypothesized that starch shields the IONP surface, hindering aggregation through steric repulsion, leading to size reduction and a slight enhancement in stability, as evidenced by a slight increase in negative zeta potential [Citation36]. Additionally, most starch-coated nanoparticles exhibit homogeneous spherical shapes with excellent dispersibility [Citation37].
Additionally, DOX@St-IONPs demonstrated a reduced particle size and a lower negative zeta potential compared to DOX loaded onto uncoated IONPs (DOX@IONPs). DOX@St-IONPs exhibited a particle size of 38.1 ± 1.9 nm with a PDI of 0.5 and a zeta potential of −12.3 mV, while the uncoated DOX@IONPs had a larger particle size of 131.5 ± 1.8 nm with a PDI of 0.7 and a zeta potential of −9.2 mV. The positive charge of DOX affected the stability of IONPs, leading to a decrease in the negativity of the zeta potential. Starch coating was facilitated by the electrostatic interaction between the positively charged DOX and the anionic starch, resulting in a smaller particle size. The drug loading in DOX@St-IONPs was determined to be 84.4%, calculated using the linear calibration curve (y = 0.0411×) with a regression coefficient (R2) of 0.9999 at a wavelength of 450 nm [Citation38].
represents the FTIR absorption peaks of IONPs, St-IONPs, and DOX@St-IONPs. A distinctive peak at 538 cm−1 was observed in the IONPs spectrum, which could be assigned to the Fe – O bond vibration of Fe3O4, as reported previously at 530 cm−1 [Citation39]. Other absorption peaks were detected at 1074 cm−1 for – C – O stretching, 1600 cm−1 for – C=O stretching, and 2179 cm−1 for – CN stretching, which attributed to the phytochemicals present in the banana peel extract that stabilized the IONPs [Citation40]. The absorption bands at around 1309–1350 and 1400–1426 cm−1 were characteristic peaks of C=C in aromatics rings of banana peels extract, as previously reported [Citation41]. Moreover, the weak stretching peaks at 3014 and 3183 cm−1 were assigned to the C-H stretching mode, representing the aliphatic nature of the peaks, while the peak at 3406 cm−1 represents the O-H stretching [Citation42].
New absorption bands supported the presence of starch in the FTIR spectra of St-IONPs at 852, 1657, and 2961 cm−1. The peak at 852 referred to C-O vibration in the C-O-C groups, while 1657 cm−1 referred to O-H bending of water, and 2961 cm−1 was attributed to C-H bond stretching [Citation43]. In addition, the peak at 3406 cm−1 became broader and split into two peaks that were assigned to the O-H deformation of starch.
Chemisorption of starch onto the surface of IONPs occurred via hydroxyl groups (OH). The chemical interaction of the amine group of DOX with the hydroxyl groups on the sugar backbone of starch was postulated to occur through hydrogen bonding, in agreement with a previous study [Citation44]. Peaks positioned at 1641 and 3406 cm−1 were due to hydroxyl group (O – H) stretching and H – O – H vibrational bands due to adsorbed water molecules in the sample as the solvent was aqueous in all three cases. A shift was observed for the hydroxyl vibration of IONPs and St-IONPs from 1600 to 1610 cm−1, and the broad peaks cantered at 3344 cm−1 of St-IONPs was shifted to sharp peak at 3406 cm−1 in the case of DOX@St-IONPs, indicating that the NH2 group in DOX has interacted with the hydroxyl groups in the structure of starch.
represents the XRD diffractograms of St-IONPs and DOX@St-IONPs, showing the absence of characteristic diffraction peaks, suggesting their amorphous nature, consistent with findings reported by [Citation45].
Anticancer activity of IONPs
To detect cell proliferation, researchers utilized the MTT (3-(4,5-dimethylthiazol-2-yl)-2,5-diphenyltetrazolium bromide) assay. In this assay, the yellow colour of the tetrazolium salt transforms into purple formazan within living cells. The intensity of the purple colour increases with the presence of more living cells [Citation46].
illustrates the viability of cell lines treated with banana peel extract, IONPs, St-IONPs, DOX@St-IONPs and Free DOX resulting in viability rates of 67.8%, 31.7%, 25.1%, 5%, and 52% respectively, at a concentration of 1 µg/mL. These values indicate varying degrees of cytotoxicity against TNBC-MDA-mb 231 cell lines: 32.2%, 68.3%, 74.9%, 95.0%, and 48% respectively. Notably, DOX@St-IONPs exhibit significantly higher cytotoxicity (95.0%) compared to free DOX (48%). The incorporation of starch coating and DOX loading enhances the cytotoxic impact of IONPs on TNBC-MDA- mb 231 cells. Starch coating reduces the likelihood of IONPs aggregation, thereby improving their stability and potentially influencing cell viability upon internalization. Moreover, the synergistic cytotoxic effect of DOX with St-IONPs on TNBC-MDA-mb 231 is evident, as demonstrated previously using DOX-loaded carboxymethyl Assam bora rice starch-coated superparamagnetic iron oxide nanoparticles against MCF-7 and HeLa cell lines [Citation44].
Figure 3. %cancer cell viability using different treatments including plant extract, IONPs, St-IONPs, DOX@St-IONPs, DOX and FeCl3.

Similarly, the banana peel extract displayed the highest IC50 values (2.27 µg/mL) among all treatments, indicating its lower potency against TNBC-MDA-mb 231 cell lines. In contrast, IONPs exhibited an IC50 of 1.11 µg/mL, demonstrating approximately twice the potency of the banana peel extract. Moreover, St-IONPs demonstrated a lower IC50 (0.55 µg/mL) compared to IONPs (1.11 µg/mL), signifying higher potency. Notably, DOX@St-IONPs exhibited the highest cytotoxicity with an IC50 of 0.26 µg/mL. St-IONPs exhibited a synergistic cytotoxic effect with DOX compared to free DOX, which had an IC50 of 4.58 µg/mL. This enhanced activity may be attributed to the slow release of the entrapped drug from the IONPs, bypassing the drug efflux in the interior of the cells, and the intrinsic cytotoxic activity of IONPs [Citation47].
Preparation of transdermal patches
The backing layer
The backing layer of EVA was flexible and transparent, with a uniform smooth surface. EVA copolymer was used to regulate the transdermal drug delivery system by preventing the loss of medication at the desired location and reducing drug exposure to other tissues by inhibiting bidirectional flow [Citation48]. The backing layer was prepared 24 hours before preparing the medicated gelling layer.
The medicated gelling layer
The PVA and CP dispersions were selected as adhesive gelling polymers [Citation49]. The prepared dispersions of CP and PVA were clear, transparent, and homogenous. The prepared patches (M1-M4) using those polymers were flexible and well adhered to the backing layer. M1 and M2 patches were brown to black, and uniform with homogenous distribution, whereas M3 and M4 patches were clear, with a uniform distribution of orange colour.
Characterization of the patches
shows the properties of the prepared patches. The thickness and weights of the patches were uniform. Drug content ranged between 97.2% and 97.8%. All patches were flexible; folded more than 300 times without any observation of any cracks or breaks. The surface pH of the patches (7.10-to 7.42) was assumed to be compatible with the skin pH [46]. Additionally, all patches showed an increase in weight with time upon contact with PB (pH 7.4). The percentage of swelling ranged between 56% and 68%. The patches with higher CP content (M1 and M4) showed the highest swelling percentage.
Table 2. Physicochemical properties of M1-M4 patches.
In vitro drug diffusion
illustrates the cumulative diffusion of DOX per unit surface area (1.77 cm2) over 24 hours. The M2 patch exhibited the highest cumulative DOX diffusion at 124.31 µg/cm2, while the lowest was observed with the M4 patch at 29.62 µg/cm2. The utilization of DOX@St-IONPs in M1 and M2 patches, featuring a nano-size range of 10–100 nm, enhanced DOX penetration compared to M3 and M4 patches loaded with free DOX. Furthermore, the diffusion of DOX across the cellulose membrane was greater with the M2 patch than with the M1 patch, indicating the influence of CP ratio on drug release rate. Increasing CP ratio led to decreased drug diffusion, attributed to CP’s ability to form a structured network, reducing drug permeability across the membrane [Citation50]. This aligns with swelling results, showing higher swelling percentage for M1 (65%) than M2 (58%). CP’s swelling property is well utilized in bio-adhesive topical formulations for controlled drug release [Citation51]. Additionally, Jss values, derived from cumulative amount per surface area versus time (), correlated with in vitro profiles, with M2 patch exhibiting the highest Jss (14.85 µg/cm2/h) and P (0.95 cm/h), while M3 patch had the lowest Jss (2.98 µg/cm2/h) and P (0.19 cm/h) values.
Figure 4. The cumulative amount of DOX diffused per unit surface area across cellulose membrane (surface area = 1.77 cm2) from different patches (M1-M4).

Table 3. The diffusion parameters (flux and permeability) of DOX across cellulose membrane.
M1 and M2 patches contained DOX@St-IONPs, resulting in significantly higher DOX diffusion compared to M3 and M4 patches. Conjugating DOX to metallic oxide nanoparticles with starch coating likely reduced NP volume and size, enhancing drug permeation. Additionally, CP and PVA combination formed a stable gel layer, controlling DOX diffusion while maintaining structure post-diffusion. outlines the proposed DOX release mechanism, suggesting CP forms a mesh network upon hydration to stabilize drug release gradually. PVA strengthens CP’s gel structure by increasing pH above CP’s pKa (4.28), enhancing carboxylic group ionization, and swelling for better drug release control. Moreover, DOX back diffusion across EVA was minimal after 24 hours (5.12–5.85%), ensuring unidirectional release and preventing drug loss at the target site.
Drawbacks of the patches
The proposed approach faces several drawbacks and limitations. Firstly, ensuring technical feasibility is crucial, particularly in achieving a lengthy circulation half-life with low systemic toxicity for effective clinical translation. Patient adherence to transdermal patches is essential, as non-compliance with application schedules may impact treatment outcomes. Moreover, there’s a risk of skin irritation or discomfort associated with these patches, potentially affecting patient compliance and tolerance. Maintaining optimal drug dosage control is challenging, especially concerning potential cytotoxic effects on healthy cells. Despite the promise of nanoparticle-based drug delivery systems, challenges persist in achieving effective drug penetration through the skin barrier, warranting further investigation. Lastly, rigorous evaluation through in vivo studies and regulatory scrutiny is indispensable for the successful clinical translation of such systems.
Conclusion
Non-invasive bilayer transdermal patches comprising PVA and CP 971 were successfully developed. These patches, loaded with greenly synthesized DOX@St-IONPs (particle size: 38.1 nm, PDI: 0.5, zeta potential: −12.3 mV), exhibited promising in vitro anticancer activity against TNBC-MDA-mb 231 (cell cytotoxicity: 95%, IC50: 0.26 µg/mL). This novel delivery approach holds potential as a remote cancer treatment strategy. The optimal particle size and DOX conjugation contributed to enhanced cytotoxic activity and efficient skin penetration. External magnetic field application could enable precise drug release, facilitating magnetic nanoparticle accumulation in specific tissues, thereby reducing required dosages and associated side effects. However, comprehensive in vivo studies and regulatory scrutiny are imperative for successful clinical translation. Further research is needed to ensure prolonged circulation half-life, minimal systemic toxicity, and address potential skin irritation and allergic reactions at the application site.
Disclosure statement
No potential conflict of interest was reported by the author(s).
Additional information
Funding
References
- Rivankar S. An overview of doxorubicin formulations in cancer therapy. J Can Res Ther. 2014;10(4):853–12. doi: 10.4103/0973-1482.139267
- Petrilli R, Lopez RFV. Physical methods for topical skin drug delivery: concepts and applications. Braz J Pharm Sci. (2018);54(Special Issue). doi: 10.1590/S2175-97902018000001008
- Raviraj V, Pham BTT, Kim BJ, et al. Non-invasive transdermal delivery of chemotherapeutic molecules in vivo using superparamagnetic iron oxide nanoparticles. Cancer Nanotechnol. (2021);12(1):6. doi: 10.1186/s12645-021-00079-7
- Ahmed KS, Shan X, Mao J, et al. Derma roller® microneedles-mediated transdermal delivery of doxorubicin and celecoxib co-loaded liposomes for enhancing the anticancer effect. Mater Sci Eng C. 2019;99:1448–1458. doi: 10.1016/j.msec.2019.02.095
- Jiang SP, He SN, Li YL, et al. Preparation and characteristics of lipid nanoemulsion formulations loaded with doxorubicin. Int J Nanomedicine. 2013;8:3141–3150. doi: 10.2147/IJN.S47708 Epub 2013 Aug 19. PMID: 23990722; PMCID: PMC3753155.
- Huang S, Liu H, Huang S, et al. Dextran methacrylate hydrogel microneedles loaded with doxorubicin and trametinib for continuous transdermal administration of melanoma. Carbohydr Polym. 2020;246:116650. doi: 10.1016/j.carbpol.2020.116650
- Liu J, Tagami T, Ozeki T. Fabrication of 3D-printed fish-gelatin-based polymer hydrogel patches for local delivery of pegylated liposomal doxorubicin. Mar Drugs. 2020;18(6):325. doi: 10.3390/md18060325
- Dadfar SM, Roemhild K, Drude NI, et al. Iron oxide nanoparticles: diagnostic, therapeutic and theranostic applications. Adv Drug Deliv Rev. 2019 Jan 1;138:302–325. doi: 10.1016/j.addr.2019.01.005 Epub 2019 Jan 11. PMID: 30639256; PMCID: PMC7115878.
- Mahmoud N, Hamed R, Khalil E. Colloidal stability and rheological properties of gold nanoparticle–loaded polymeric hydrogels: impact of nanoparticle’s shape, surface modification, and concentration. Colloid Polym Sci. 2020;298(8):989–999. doi: 10.1007/s00396-020-04659-8
- El-Maddawy ZK, Abd El Naby WSH. Protective effects of zinc oxide nanoparticles against doxorubicin induced testicular toxicity and DNA damage in male rats. Toxicol Res (Camb). 2019 Jun 15;8(5):654–662. doi: 10.1039/c9tx00052f PMID: 31588342; PMCID: PMC6762007.
- Abu-Huwaij R, Al-Assaf S, Mousli F, et al. Perceptive review on properties of iron oxide nanoparticles and their antimicrobial and anticancer activity. Sys Rev Pharm 2020;11(8):418–431
- Vo TMT, Mondal S, Nguyen VT, et al. Rice starch coated iron oxide nanoparticles: a theranostic probe for photoacoustic imaging-guided photothermal cancer therapy. Int j biol macromol. 2021a;183:55–67. doi: 10.1016/J.IJBIOMAC.2021.04.053
- Arias LS, Pessan JP, Vieira APM, et al. Iron oxide nanoparticles for biomedical applications: a perspective on synthesis, drugs, antimicrobial activity, and toxicity. Antibiotics. 2018 Jun 9;7(2):46. doi: 10.3390/antibiotics7020046 PMID: 29890753; PMCID: PMC6023022.
- Turrina C, Berensmeier S, Schwaminger SP. Bare iron oxide nanoparticles as drug delivery carrier for the short cationic peptide Lasioglossin. Pharmaceuticals (Basel). 2021 Apr 24;14(5):405. doi: 10.3390/ph14050405 PMID: 33923229; PMCID: PMC8146918.
- Somvanshi S, Kharat P, Saraf T, et al. Multifunctional nano-magnetic particles assisted viral RNA-extraction protocol for potential detection of COVID-19. Mater Res Innovations. 2021;25(3):169–174. doi: 10.1080/14328917.2020.1769350
- Vangijzegem T, Stanicki D, Laurent S. Magnetic iron oxide nanoparticles for drug delivery: applications and characteristics. Expert Opin Drug Delivery. 2019;16(1):69–78. doi: 10.1080/17425247.2019.1554647 Taylor and Francis Ltd.
- Phalake SS, Tofail S, Thorat N, et al. Functionalized manganese iron oxide nanoparticles: a dual potential magneto-chemotherapeutic cargo in a 3D breast cancer model. Nanoscale. (2023);15 (38):15686–15699. doi: 10.1039/D3NR02816J
- Al-Shalabi R, Abu-Huwaij R, Hamed R, et al. The antimicrobial and the antiproliferative effect of human triple negative breast cancer cells using the greenly synthesized iron oxide nanoparticles. J Drug Delivery Sci Technol. 2022;75. doi: 10.1016/j.jddst.2022.103642
- Salem SS, Fouda A. Green synthesis of metallic nanoparticles and their prospective biotechnological applications: an overview. Biol Trace Elem Res. 2021 Jan;199(1):344–370. doi: 10.1007/s12011-020-02138-3
- García SL, Raghavan V. Green extraction techniques from fruit and vegetable waste to obtain bioactive compounds-a review. Crit Rev Food Sci Nutr. 2022;62(23):6446–6466. doi: 10.1080/10408398.2021.1901651 Epub 2021 Apr 1. PMID: 33792417.
- Dauda AB, Ajadi A, Tola-Fabunmi AS, et al. Waste production in aquaculture: sources, components and managements in different culture systems. Aquacult Fish. 2019;4(3):81–88. doi: 10.1016/j.aaf.2018.10.002
- Joshi R, Sharma DC (2018). Banana peel: a valuable waste. J Pharmacogn Phytochem, 7(1):1790–1793.
- Mahmoud N, Aqabani H, Hikmat S, et al. Colloidal stability and cytotoxicity of polydopamine-conjugated gold nanorods against prostate cancer cell lines. Molecules. 2021;26(5):1299. doi: 10.3390/molecules26051299
- Bankar A, Joshi B, Kumar AR, et al. Banana peel extract mediated novel route for the synthesis of silver nanoparticles. Colloids Surf A Physicochem Eng Asp. (2010);368(1–3):58–63. doi: 10.1016/j.colsurfa.2010.07.024
- Majumder A, Ramrakhiani L, Mukherjee D, et al. Green synthesis of iron oxide nanoparticles for arsenic remediation in water and sludge utilization. Clean Technol Envir. (2019);21(4):795–813. doi: 10.1007/s10098-019-01669-1
- Tyagi PK, Gupta S, Tyagi S, et al. Green synthesis of iron nanoparticles from spinach leaf and banana peel aqueous extracts and evaluation of antibacterial potential. J Nanomater. 2021;2021:4871453. doi: 10.1155/2021/4871453
- Ehiowemwenguan G, Emoghene AO, Inetianbor JE. Antibacterial and phytochemical analysis of banana fruit peel. IOSR J Pharm. (2014);4(8):18–25. doi: 10.9790/3013-0408018025
- Zou Y, Li D, Wang Y, et al. Polyethylenimine nanogels incorporated with ultrasmall iron oxide nanoparticles and doxorubicin for MR imaging-guided chemotherapy of tumors. Bioconjugate Chem. 2020;31(3):907–915. doi: 10.1021/acs.bioconjchem.0c00036
- Abu-Rumman A, Abu-Huwaij R, Rania Hamed R. Development and in vitro appraisal of Soluplus® and/or Carbopol® 971 buccoadhesive patches releasing atorvastatin. J Adhes. 2022;98(7):915–933. doi: 10.1080/00218464.2020.1864337
- Abu-Huwaij R, Abbas MM, Al-Shalabi R, et al. Synthesis of transdermal patches loaded with greenly synthesized zinc oxide nanoparticles and their cytotoxic activity against triple negative breast cancer. Appl Nanosci. (2022);12(1):69–78. doi: 10.1007/s13204-021-02166-y
- Saif S, Tahir A, Chen Y. Green synthesis of iron nanoparticles and their environmental applications and implications. Nanomaterials. 2016;6(11):209. doi: 10.3390/nano6110209
- Goh HT, Cheok CY, Yeap SP. Green synthesis of silver nanoparticles using banana peel extract and application on banana preservation. Food Front. (2023);4(1):283–288. doi: 10.1002/fft2.206
- Abid MA, Kadhim DA, Aziz WJ (2020). Iron oxide nanoparticle synthesis using trigonella and tomato extracts and their antibacterial activity. Mater Technol, 37(8):547–554. doi: 10.1080/10667857.2020.1863572
- Wabler M, Zhu W, Hedayati M, et al. Magnetic resonance imaging contrast of iron oxide nanoparticles developed for hyperthermia is dominated by iron content. Int J Hyperthermia. (2014);30(3):192–200. doi: 10.3109/02656736.2014.913321
- Yu M, Ji N, Wang Y, et al. Starch-based nanoparticles: stimuli responsiveness, toxicity, and interactions with food components. Compr Rev Food Sci Food Saf. 2021 Jan;20(1):1075–1100. doi: 10.1111/1541-4337.12677
- Kim DK, Mikhaylova M, Wang FH, et al. Starch-coated superparamagnetic nanoparticles as MR contrast agents. Chem Mater. (2003);15(23):4343–4351. doi: 10.1021/cm031104m
- Suljagić M, Vulić P, Jeremić D, et al. The influence of the starch coating on the magnetic properties of nanosized cobalt ferrites obtained by different synthetic methods. Mater Res Bull. 2021;134:111117. doi: 10.1016/j.materresbull.2020.111117
- Al-Qubaisi M, Rasedee A, Flaifel MH, et al. Green synthesis of magnetite (Fe3O4) nanoparticles using seaweed (Kappaphycus alvarezii) extract. Nanoscale Res Lett. (2016);11(1):276. doi: 10.1186/s11671-016-1498-2
- Shahdeo D, Roberts A, Kesarwani V, et al. Polymeric biocompatible iron oxide nanoparticles labelled with peptides for imaging in ovarian cancer. Biosci Rep. 2022;42(2): BSR20212622. doi: 10.1042/BSR20212622
- Khalil T, Ovais M, Ullah I, et al. Artificial cells, Nanomedicine, and biotechnology sageretia thea (Osbeck.) modulated biosynthesis of NiO nanoparticles and their in vitro pharmacognostic, antioxidant and cytotoxic potential. Artific Cells Nanomed Biotechnol. (2017);46(4):838–852. doi: 10.1080/21691401.2017.1345928
- Kamsonlian S, Balomajumder C, Chand S (2012). A potential of biosorbent derived from banana peel for removal of as (III) from contaminated water. Int J Chem Sci Appl. 3(2):269–275
- Sankadiya S, Oswal N, Jain P, et al. Synthesis and characterization of Fe2O3 nanoparticles by simple precipitation method. AIP Conf Proc. 2016 April;1724(1):020064. AIP Publishing LLC.
- Dung TT, Danh TM, Hoa LTM, et al. Structural and magnetic properties of starch-coated magnetite nanoparticles. J Exp Nanosci. 2009;4(3):259–267. doi: 10.1080/17458080802570609
- Mohapatra S, Asfer M, Anwar M, et al. Doxorubicin loaded carboxymethyl Assam bora rice starch coated superparamagnetic iron oxide nanoparticles as potential antitumor cargo. Heliyon. 2019;5(6):e01955. doi: 10.1016/j.heliyon.2019.e01955
- Saikia C, Das MK, Ramteke A, et al. Effect of crosslinker on drug delivery properties of curcumin loaded starch coated iron oxide nanoparticles. Int j biol macromol. 2016;93:1121–1132.. doi: 10.1016/j.ijbiomac.2016.09.043
- Mahmoud N, Qabooq H, Alsotari S, et al. Quercetin-gold nanorods incorporated into nanofibers: development, optimization and cytotoxicity. RSC Adv. 2021 Jun 7;11(33): 19956–19966. doi: 10.1039/d1ra02004h PMID: 35479887; PMCID: PMC9033756.
- Lee H, Park S, Kim JB, et al. Entrapped doxorubicin nanoparticles for the treatment of metastatic anoikis-resistant cancer cells. Cancer Lett. 2013 May 10;332(1):110–9. doi: 10.1016/j.canlet.2013.01.021
- Chen Y, Pan R, Guo L, et al. Transdermal delivery of meloxicam via hydrogel and microemulsion-based gel: a comparative study. J Pharmaceut sci. (2019);108(6):2086–2093. doi: 10.1016/j.xphs.2019.01.010
- Chen X, Taguchi T. Enhanced skin adhesive property of hydrophobically modified poly(vinyl alcohol) films. ACS Omega. 2020;5(3):1519–1527. doi: 10.1021/acsomega.9b03305
- Patel MR, Patel RB, Parikh JR, et al. Novel isotretinoin microemulsion-based gel for targeted topical therapy of acne: formulation consideration, skin retention and skin irritation studies. Appl Nanosci (Switzerland). (2016);6(4):539–553. doi: 10.1007/s13204-015-0457-z
- Pagano C, Ceccarini MR, Calarco P, et al. Bioadhesive polymeric films based on using acid for burn wound treatment: antibacterial and cytotoxicity studies. Colloids Surf B Biointerfaces. 2019;178:488–499. doi: 10.1016/j.colsurfb.2019.03.001