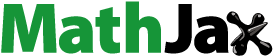
ABSTRACT
This study explores enhancing epoxy polymers with cerium aluminate (CeAlO3) nanoparticles via a solution combustion process. CeAlO3 loading (0.5 to 2.5 wt.%) significantly influences resulting composite properties. Characterization techniques confirm CeAlO3 presence, porous spherical morphology, and formation. FTIR spectroscopy identifies functional groups, while TGA shows excellent thermal stability below 200 °C, with minimal mass loss, and stability at 900 °C. The composites exhibit enhanced properties, with maximum tensile strength at 0.5 wt.%, a linear increase in tensile modulus up to 2.5 wt.%, and maximum compressive strength at 0.5 wt.%, with increasing modulus at higher filler loadings. These findings suggest CeAlO3-filled epoxy composites hold promise for high-temperature applications, emphasizing the importance of low filler concentrations for optimal structural properties. Overall, this research underscores the potential of CeAlO3 nanoparticles in advancing epoxy-based materials across various industries.
Introduction
The combustion synthesis method is widely employed for producing uniformly sized, finely dispersed crystalline oxides, metals, and alloys homogeneously [Citation1]. This method offers the advantage of bypassing the need for intermediate preparation steps, making it a quick, straightforward, and time-efficient process. It is particularly versatile, enabling the synthesis of various metal-based materials, including nitrides, perovskites, oxides, carbides, and alloys, which find applications across diverse fields [Citation2]. Among the different combustion processes, the solution combustion method stands out, especially when it comes to producing metal oxide nanoparticles. It offers the flexibility to tailor the qualities of oxides for a wide range of applications by adjusting the metal composition during synthesis. The solution combustion route is advantageous in generating homogeneous products with lower ignition temperatures.
The solution combustion method of nanoparticle synthesis is a chemical technique employed to produce nanoparticles, which are extremely small particles typically in the range of 1 to 100 nanometers. This approach involves a rapid and highly exothermic reaction between metal salts or metal-containing compounds and a suitable fuel, often an organic compound. This reaction takes place within a liquid medium, typically a solvent, leading to the formation of suspended nanoparticles within the solution [Citation3,Citation4]. In the solution combustion process, organic molecules containing functional groups, such as glycine, serve as fuels, while metal nitrates act as oxidisers. When the fuel-metal nitrate mixture is heated, it undergoes an extremely exothermic combustion reaction, producing fine solid powder and significant quantities of gaseous byproducts. The shape and size of the resulting nanoparticles are often strongly influenced by the type and quantity of the fuel in the reaction mixture. In certain cases, the choice and amount of fuel can even induce phase changes and the formation of metastable phases [Citation4,Citation5]. Cerium Aluminate (CeAlO3) nanoparticles, a significant inorganic material, have widespread applications in catalysis, superconductors, and ceramics. They are used as catalysts and electrode-active materials for processes like carbon monoxide oxidation, hydrocarbon reactions, phenol degradation in supercritical water, and nitrous oxide decomposition with ammonia [Citation6–12].
The solution combustion method is a chemical process utilised to fabricate nanoparticles, which are minute particles typically measuring between 1 to 100 nanometers in size. This technique involves a rapid and highly exothermic reaction between metal salts or compounds containing metals and a suitable fuel, often an organic compound. This reaction occurs within a liquid medium, commonly a solvent, resulting in the formation of suspended nanoparticles within the solution. In the solution combustion process, organic molecules containing functional groups, like glycine, act as fuels, while metal nitrates serve as oxidisers. Upon heating the mixture of fuel and metal nitrate, an extremely exothermic combustion reaction ensues, yielding fine solid powder along with significant amounts of gaseous byproducts. The shape and size of the resultant nanoparticles are frequently influenced by the type and quantity of the fuel incorporated into the reaction mixture. In specific instances, the selection and proportion of the fuel can even trigger phase transitions and the formation of metastable phases. One notable application of nanoparticles synthesised through this method is in the production of cerium aluminate (CeAlO3) nanoparticles, which find extensive use in various fields such as catalysis, superconductors, and ceramics. These nanoparticles serve as catalysts and electrode-active materials in numerous processes including carbon monoxide oxidation, hydrocarbon reactions, phenol degradation in supercritical water, and nitrous oxide decomposition with ammonia [Citation13–19].
Various-sized fillers are frequently used to reinforce polymers, aiming to enhance their properties and overcome some of the limitations inherent to polymers, thereby broadening their application potential. Traditional polymer composites have given way to a novel approach employing nanoscale fillers to augment the mechanical, physical, and chemical attributes of polymers. Nanofillers can be classified into three main categories: two-dimensional (2D) layered materials such as silicate and graphene, one-dimensional (1D) materials like carbon nanofibers and nanotubes, and fibrous or zero-dimensional (0D) materials such as quantum dots and spherical silica [Citation20–24]. Nano-metal oxide polymer composites incorporate minute metal particles into a conventional polymer matrix. Incorporating metal oxide nanoparticles into polymers leads to significant improvements in properties, including increased toughness, enhanced mechanical strength, improved thermal conductivity, and elevated electrical conductivity. Typically, less than 5% by weight of metal oxide nanoparticles are incorporated into the polymer matrix due to their remarkable efficiency in influencing the properties of the polymer composites [Citation25–30].
Incorporating metal oxide nanoparticles into polymer composites imparts unique qualities to these materials, making them suitable for a wide range of applications. Notably, metal oxide nanoparticles like CeAlO3 exhibit distinctive optical properties, which are contingent on both their size and shape. This renders them promising candidates for applications such as heterogeneous catalysis, and more. The present work is focused on the synthesis of metal oxide-polymer nanocomposites using CeAlO3 nanoparticles. The present study aims to synthesise composite materials comprising nano-CeAlO3 and epoxy, varying the quantities of synthesised CeAlO3 nanoparticles. To assess these materials, various characterisation techniques were employed, including Scanning Electron Microscopy (SEM), Energy Dispersive X-ray (EDX), X-ray Diffraction (XRD), Fourier-Transform Infrared Spectroscopy (FTIR), and Thermogravimetric Analysis (TGA), Photoluminescence (PL), Mechanical properties utilised using Universal Testing Machine (UTM). Experiments were conducted to investigate the influence of CeAlO3 nanoparticle loading on polymer curing time, thermal properties, optical properties, and mechanical properties. These characteristics are crucial when using these nanocomposites as strengthening materials [Citation31,Citation32].
The even and consistent dispersion of nanoparticles throughout the epoxy matrix plays a crucial role in making a polymer composite distinct and improving its overall mechanical characteristics. Several studies have highlighted that incorporating low concentrations of nano-fillers, typically ranging from 0.5 wt.% to 2.5 wt.%, results in a substantial enhancement in mechanical properties. In specific investigations, CeAlO3 was employed as a nanofiller in epoxy, demonstrating its capability to enhance surface damage under multi-scratching conditions after viscoelastic recovery. Researchers noted that the addition of nanoparticles increased the stiffness of epoxy. The impact of CeAlO3 concentration on the mechanical properties of epoxy was thoroughly examined. Tensile properties, including ultimate tensile strength and tensile modulus, as well as compressive properties, such as compressive strength and compressive modulus, showed significant improvements. The introduction of CeAlO3 also contributed to enhanced crack resistance, particularly up to a 2.5wt% concentration in epoxy. However, a further increase in filler content resulted in a decline in mechanical properties. These findings underscore the delicate balance required in optimising the concentration of CeAlO3 for achieving the desired improvements in epoxy mechanical performance [Citation33–35].
Over the past decade, polymer nanocomposites have garnered considerable attention from both industry and academia. These materials represent a novel category of multiphase substances characterised by the dispersion of an ultrafine phase typically ranging from 1 to 100 nanometers. They offer an intriguing blend of inorganic-organic or organic-organic components, presenting not only promising technological applications but also serving as valuable systems for investigating fundamental scientific principles on a scale intermediate between nano- and microscale. Numerous experimental studies have revealed that polymer nanocomposites exhibit novel and often enhanced properties that surpass those of individual phases or conventional composite materials. These improvements encompass mechanical characteristics such as increased tensile strength and modulus, reduced thermal expansion coefficients, and enhanced solvent resistance. Moreover, the inclusion of nanoparticles generally augments the elastic modulus without compromising rheological behaviour or processing efficiency, while also influencing the optical properties of the polymer matrix. The distinctive conformation of polymers within the nanoparticle host galleries, coupled with specific polymer-surface interactions not typically observed in bulk materials, significantly alters local and global polymer dynamics. This confinement effect profoundly impacts chain dynamics and contributes to the observed synergistic enhancements in material properties. Despite these advancements, the underlying mechanisms driving these improvements remain incompletely understood. Current theories suggest that nanoparticle effects stem from optically confined polymer matrices, quantum size phenomena, and Coulombic charging effects arising from ultrafine sizes, morphologies, and interfacial interactions between phases [Citation36–47].
CeAlO3 nanoparticles and nano-CeAlO3 polymer composites are incredibly versatile materials with significant applications, especially in the aerospace sector. They possess distinct properties, including size-dependent optical and catalytic characteristics, which are highly valuable in modern technology. To fully harness their potential in aerospace applications, a comprehensive set of analytical techniques is required for their characterisation. This includes using SEM and EDX for examining surface morphology and elemental composition, XRD for studying crystal structures, FTIR for identifying functional groups, TGA for assessing thermal stability, PL for assessing Luminescence spectra, UTM for assessing mechanical properties, and this characterisation process enables tailoring these materials to meet the specific demands of the aerospace industry. In aerospace applications, these materials excel under extreme conditions and seamlessly integrate into critical components such as engine parts, heat shields, and structural elements. They contribute to enhanced safety and durability while also serving as effective reinforcing agents for polymers, thus improving the thermal properties of materials used in aircraft construction. This results in reduced weight, improved fuel efficiency, and enhanced overall performance of aerospace systems [Citation48]. The incorporation of CeAlO3 nanoparticles in the synthesis of CeAlO3 nanoparticles and nano-CeAlO3 epoxy composite materials is aimed at enhancing the composite’s properties and expanding its applications across various fields [Citation49]. The integration of nanofillers into polymer matrices represents a promising strategy to enhance resistance against chemical degradation and environmental challenges. This advancement is particularly valuable for applications exposed to harsh chemicals and extreme conditions, commonly encountered in industrial and outdoor settings. This approach holds substantial potential for addressing durability concerns in demanding operational environments [Citation50].
In terms of applications, polymer composites incorporating CeAlO3 and similar nano-fillers are being employed in the aerospace industry for the manufacturing of components such as aircraft panels, wings, and structural elements [Citation51]. These advanced materials contribute to the development of lightweight yet robust structures, improving fuel efficiency and overall aircraft performance. The enhanced polymer composites designed for food packaging find diverse applications. The improved mechanical properties contribute to the packaging’s increased robustness, offering superior protection for the contents within. Furthermore, the heightened resistance to cracks observed in these composites proves particularly valuable in averting the formation of fractures that may endanger the packaging’s reliability, thereby ensuring the safety and quality of the packaged food [Citation52,Citation53].
Materials and methods
Materials
The precursor salt of Cerium nitrate hexahydrate (Ce(NO3)3. 6H2O, ≥ 90 %), Aluminium nitrate nonahydrate (Al(NO3)3 · 9H2O ≥ 90 %) and Glycine (CH2NH2COOH) were obtained from Sigma-Aldrich. The Araldite epoxy resin XIN-100 IN and Araldite hardener XIN-900 IN were used as received. Metal precursor solutions were created using deionised water.
Synthesis of CeAlO3 nanoparticles
CeAlO3 nanoparticles were synthesised via the solution combustion method, where Glycine was employed as a combustion enhancer along with Cerium nitrate hexahydrate precursor salt. The fuel-to-nitrate ratio (F/N) was held at 0.3, requiring the dissolution of 4.34 g of Cerium Nitrate Hexahydrate, 7.5 g of Aluminium nitrate nonahydrate, and 1.8 grams of Glycine dissolved in 100 mL of deionised water. The reaction mixture was consistently agitated on a heated plate. As the heating process advanced, it resulted in the release of gases, including carbon dioxide, water vapour, and nitrogen dioxide (distinguished by its reddish-brown hue and potent odour), leading to the formation of a gel. Within a short period after gel formation, the reaction spontaneously reached completion through self-ignition combustion, producing a fine, porous powder. Subsequently, the ultimate product underwent a 3-hour calcination process at 600 °C in a muffle furnace.
Preparation of nano CeAlO3 polymer-epoxy composite
A specified quantity of epoxy was measured and subjected to sonication for 45 minutes along with CeAlO3 using a 200 W ultrasonicator, which caused the epoxy’s temperature to rise to 60 °C. Different weight percentages (wt.%) of CeAlO3, specifically 0.5, 1.0, 1.5, 2.0, and 2.5 wt.%, were prepared and mixed during sonication. Following cooling to room temperature, a mixture of epoxy and hardener was prepared and cast into a mould. The mixture was left to dry at ambient temperature for 24 hours. To obtain Pristine epoxy, a combination of 90 % resin and 10 % hardener by volume was mixed. The same process was repeated to produce polymer composites infused with various proportions of CeAlO3. After the drying process, epoxy and nano-CeAlO3 polymer epoxy composites were obtained. The thickness of both the pristine epoxy and epoxy composites was measured using a digital vernier calliper, and it was determined that the average thickness for both was approximately 3 mm.
Material characterization techniques
The elemental composition and morphology analysis of CeAlO3 nanoparticles and nano-CeAlO3 polymer composites were carried out using a Hitachi S-3400 scanning electron microscope (SEM) coupled with energy-dispersive X-ray spectroscopy (EDX) and the INCA EDS detector. X-ray diffraction (XRD) measurements were conducted using an X-ray diffractometer with a tube voltage and current set at 40 kV and 40 mA. X-ray diffraction data collection was performed using a Bruker D8 ADVANCE from Germany. A Cu Kα (λ=1.5406 Å) source with a Ni filter was employed to generate X-rays. Data was collected over the 2θ range from 10 to 90 degrees, with a step size of 0.02 degrees. FTIR Spectral data was recorded as a percentage of transmittance, with a bandwidth of 5 nm, and were acquired using the ALPHA FT-IR instrument (Bruker) over a range of 4000 to 500 cm−1 in ECO-ATR transmittance mode. A total of 64 scans were performed during the FTIR analysis, with a resolution of 4 cm−1. Thermal properties were evaluated using a Thermal Analyzer-STA 449 F5 Jupiter, employing N2 gas as the sweeping gas within a SiC furnace. The mass loss data was collected over a temperature range of 30 to 900 ℃ for CeAlO3 nanoparticles, using a heating rate of 10 ℃/min, and maintaining gas flow rates of 20 mL/min for Gas Flow (protective) and 50 mL/min for Gas Flow (purge2). For the TGA experiments, an empty Al2O3 crucible was employed as a reference, while an Al2O3 crucible filled with the sample was used for the CeAlO3 nanoparticle analysis. The same experimental parameters were applied to the nano-CeAlO3 epoxy polymer composites, except for the heating temperature, which ranged from 30 to 600 ℃. The F2700 fluorescence spectrometer was used for examining the fluorescence properties of composites comprising CeAlO3 and Epoxy. The mechanical properties, including tensile and compressive strength, were evaluated utilising the Wance Universal Tensile Testing Machine (UTM). Tensile properties were assessed following the ASTM D638 standard testing procedure, with a crosshead speed of 10 mm-min-1. Compression properties were tested according to the ASTM D695 standard, with a testing speed of 2 mm-min−1. Three samples were taken and tested for each composite composition, and the reported values represent the averages.
Results and discussion
FE-SEM and EDX analysis
The CeAlO3 nanoparticles shown in predominantly display a spherical shape [Citation54]. illustrates the pure CeAlO3 nanoparticles, which are spherical, porous, and tend to aggregate. These CeAlO3 nanoparticles have sizes falling within the range of 44.57 to 74.66 nm. The agglomeration of particles is primarily attributed to the high surface energy and substantial surface area of the nanoparticles. At the nanoscale, large Van der Waals surface charges contribute to this agglomeration phenomenon [Citation55–59]. In , SEM images depict (a) pristine epoxy and epoxy- CeAlO3 polymer composites with varying CeAlO3 contents of (b) 0.5 wt.%, (c) 1.0 wt.%, (d) 1.5 wt.%, (e) 2.0 wt.%, and (f) 2.5 wt.%.
Figure 1. Scanning electron microscopy (SEM) images depicting cerium aluminate nanoparticles synthesized through the solution combustion method.

Figure 2. The scanning electron microscopy (SEM) images of (a) the pristine epoxy and epoxy- CeAlO3 polymer composites with different CeAlO3 content, specifically (b) 0.5 wt.%, (c) 1.0 wt.%, (d) 1.5 wt.%, (e) 2.0 wt.%, and (f) 2.5 wt.%.

reveals that at lower concentrations, the nanoparticles are evenly distributed within the epoxy matrix. However, as the nanofiller content increases, agglomeration becomes more evident, with larger clusters forming in some of the images. The extent of aggregation of CeAlO3 nanoparticles rises with higher filler content. Achieving a uniform dispersion of nanofillers within an epoxy polymer poses a significant challenge in the development of polymer composites [Citation60–62]. Furthermore, this increased agglomeration subsequently affects the thermal characteristics of the polymer composite.
In , the EDX spectrum displays seven prominent peaks, approximately at 0.3 keV, corresponding to the O element, the peaks at 1.6 KeV attributed to the Al element, and peaks at 0.85, 4.9, 5.1, 5.2, and 5.4 keV, attributed to the Ce element. Furthermore, EDX analysis was utilised to investigate potential impurities and provide information about the elemental composition of the synthesised CeAlO3 nanoparticles, as illustrated in . The detailed elemental composition of CeAlO3 can be found in .
Table 1. The elemental composition of CeAlO3 nanoparticles that was measured using energy dispersive X-ray (EDX).
XRD analysis of CeAlO3 and epoxy-CeAlO3 composites
The X-ray diffraction (XRD) patterns of the CeAlO3 nanoparticles, depicted in , display distinct peaks. These patterns prominently feature specific angles at 2θ values of approximately 16.9°, 28.9°, 33.3°, 47.8°, 56.65°, 70.0°, and 77.3°. Each of these angles corresponds to distinct crystal planes within the CeAlO3 crystal structure, namely the (100), (111), (110), (111), (200), (210), and (211) planes, respectively. The sample of CeAlO3 displayed a high degree of crystallinity, closely matching the CeAlO3 crystal structure as per the JCPDS file JCPDS 48–1548. The Scherrer equation, considering the Full Width at Half Maximum (FWHM) of peaks, X-ray wavelength, and diffraction angles, was employed to determine the crystallite size of CeAlO3. This approach involved analysing X-ray diffraction (XRD) patterns and their correlation with the atomic arrangement within the crystals. Wider peaks suggest a greater degree of size variation [Citation63,Citation64].
Figure 4. The XRD pattern of cerium aluminate nanoparticles synthesized using the solution combustion method with a lattice plane for each peak.

The average size of crystallites (D) was calculated using the Debye–Scherrer formula [Citation64], as represented in EquationEquation (1)(1)
(1) .
In the analysis, λ signifies the wavelength of the X-ray source (0.15418 nm), specifically corresponding to Cu K-alpha radiation. The parameter β denotes the Full Width at Half Maximum (FWHM), and θ represents the diffraction angle in the determination of crystallite size using the Debye–Scherrer formula. The computed mean crystallite size was established at 53.32 nm [Citation65]. This measurement falls within the range of particle sizes obtained from the SEM data.
In the composite polymer with nano-CeAlO3, you can find aggregated clusters that reach sizes of up to 500 nm. With an increase in the concentration of CeAlO3 in the nano-CeAlO3 polymer composite from 0.5% to 2.5% by weight, there is a corresponding elevation in saturation levels, leading to a reduction in the distance between CeAlO3 molecules among the particles. The XRD patterns of the polymer composite, containing CeAlO3 nanoparticles ranging from 0.5 to 2.5 wt.%, reveal prominent and strong peaks, reflecting the extent of CeAlO3 integration into the epoxy matrix, as depicted in . The strength of the peaks in the polymer composites is influenced by the amount of CeAlO3 loading. The augmentation of CeAlO3 loading from 0.5% to 2.5 wt.% leads to an increase in peak intensity, signifying enhanced crystallinity and a greater presence of CeAlO3 in the polymer composites. However, the peak position remains consistent even with increasing CeAlO3 loading. illustrates that the XRD analysis reveals the slightly amorphous nature of epoxy, with a crystallinity level of 90%. In , the XRD patterns of the nano-CeAlO3 polymer composites, containing varying amounts of CeAlO3 from 0.5 to 2.5 wt.%, demonstrate the crystalline nature of epoxy. This is apparent from the peak observed around 2θ = 28.9°, corresponding to the epoxy (111) crystal plane. Additionally, a few peaks around 18.1 and 28.7° become more prominent as the amount of CeAlO3 nanoparticles incorporated into the composite increases [Citation66–70].
Fourier-transform infrared spectroscopy (FTIR)
The FTIR spectra depicted in and exhibit distinctive peaks for CeAlO3 within the range of 4000 to 1300 cm−1, corresponding to the stretching vibrations of CeAlO3. The peak at 3700 cm−1 in the spectrum is associated with O-H stretching vibrations, indicating the presence of water molecules adsorbed on the surface of CeAlO3. The peaks detected at 3773 cm−1 in the FTIR spectrum indicate the presence of surface hydroxyl groups. The range of 3000–1200 cm−1 exhibits peaks associated with C-H groups (as summarised in ). The FTIR spectrum revealed peaks at approximately 1217 and 1068 cm−1, implying the presence of C-O groups. This observation aligns with previous research [Citation71–75]. The intensity of the CeAlO3 stretching vibration peak is observed to increase with the rise in the weight percentage of CeAlO3 in the polymer composites, thereby suggesting a direct correlation between peak intensity and the quantity of CeAlO3 in the composite. The peak within the range of 1600–1500 cm−1 is an indicator of the presence of a C=C group. within the FTIR spectra reveals peaks at approximately 1300 cm−1 and 800 cm−1, which can be associated with C-O stretching vibrations. Additionally, a peak at approximately 1236 cm−1 points to the existence of a C-N group. The Peaks within the 800–500 cm−1 range denote the presence of C-H groups, contributing to a more comprehensive view of the material’s structural features. The peaks at 2937 and 2918 cm−1 are indicative of the presence of C-H groups, and their intensity exhibits an upward trend with the increasing weight of CeAlO3 nanoparticles in the polymer composites [Citation67,Citation76,Citation77].
Figure 6. The FTIR spectrum of CeAlO3 nanoparticles illustrates vibrational modes or functional groups.

Figure 7. The FTIR spectra encompass (a) pristine epoxy and nano-CeAlO3 polymer composites with varying loads of CeAlO3 nanoparticles at (b) 0.5 wt.%, (c) 1.0 wt.%, (d) 1.5 wt.%, (e) 2.0 wt.%, and (f) 2.5 wt.%.

Table 2. FTIR peaks and respective functional groups.
Thermogravimetric analysis (TGA)
The CeAlO3 nanoparticles underwent Thermo Gravimetric Analysis (TGA) across a temperature range spanning from 30 to 900 °C. According to the data presented in , it was observed that, at 900 °C, the residual mass of the CeAlO3 nanoparticles remained at an impressive 94.88 %. During the decomposition process, merely 5.2 % of the initial mass was lost at this high temperature, and at the lower end of the spectrum (200 °C), there was only a minimal loss of mass. The outstanding thermal stability of the CeAlO3 nanoparticles positions them as excellent candidates for applications requiring exposure to high temperatures. This exceptional thermal stability is substantiated by the large residual mass that remains at 900 °C. The great surface area and crystallinity of CeAlO3 nanoparticles contribute significantly to their remarkable thermal stability and structural integrity. The crystalline nature of these nanoparticles also indicates that they can maintain their structural integrity even when subjected to elevated temperatures [Citation78–80]. The nano-CeAlO3 polymer composites underwent measurements to determine the transition temperature values at 250 °C. From , and the polymer composite samples displayed different levels of residual mass at 200 °C for various CeAlO3 loadings: 91% for 0–0.5 wt.%, 88% for 1.0 wt.%, 93% for 1.5 wt.%, 88% for 2.0 wt.%, and 95% for 2.5 wt.%. Across all CeAlO3 loadings, the residual mass varied from 88% to 95%, suggesting minimal substance breakdown at this temperature. The inclusion of CeAlO3 nanoparticles had a notable impact on the thermal stability of the epoxy matrix. The significant thermal stability exhibited by CeAlO3 nanoparticles, functioning as a thermal barrier, played a pivotal role in preventing the degradation of the epoxy matrix, as indicated by the substantial residual mass [Citation81].
Figure 8. Thermogravimetric analysis of CeAlO3 nanoparticles reveals the percentage weight loss relative to temperature.

Figure 9. Thermogravimetric analysis of CeAlO3 nanoparticles embedded in an epoxy polymer composite illustrates the percentage mass loss with respect to temperature.

Table 3. The remaining mass of CeAlO3 nanoparticles and epoxy polymer composites were impregnated with CeAlO3 at 250 °C.
Photoluminescence (PL)
Photoluminescence spectroscopy was employed to investigate the light emission properties of CeAlO3 when exposed to specific wavelengths. Electrons in CeAlO3 samples can be excited from their ground state to higher energy levels upon light exposure, resulting in a broad band of photoluminescence centred between 240 and 420 nm, corresponding to red light emission. This red emission is attributed to the release of photons during the recombination of excited electrons with holes at oxygen vacancies. Various factors, such as excitation wavelength, temperature, and the presence of defects or impurities, can influence the intensity and structure of the photoluminescence spectrum. Analysis of CeAlO3 polymer composite spectra revealed multiple peaks between 400 and 800 nm shown in . Peak shifting, where the peak location moves towards longer wavelengths as the excitation wavelength increases, was observed, indicating the re-absorption of photons by nanoparticles, causing a redshift in the photoluminescence peak [Citation82].
The confirmation that light recombination results in a hole with a single ionised electron in the valence band is supported by the intensity of a single ionised oxygen vacancy, leading to the red emission peak at 600 nm. Energy transfer between CeAlO3 nanoparticles and the polymer composite explains this phenomenon [Citation83]. The study suggests the potential for designing red-emitting optoelectronic devices using CeAlO3 nanoparticles loaded polymer composites. The increase in intensity varies with the amount of CeAlO3 loading intensity into the epoxy polymer. shows the increase in the conducting properties of the composite. The integration of CeAlO3 nanoparticles into epoxy polymer not only increases intensity but also improves the conducting property of the composite upon irradiation. Consequently, these composites find applications in electronics, providing better thermal qualities for reinforcement. Furthermore, polymer nanocomposites, incorporating CeAlO3 nanoparticles, demonstrate enhanced barrier properties, making them suitable for protective applications such as food packaging, thereby expanding their capabilities for safeguarding various products.
Mechanical properties
The Mechanical properties of a polymer matrix composite were examined using a universal testing machine (UTM). Tensile and compressive strength values were recorded and tabulated in and , respectively. The results are represented in . The primary focus of the study was to assess the impact of CeAlO3 filler concentration on the mechanical properties of the polymer matrix, particularly exploring tensile strength variations within the filler loading range of 0 to 2.5% weight. defines the influence of CeAlO3 loading on tensile strength within epoxy-CeAlO3 nanocomposites. reveals a good enhancement in tensile strength values with the addition of nanofillers. Notably, a noticeable increase of 30% was observed for nanocomposites containing 0.5 wt.% CeAlO3 compared to the pristine epoxy matrix. This can be attributed to the establishment of favourable interfacial tension between CeAlO3 and the epoxy matrix at the specified CeAlO3 concentration [Citation84].
Figure 12. The tensile strength of nanocomposites consists of epoxy and CeAlO3 at various concentrations.

Figure 13. The tensile modulus of nanocomposites consists of epoxy and CeAlO3 at various concentrations.

Figure 14. The compressive strength of nanocomposites consisting of epoxy and CeAlO3 at various concentrations.

Figure 15. The compressive modulus of nanocomposites consisting of epoxy and CeAlO3 at various concentrations.

Table 4. Tensile properties of nanocomposites synthesised.
Table 5. Compressive properties of nanocomposites synthesised.
A subsequent increase in the filler loading beyond 0.5 wt.% resulted in a decreasing trend in tensile strength. This phenomenon can be elucidated by the adverse effects of nanofiller agglomeration, wherein an excessive concentration led to a suboptimal dispersion within the polymer matrix. The resultant agglomeration detrimentally impacted the mechanical properties, notably causing a decrease in tensile strength. This investigation highlights the relationship between filler concentration, dispersion, and ensuing mechanical properties in polymer matrix composites. The findings affirm the importance of optimising filler content to utilise the beneficial effects on material strength while avoiding the adverse outcomes linked to the clumping together of particles [Citation78,Citation85,Citation86].
The interaction between the polymer matrix and nanofiller is more potent than that of the pure polymer, demonstrating increased strength compared to the pristine polymer.
depicts the variation in tensile modulus within epoxy-CeAlO3 nanocomposites. The tensile modulus increased linearly with the rise in filler concentration, as evidenced by both and in . The epoxy reached its highest strength in epoxy-2.5wt.% CeAlO3 composite, signifying the efficacy of incorporating nanoparticles into the epoxy matrix, resulting in heightened stiffness of the composite. This observation underscores the effectiveness of the polymer matrix-nanofiller interface, displaying a greater intensity compared to the original polymer. Examining the impact of filler loading on the compressive strength of the epoxy polymer is tabulated in and showing the improved trend. The epoxy-0.5wt.% CeAlO3 nanocomposite exhibited superior compressive strength when compared to the pristine epoxy, demonstrating an enhancement of approximately 10%. However, as the nanofiller loading increased, a linear reduction in compressive strength was observed. This decrease is associated with the possible liberation of free radicals during the homo-polymerisation process when epoxy and nanofiller load, resulting in reduced interactions between the epoxy matrix and nanofiller. The subsequent linear decrease in compressive strength beyond 0.5wt.% CeAlO3 can be attributed to the agglomeration of CeAlO3 nanoparticles [Citation87–89].
depicts changes in compressive modulus within epoxy-CeAlO3 nanocomposites. Higher filler loading in the epoxy-CeAlO3 nanocomposite results in a notably greater compressive modulus compared to the original epoxy. Specifically, the epoxy-2.5wt.% CeAlO3 nanocomposite demonstrated a substantial enhancement of approximately 45% in compressive modulus. This increase in compressive modulus was consistently observed with an increased loading of CeAlO3 [Citation90].
The future scope of this research involves a more detailed exploration of the CeAlO3 nanoparticle content, seeking an optimal balance between increased material properties and economical efficiency. Additionally, delving into the compatibility of these composites with diverse polymer matrices and analysing their performance across varied environmental conditions could unveil novel applications. Further investigation into the fundamental mechanisms underlying the observed peak shifting and photoluminescence behaviour could contribute to tailoring luminescent materials with precision. Addressing scalability and manufacturability considerations is paramount for the practical integration of these findings into industrial applications [Citation91].
The SEM analysis provided insights into the homogeneous distribution of CeAlO3 nanoparticles within the epoxy, manifesting as well-defined spherical particles. This uniform dispersion is pivotal for enhancing mechanical properties, rendering these composites well-suited for aerospace and automotive industries where lightweight yet robust materials are imperative. TGA results underscored minimal mass loss below 250 °C for nano-CeAlO3 polymer composites, signifying exceptional thermal stability. This thermal toughness positions these composites favourably for high-temperature applications, such as aerospace components or the construction of materials resistant to elevated temperatures [Citation92]. The study suggests that adding CeAlO3 nanoparticles to epoxy makes it better in certain ways, and this improvement depends on how much CeAlO3 is added. The experimental outcomes showcase that the good binding of CeAlO3 nanoparticles into epoxy polymer composites can yield materials endowed with a diverse spectrum of applications [Citation93]. These range from the development of high-strength aerospace components to the formulation of thermally stable adhesives.
The application of CeAlO3 as a nanofiller in epoxy matrices has shown promise in enhancing the stiffness of polymers used in aerospace components. This increased stiffness is particularly beneficial for structural elements that require strength and durability while remaining lightweight. Studies have also investigated the impact of CeAlO3 on crack resistance and overall mechanical performance. Improved crack resistance is crucial in aerospace applications where structural integrity is paramount, and materials must withstand varying stresses, including vibrations, impacts, and extreme temperature fluctuations [Citation94].
The use of CeAlO3 as a nanofiller in epoxy for food packaging purposes has shown promising results. Studies indicate that the inclusion of CeAlO3 enhances the surface durability of the polymer under various conditions, including multiscratching scenarios [Citation95]. This improved surface performance contributes to the overall integrity of the packaging material, ensuring it can withstand potential stresses during handling, transportation, and storage [Citation92,Citation96]. Moreover, investigations into the mechanical properties of polymer composites with CeAlO3 reveal positive outcomes. Tensile properties, such as ultimate tensile strength and tensile modulus, exhibit significant improvements, enhancing the material’s ability to withstand tensile forces. Compressive properties, including compressive strength and compressive modulus, also benefit from the incorporation of CeAlO3, contributing to the material’s capacity to withstand compression forces. The applications of such enhanced polymer composites in food packaging are diverse [Citation97].
Conclusion
In this research, CeAlO3 nanoparticles were synthesised using the solution combustion method and then incorporated into an epoxy matrix through ultrasonication to ensure even distribution. To enhance the properties of epoxy composites by reinforcing them with CeAlO3 nanoparticles. The epoxy matrix selected was Araldite epoxy resin XIN-100 IN and Araldite hardener XIN-900 IN. Then, a thorough analysis of the materials, focusing on their morphology, elemental composition, phase identification, and thermal characteristics was done. SEM images indicated that, at higher concentrations, CeAlO3 nanoparticles exhibited porosity but tended to aggregate. Specifically, samples containing 0.5 wt.% CeAlO3 displayed agglomerated spherical particles. EDX analysis validated the existence of Ce, Al, and O elements in the CeAlO3 nanoparticles. X-ray Diffraction (XRD) patterns validated the formation of the CeAlO3 phase, with distinct peaks at around 28.9° in samples impregnated with CeAlO3 nanofillers. Fourier Transform Infrared (FTIR) spectra displayed a unique peak at 2937 and 2918 cm−1, indicating OH stretching vibrations. In CeAlO3-impregnated epoxy polymer composites, the intensity of the CeAlO3 stretching vibration peak correlated directly with the CeAlO3 concentration, increasing from 0.5 to 2.5 wt.%. Thermogravimetric Analysis (TGA) revealed that pristine CeAlO3 showed minimal mass loss of 8.63 % at 200 °C. Adding 0.5 wt.% of CeAlO3 increased the onset temperature of degradation. CeAlO3-impregnated epoxy polymer composites (ranging from 0.5 to 2.5 wt.%) retained between 89–93 % of their mass at 200 °C, indicating improved thermal stability. However, a higher concentration of CeAlO3 led to a reduction in the onset temperature for degradation. This study highlights the potential of incorporating a small quantity of CeAlO3 nanoparticles to create advanced polymer composites with multiple scales. It provides the opportunity to combine nano- and micro-scale particles within fibre-reinforced polymer matrices, offering enhanced properties and capabilities for various applications. The luminescence spectra of both CeAlO3 nanoparticles and CeAlO3 polymer composites displayed peaks falling within the 400–800 nm range. A phenomenon known as peak shifting, where the peak location transitions towards longer wavelengths with an increase in excitation wavelength, was observed. The peak shift is due to nanoparticles re-absorbing photons, leading to a redshift in the photoluminescence peak. Mechanical testing revealed that the tensile strength reached its maximum at 0.5wt.% CeAlO3 filler loading. Tensile modulus increased with an increase in filler loading. Initially, compressive strength saw an increment, but it gradually declined as the filler concentration increased. On the other hand, the compressive modulus of the epoxy composites showed a linear increase with the concentration of nanoparticles in the matrix. The optimal combination for both tensile strength and compressive strength was observed in the epoxy-0.5 wt.% CeAlO3 composite improved over the original epoxy. The highest values for tensile modulus and compressive modulus were noted in a nanocomposite with epoxy-2.5wt.% CeAlO3 compared to other filler loading and pristine epoxy.
Acknowledgments
The authors acknowledge the Centre for Advanced Materials Technology of M. S. Ramaiah Institute of Technology, Bangalore, Karnataka, India for the necessary characterisation facilities used in this study. SEM-EDX characterisation was performed at BMS College of Engineering, Bangalore, Karnataka, India.
Disclosure statement
No potential conflict of interest was reported by the author(s).
References
- Venkatesan K, Rajan Babu D, Kavya Bai MP, et al. Structural and magnetic properties of cobalt-doped iron oxide nanoparticles prepared by solution combustion method for biomedical applications. Int J Nanomed. 2015;10(sup2): 189–17. doi: 10.2147/IJN.S82210
- Jani P, Desai H, Madhukar BS, et al. Investigations of calcium ferrite nanoparticles synthesized by sol-gel auto combustion and solution mixture methods. Mater Res Innovations. 2022;26(3):189–195. doi: 10.1080/14328917.2021.1932318
- La J, Huang Y, Luo G, et al. Synthesis of bismuth oxide nanoparticles by solution combustion method. Part Sci Technol. 2013;31(3):287–290. doi: 10.1080/02726351.2012.727525
- Ortiz-Quiñonez JL, Pal U, Villanueva MS. Structural, magnetic, and catalytic evaluation of spinel co, ni, and co–ni ferrite nanoparticles fabricated by low-temperature solution combustion process. ACS Omega. 2018;3(11):14986–15001. doi: 10.1021/acsomega.8b02229
- Panthong P, Klaytae T, Boonma K, et al. Preparation of SrTiO3 nanopowder via Sol-gel combustion method. Ferroelectrics. 2013;455(1):29–34. doi: 10.1080/00150193.2013.843412
- Liu Y, Zhang Y, Feng JD, et al. Dependence of magnetic properties on crystallite size of CoFe2O4 nanoparticles synthesised by auto-combustion method. J Exp Nanosci. 2009;4(2):159–168. doi: 10.1080/17458080902929895
- ) Hamisu A, Gaya UI, Abdullah AH. Bi-template assisted sol-gel synthesis of photocatalytically-active mesoporous anatase TiO2 nanoparticles. Appl Sci And Eng Prog. 2021;14(3):313–327. doi: 10.14416/j.asep.2021.04.003
- Rao KV, Sunandana CS. Structure and micro structure of combustion synthesized MgO nanoparticles and nanocrystalline MgO thin films synthesized by solution growth route. Synth ReactInorg Metal-Org Nano-Metal Chem. 2008;38(2):173–180.
- Devasena SM, Murali G, Amaranatha Reddy D, et al. Optical, magnetic, and photoluminescence properties of Cr/Mn-doped ZnO nanoparticles synthesised by solution combustion method. Mater Sci Technol. 2023;39(18):3076–3089. doi: 10.1080/02670836.2023.2239630
- Pramila S, Lakshmi Ranganatha V, Nagaraju G, et al. Microwave and combustion methods: a comparative study of synthesis, characterization, and applications of NiO nanoparticles. Inorg Nano-Metal Chem. 2023;53(6):527–538. doi: 10.1080/24701556.2022.2081188
- Rani R, Dhiman P, Sharma SK, et al. Structural and magnetic studies of Co0.6Zn0. 4Fe2O4 nanoferrite synthesized by solution combustion method. Synth ReactInorg Metal-Org Nano-Metal Chem. 2012;42(3):360–363. doi: 10.1080/15533174.2011.611062
- Chaiyo N, Ruangphanit A, Boonchom B, et al. Facile synthesis of lead-free piezoelectric sodium niobate (NaNbo3) powders via the solution combustion method. Ferroelectrics. 2011;415(1):75–82. doi: 10.1080/00150193.2011.577374
- Rana S, Rawat J, Sorensson MM, & Misra RDK. Antimicrobial function of Nd3±doped anatase titania-coated nickel ferrite composite nanoparticles: a biomaterial system. Acta Biomaterialia. 2006;2(4):421–432. doi: 10.1016/j.actbio.2006.03.005
- Sunkara BK, & Misra RDK. Enhanced antibactericidal function of W4±doped titania-coated nickel ferrite composite nanoparticles: a biomaterial system. Acta Biomaterialia. 2008;4(2):273–283. doi: 10.1016/j.actbio.2007.07.002
- Misra RDK. Magnetic nanoparticle carrier for targeted drug delivery: perspective, outlook and design. Mater Sci Technol. 2008;24(9):1011–1019. doi: 10.1179/174328408X341690
- Rawat J, Rana S, Srivastava R, & Misra RDK. Antimicrobial activity of composite nanoparticles consisting of titania photocatalytic shell and nickel ferrite magnetic core. Mater Sci Eng C. 2007; 27(3):540–545. doi: 10.1016/j.msec.2006.05.021
- Misra RDK, Gubbala S, Kale A, et al. A comparison of the magnetic characteristics of nanocrystalline nickel, zinc, and manganese ferrites synthesized by reverse micelle technique. Mater Sci Eng B. 2004;111(2–3):164–174. doi: 10.1016/j.mseb.2004.04.014
- Venkatasubramanian R, Srivastava RS, Misra RDK. Comparative study of antimicrobial and photocatalytic activity in titania encapsulated composite nanoparticles with different dopants. Mater Sci Technol. 2008;24(5):589–595. doi: 10.1179/174328408X282065
- Chandrasekaran S, Misra RDK. Photonic antioxidant ZnS (cd) nanorod synthesis for drug carrier and bioimaging. Mater Technol. 2013;28(4):228–233. doi: 10.1179/1753555713Y.0000000084
- Deeraj BDS, Joseph K, Jayan JS, & Saritha A. Dynamic mechanical performance of natural fiber reinforced composites: a brief review. Appl Sci And Eng Prog. 2021;14(4):614–623.
- Thakur VK, Thakur MK, Gupta RK. Review: raw natural fiber–based polymer composites. Int J Polym Anal Charact. 2014;19(3):256–271. doi: 10.1080/1023666X.2014.880016
- Kumar R, Ul Haq MI, Raina A, et al. Industrial applications of natural fibre-reinforced polymer composites–challenges and opportunities. Int J Sustainable Eng. 2019;12(3):212–220. doi: 10.1080/19397038.2018.1538267
- Thakur VK, Singha AS. Mechanical and water absorption properties of natural fibers/polymer biocomposites. Polym-Plast Technol Eng. 2010;49(7):694–700. doi: 10.1080/03602551003682067
- Jagadeesh P, Puttegowda M, Thyavihalli Girijappa YG, et al. Effect of natural filler materials on fiber reinforced hybrid polymer composites: an overview. J Nat Fibers. 2022;19(11):4132–4147. doi: 10.1080/15440478.2020.1854145
- Todkar SS, & Patil SA. Review on mechanical properties evaluation of pineapple leaf fibre (PALF) reinforced polymer composites. Composites. 2019;174:106927. doi: 10.1016/j.compositesb.2019.106927
- Vadivelu MA, Kumar CR, Joshi GM. Polymer composites for thermal management: a review. Compos Interfaces. 2016;23(9):847–872. doi: 10.1080/09276440.2016.1176853
- Sudeep PK, Emrick T. Polymer‐nanoparticle composites: preparative methods and electronically active materials. Polymer Rev. 2007;47(2):155–163. doi: 10.1080/15583720701271229
- Srikanth C, & Madhu GM (2017, December). Synthesis, characterization and properties evaluation of ZrO2 and its composites–A review. In International Conference on Advances in Thermal Systems, Materials and Design Engineering; Mumbai, India. (ATSMDE2017).
- Puttegowda M, Pulikkalparambil H, & Rangappa SM Trends and developments in natural fiber composites. Appl Sci And Eng Prog, 14(4):2021;543–552. doi: 10.14416/j.asep.2021.06.006
- Njuguna J, Pielichowski K. Polymer nanocomposites for aerospace applications: properties. Adv Eng Mater. 2003;5(11):769–778. doi: 10.1002/adem.200310101
- ) Setswalo K, Molaletsa N, Oladijo OP, Akinlabi ET, Rangappa SM, & Siengchin S. The influence of fiber processing and alkaline treatment on the properties of natural fiber-reinforced composites: a review. Appl Sci And Eng Prog.2021;14(4):632–650. doi: 10.14416/j.asep.2021.08.005
- Kausar A. Thermally conducting polymer/nanocarbon and polymer/inorganic nanoparticle nanocomposite: a review. Polym Plast Technol Eng. 2020;59(8):895–909. doi: 10.1080/25740881.2019.1708103
- Arbaoui J, Moustabchir H, Vigué JR, et al. The effects of various nanoparticles on the thermal and mechanical properties of an epoxy resin. Mater Res Innovations. 2016;20(2):145–150. doi: 10.1179/1433075X15Y.0000000026
- Jabbar M, Karahan M, Nawab Y, et al. Effect of silica nanoparticles on mechanical properties of Kevlar/epoxy hybrid composites. J Tex Inst. 2019;110(4):606–613. doi: 10.1080/00405000.2018.1529213
- Tee ZY, Yeap SP, Hassan CS, et al. Nano and non-nano fillers in enhancing mechanical properties of epoxy resins: a brief review. Polym Plast Technol Eng. 2022;61(7):709–725. doi: 10.1080/25740881.2021.2015778
- Yuan Q, Awate S, Misra RDK. Nonisothermal crystallization behavior of polypropylene–clay nanocomposites. Eur Polym J. 2006;42(9):1994–2003. doi: 10.1016/j.eurpolymj.2006.03.012
- Zhang JL, Srivastava RS, Misra RDK. Core− shell magnetite nanoparticles surface encapsulated with smart stimuli-responsive polymer: synthesis, characterization, and LCST of viable drug-targeting delivery system. Langmuir. 2007;23(11):6342–6351. doi: 10.1021/la0636199
- Yuan Q, Misra RDK. Polymer nanocomposites: current understanding and issues. Mater Sci Technol. 2006;22(7):742–755. doi: 10.1179/174328406X101292
- Zhang J, Misra RDK. Magnetic drug-targeting carrier encapsulated with thermosensitive smart polymer: core–shell nanoparticle carrier and drug release response. Acta Biomaterialia. 2007;3(6):838–850. doi: 10.1016/j.actbio.2007.05.011
- Misra RDK, Hadal R, Duncan SJ. Surface damage behavior during scratch deformation of mineral reinforced polymer composites. Acta Materialia. 2004;52(14):4363–4376. doi: 10.1016/j.actamat.2004.06.003
- Challa VSA, Misra RDK. Periodic crystallisation of polymers on carbon nanotubes: geometrical confinement versus epitaxy. Mater Technol. 2017;32(2):109–115. doi: 10.1080/10667857.2016.1143691
- Majidi B. Geopolymer technology, from fundamentals to advanced applications: a review. Mater Technol. 2009;24(2):79–87. doi: 10.1179/175355509X449355
- Ileperuma OA. Gel polymer electrolytes for dye sensitised solar cells: a review. Mater Technol. 2013;28(1–2):65–70. doi: 10.1179/1753555712Y.0000000043
- Singh R, Lee PD, Dashwood RJ, et al. Titanium foams for biomedical applications: a review. Mater Technol. 2010;25(3–4):127–136. doi: 10.1179/175355510X12744412709403
- Chaubey A, Aadil KR, Jha H. Synthesis and characterization of lignin-poly lactic acid film as active food packaging material. Mater Technol. 2021;36(10):585–593. doi: 10.1080/10667857.2020.1782060
- Jeong SY, Wagh AS. Cementing the gap between ceramics, cements, and polymers. Mater Technol. 2003;18(3):162–168. doi: 10.1080/10667857.2003.11753035
- Wang H, Sun J, Li J, et al. Roughness modelling analysis for milling of carbon fibre reinforced polymer composites. Mater Technol. 2015;30(sup1):A46–A50. doi: 10.1179/1753555715Y.0000000002
- Rafique I, Kausar A, Anwar Z, et al. Exploration of epoxy resins, hardening systems, and epoxy/carbon nanotube composite designed for high performance materials: a review. Polym-Plast Technol Eng. 2016;55(3):312–333. doi: 10.1080/03602559.2015.1070874
- Giovino M, Pribyl J, Benicewicz B, et al. Mechanical properties of polymer grafted nanoparticle composites. Nanocomposites. 2018;4(4):244–252. doi: 10.1080/20550324.2018.1560988
- Rathod VT, Kumar JS, Jain A. Polymer and ceramic nanocomposites for aerospace applications. Appl Nanosci. 2017;7(8):519–548. doi: 10.1007/s13204-017-0592-9
- Irzhak VI, Dzhardimalieva GI, Uflyand IE. Structure and properties of epoxy polymer nanocomposites reinforced with carbon nanotubes. J Polym Res. 2019;26(9):1–27. doi: 10.1007/s10965-019-1896-0
- Idumah CI, Zurina M, Ogbu J, Ndem JU, & Igba EC. A review on innovations in polymeric nanocomposite packaging materials and electrical sensors for food and agriculture. Compos Interfaces. 2019; 27 1 1–72 10.1080/09276440.2019.1600972
- Prasanth G, Madhu GM, Kottam N. Combustion assisted synthesis of CuO nanoparticles and structure-property evaluation in nano-CuO polymer composites. Appl Sci And Eng Prog. 2024 Apr-Jun;17(2): In progress. doi: 10.14416/j.asep.2023.11.009
- Kashyap SJ, Sankannavar R, Madhu GM. Insights on the various structural, optical and dielectric characteristics of La1-x ca x FeO3 perovskite-type oxides synthesized through solution-combustion technique. Appl Phys A. 2022;128(6):518. doi: 10.1007/s00339-022-05628-4
- Priyadharsini P, Pradeep A, Murugesan C, et al. Phase evolution in BiFeO3 nanoparticles prepared by glycine-assisted combustion method. Combust Sci Technol. 2014;186(3):297–312. doi: 10.1080/00102202.2013.859682
- Assi N, Mohammadi A, Manuchehri QS, et al. Synthesis and characterization of ZnO nanoparticle synthesized by a microwave-assisted combustion method and.
- ) Ma Y, Ma Y, Li J, Li Q, Hu X, Ye Z, … & Dong D. CeO2-promotion of NiAl2O4 reduction via CeAlO3 formation for efficient methane reforming. J Energy Inst. 2020; 93(3):991–999. doi: 10.1016/j.joei.2019.09.001
- Rao JK, Raizada A, Ganguly D, et al. Investigation of structural and electrical properties of novel CuO–PVA nanocomposite films. J Mater Sci. 2015;50(21):7064–7074. doi: 10.1007/s10853-015-9261-0
- Shahbakhsh S, Tohidlou E, Khosravi H. Influence of modified carbonate calcium nanoparticles on the mechanical properties of carbon fiber/epoxy composites. J Tex Inst. 2020;111(4):550–554. doi: 10.1080/00405000.2019.1651155
- Aigbodion VS. Explicit microstructure and electrical conductivity of epoxy/carbon nanotube and green silver nanoparticle enhanced hybrid dielectric composites. Nanocomposites. 2021;7(1):35–43. doi: 10.1080/20550324.2020.1868690
- Mallakpour S, Derakhshan F. Opportunities and challenges in the use of TiO2 nanoparticles modified with citric acid to synthesize advanced nanocomposites based on poly (amide-imide) containing N, N′-(pyromellitoyl)-bis-L-leucine segments. Int J Polym Anal Charact. 2014;19(8):750–764. doi: 10.1080/1023666X.2015.954771
- Srikanth C, and Madhu GM, “Effect of ZTA concentration on structural, thermal, mechanical and dielectric behavior of novel ZTA–PVA nanocomposite films,” SN Applied Sci, vol. 2, no. 3, 2020, doi:10.1007/s42452-020-2232-3.
- Aruna ST, Kini NS, Shetty S, et al. Synthesis of nanocrystalline CeAlO3 by solution-combustion route. Materials Chemistry & Physics. 2010;119(3):485–489. doi: 10.1016/j.matchemphys.2009.10.001
- Srikanth C, Madhu GM. Effect of nano CdO-ZnO content on structural, thermal, optical, mechanical and electrical properties of epoxy composites. J Met Mater Miner. 2023;33(2):38–52. doi: 10.55713/jmmm.v33i2.1590
- Venâncio SA, & de Miranda PEV. Synthesis of CeAlO3/CeO2–Al2O3 for use as a solid oxide fuel cell functional anode material. Ceram Int. 2011;37(8):3139–3152. doi: 10.1016/j.ceramint.2011.05.054
- Jena PK, Samal P, Nayak S, Behera JR, Khuntia SK, Mohapatra J, … & Malla C. Experimental investigation on the mechanical, thermal, and morphological behaviour of Prosopis juliflora bark reinforced epoxy polymer composite. J Nat Fibers.2022;19(14):8593–8603. 10.1080/15440478.2021.1964144
- Sharma P, Shah DV, Thakor S, Watpade AD, Rana VA, & Vaja CR. Compositional influence of synthesized magnetic nanoparticles on epoxy composites: dielectric, magnetic, and optical characteristics. J Macromol Sci, Part B. 2023;1–35. doi: 10.1080/00222348.2023.2263293
- Chen W, Zhao G, Xue Q, et al. High carbon-resistance Ni/CeAlO3-Al2O3 catalyst for CH4/CO2 reforming. Appl Catal B Environ. 2013;136:260–268. doi: 10.1016/j.apcatb.2013.01.044
- Wang J, Feng L, Feng Y, et al. Preparation and properties of organic rectorite/epoxy resin nano-composites. Polym-Plast Technol Eng. 2012;51(15):1583–1588. doi: 10.1080/03602559.2012.716894
- Thakur S, Bhattacharya R, Neogi S, et al. Enhancement of microwave absorption properties of epoxy by sol–gel-Synthesised ZnO nanoparticles. Indian Chem Eng. 2016;58(4):310–324. doi: 10.1080/00194506.2015.1090888
- Kashyap SJ, Sankannavar R, Madhu GM. Synthesis and characterization of La (ce, ba) NiO3 perovskite-type oxides. J Superconductivity Novel Magnetism. 2022;35(7):2107–2118. doi: 10.1007/s10948-022-06219-3
- Sagar JS, Madhu GM, Koteswararao J, & Dixit P. Studies on thermal and mechanical behavior of nano TiO2-epoxy polymer composite. Commun Sci Technol. 2022;7(1):38–44. doi: 10.21924/cst.7.1.2022.667
- ) Kashyap SJ, Sankannavar R, & Madhu GM. Hydroxyapatite nanoparticles synthesized with a wide range of Ca/P molar ratios and their structural, optical, and dielectric characterization. J Korean Ceram Soc. 2022;59(6):846–858. doi: 10.1007/s43207-022-00225-w
- Kamonsuangkasem K, Therdthianwong S, Therdthianwong A, & Thammajak N. Remarkable activity and stability of Ni catalyst supported on CeO2-Al2O3 via CeAlO3 perovskite towards glycerol steam reforming for hydrogen production. Appl Catal B Environ. 2017;218:650–663. doi: 10.1016/j.apcatb.2017.06.073
- Kurajica S, Mandić V, Mužina K, Panžić I, Kralj D, Duplančić M, & Ivković IK. Thermal stability and properties of Pd/CeAlO3 catalyst prepared by combustion synthesis. J Therm Anal Calorim. 2023;1–10:148 20 doi: 10.1007/s10973-023-12233-x
- Kausar A. An investigation on epoxy/poly (urethane-amide)-based interpenetrating polymer network reinforced with an organic nanoparticle. Mater Res Innovations. 2018;22(2):58–68. doi: 10.1080/14328917.2016.1265232
- Wang Y, Yao D, He Z, et al. Enhanced mechanical and damping properties of epoxy using aggregated nanoparticles organic-inorganic hybrid as a filler. Compos Interfaces. 2022;29(5):523–536. doi: 10.1080/09276440.2021.1982334
- Mishra R, Tiwari R, Marsalkova M, et al. Effect of TiO2 nanoparticles on basalt/polysiloxane composites: mechanical and thermal characterization. J Tex Inst. 2012;103(12):1361–1368. doi: 10.1080/00405000.2012.685270
- Nazari A, Riahi S. RETRACTED ARTICLE: the effects of SnO2 nanoparticles on physical and mechanical properties of high-strength self-compacting concrete. J Exp Nanosci. 2012;7(5):559–577. doi: 10.1080/17458080.2010.543991
- Nazari A, Riahi S. RETRACTED ARTICLE: the effects of ZrO2 nanoparticles on strength assessments and water permeability of concrete in different curing media. J Exp Nanosci. 2013;8(4):413–433. doi: 10.1080/17458080.2011.586369
- Srikanth C, Madhu GM, & Kashyap SJ. Enhanced structural, thermal, mechanical and electrical properties of nano ZTA/epoxy composites. AIMS Mat Sci. 2022;9(2):214–235 doi: 10.3934/matersci.2022013
- Kottam N, & Smrithi SP. Luminescent carbon nanodots: current prospects on synthesis, properties and sensing applications. Methods Appl Fluoresc. 2021;9(1):012001. 10.1088/2050-6120/abc008
- Sailaja Prasannakumaran Nair S, Kottam N, & SG PK. Green synthesized luminescent carbon nanodots for the sensing application of Fe3+ ions. J Fluoresc. 2020;30(2):357–363. doi: 10.1007/s10895-020-02505-2
- Megahed M, Megahed AA, Agwa MA. Mechanical properties of on/off-axis loading for hybrid glass fiber reinforced epoxy filled with silica and carbon black nanoparticles. Mater Technol. 2018;33(6):398–405. doi: 10.1080/10667857.2018.1454022
- Marjanović M, Bajić D, Perković S, et al. Inorganic fullerene-like nanoparticles and nanotubes of tungsten disulfide as reinforcement of carbon-epoxy composites. Fuller Nanotub Car Nanostruct. 2021;29(12):1034–1044. doi: 10.1080/1536383X.2021.1928644
- Halder S, Ghosh PK, Goyat MS, et al. Ultrasonic dual mode mixing and its effect on tensile properties of SiO2-epoxy nanocomposite. J Adhes Sci Technol. 2013;27(2):111–124. doi: 10.1080/01694243.2012.701510
- Chakradhar KVP, Subbaiah KV, Kumar MA, et al. Blended epoxy/polyester polymer nanocomposites: effect of “nano” on mechanical properties. Polym-Plast Technol Eng. 2012;51(1):92–96. doi: 10.1080/03602559.2011.618157
- Hiremath A, Ambekar AM, Thipperudrappa S, et al. Understanding the interfacial interaction of TiO2 nanoparticles filled glass fiber/epoxy composites through dynamic mechanical analysis. Compos Interfaces. 2023;30(7):787–802. doi: 10.1080/09276440.2023.2176058
- Kitichatpayak D, Makcharoen W, Vittayakorn N, et al. Influence of various nanofillers on mechanical and electrical properties of epoxy resin composites. Polym Plast Technol Eng. 2022;61(16):1826–1832. doi: 10.1080/25740881.2022.2084412
- Nayak SK, Mohanty D. Silver nanoparticles decorated α-alumina as a hybrid filler to fabricate epoxy-based thermal conductive hybrid composite for electronics packaging application. J Adhes Sci Technol. 2020;34(14):1507–1525. doi: 10.1080/01694243.2020.1714138
- Pillai KV, Hunt PR, & Duncan TV. Nanoparticles in polymer nanocomposite food contact materials: uses, potential release, and emerging toxicological concerns. Toxicants In Food Packaging And Household Plastics: Exposure And Health Risks To Consumers. 2014;95–123.
- Rafique I, Kausar A, Muhammad B. Epoxy resin composite reinforced with carbon fiber and inorganic filler: overview on preparation and properties. Polym-Plast Technol Eng. 2016;55(15):1653–1672. doi: 10.1080/03602559.2016.1163597
- Sultan MTH, Rajesh M. Repair of advanced composites for aerospace applications. Jayakrishna, K. Eds. CRC Press; 2022.
- Hoseinlaghab S, Farahani M, Safarabadi M. Improving the impact resistance of the multilayer composites using nanoparticles. Mechanics Based Design Of Structures And Machines. 2023;51(6):3083–3099. doi: 10.1080/15397734.2021.1917424
- Ghanbarzadeh B, Oleyaei SA, Almasi H. Nanostructured materials utilized in biopolymer-based plastics for food packaging applications. Crit Rev Food Sci Nutr. 2015;55(12):1699–1723. doi: 10.1080/10408398.2012.731023
- Hiremath V, Shukla DK. Effect of particle morphology on viscoelastic and flexural properties of epoxy–alumina polymer nanocomposites. Plast Rubber Compos. 2016;45(5):199–206. doi: 10.1080/14658011.2016.1159778
- Sadhu SPP, Siddabattuni S, Ponraj B, Molli M, Sai Muthukumar V, & Varma KBR. Enhanced dielectric properties and energy storage density of interface controlled ferroelectric BCZT-epoxy nanocomposites. Compos Interfaces. 2017;24(7):663–675. doi: 10.1080/09276440.2017.1262665