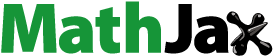
ABSTRACT
Lenalidomide is a key drug in multiple myeloma. Moreover, Al-ZnO has garnered significant attention due to its distinct physicochemical characteristics. This investigation introduces an economic approach for fabrication and characterization of Al-ZnO nanoparticles by SEM, TEM, FT-IR, XPS, UV-VIS, EDS, and XRD techniques. Subsequently, the research explored capacity of the created nanostructure to serve as a nanocarrier for Lenalidomide. Findings indicated an approximate 71% drug loading capacity that is dependent on pH and duration time. The release profile depicted a gradual release under physiological conditions (pH 7.4, 28%), contrasting with a notably accelerated release rate at pH 6.2 (78%) in 48 h. Furthermore, an in vitro assessment of cellular cytotoxicity performed on U266 multiple myeloma cancer cell line to evaluate safety and effectiveness of Al-ZnO nanoparticles. Results from MTT assay affirmed the potential of this nanoparticle as a promising vehicle for Lenalidomide delivery.
Introduction
Lenalidomide is a drug that belongs to a class of medications known as immunomodulatory drugs (IMiDs). It has been widely used in the treatment of multiple myeloma, a type of blood cancer that affects plasma cells in the bone marrow. Lenalidomide is considered a cornerstone of therapy for multiple myeloma and has significantly improved outcomes for patients with this disease. Lenalidomide has potential applications in the treatment of multiple myeloma and exerts its anticancer effects through multiple mechanisms, including enhancing the immune response against cancer cells, inhibiting the growth of blood vessels that support tumour growth, and inducing cancer cell death. Therefore, lenalidomide has become a standard of care for many patients with this disease. Its efficacy and tolerability have made it a key component of treatment strategies for both newly diagnosed and relapsed/refractory multiple myeloma [Citation1–6]. The limited bioavailability of Lenalidomide is linked to challenges like permeability, poor wettability, aqueous solubility, and short half-life [Citation7]. Consequently, akin to many chemotherapy drugs, Lenalidomide is associated with notable side effects like bone marrow suppression, fatigue, gastrointestinal symptoms, skin reactions, peripheral neuropathy, thrombosis, and risk of infection [Citation8–10]. Researchers have focused on utilizing nano-particulate drug delivery systems to enhance Lenalidomide therapeutic effectiveness while mitigating adverse effects. To address the issue of severe side effects stemming from high drug doses, different types of nanocarriers enable the use of lower drug doses [Citation11–14]. Over the years, several nanostructures including C60, magnetic nanoparticles, and carbon nanotubes have been explored as promising delivery agents for Lenalidomide. Based on the rich historical background, extensive studies have been conducted to develop novel and efficient structures for the effective delivery of Lenalidomide [Citation15–22]. Among various nanomaterials, Al-ZnO nanostructure can be optimized for drug delivery, as well as for adsorption of toxic substances, offering promising treatment options with reduced side effects. Al-ZnO has distinctive properties and can be distinguished for numerous researchers. In this context, we aimed to present a simple method to synthesize Al-ZnO and explore its application in the delivery of Lenalidomide.
Experimental details
Materials and methods
We synthesized precursors using high-purity compounds zinc acetate dihydrate (Zn(C2H3O2)2. 2 H2O), hydrated aluminium nitrate (Al(NO3)3.9 H2O), and absolute EtOH. Lenalidomide was procured from Sigma-Aldrich, while other chemicals were sourced from reputable suppliers. Deionized water was used in preparing all aqueous solutions. FT-IR spectra were recorded by a Shimadzu 8700 instrument. X-ray diffraction (XRD) patterns obtained by using a STOE diffractometer with monochromatic Cu Kα (λ 1.5406 Å) radiation at 40 kV and 35 mA. UV-visible spectroscopy was performed on a Photonix instrument. Photoelectron spectroscopic measurements were carried out using a DLD AXIS instrument. SEM conducted on a Philips XL-30 at the accelerating voltage 26 kV. Particle-size and morphology of nanoparticles characterized using transmission electron microscopy (TEM) on Philips CM-200.
Synthesis of Al-ZnO nanoparticles
Al-ZnO nanoparticle was synthesized by appropriate amounts of zinc acetate dihydrate Zn(OAC)2.2 H2O as precursor, ethanol as solvent and hydrated aluminium nitrate Al(NO3)3.9 H2O as the doping source. The mixture kept under constant stirring for 20 min until formation of a whitish solution. Then, the obtained homogeneous solution dried at supercritical conditions using iso-propanol. The molar ratio of Al/Zn was fixed around 3.5% and both primary solutions were used at the same conditions. After reaching the supercritical conditions, the solvent was removed immediately and the autoclave was allowed to cool spontaneously to room temperature. The obtained powders were then characterized without additional treatments.
Lenalidomide loading on Al-ZnO
To load Lenalidomide, 25 mg of Lenalidomide was dissolved in 12 mL absolute ethanol by sonication. Next, 220 mg of the previously synthesized Al-doped ZnO nanoparticles was added to this solution. Then, the resulting suspension ultrasonicated for three cycles. Subsequently, the mixture was stirred for 24 h. After that, the drug-loaded nanomaterial was isolated by centrifugation. The collected nanoparticles were washed by cyclohexane and finally dried in vacuum at ambient temperature. The quantity of drug-loaded nanomaterial was determined by measuring absorbance of the supernatant solution at 295 nm using EquationEquation 1.(1)
(1) shows the UV-Vis spectral changes of the drug solution during loading onto Al-doped ZnO nanoparticles. This experimental procedure revealed 71% drug-loading efficiency under the specified conditions ().
Figure 1. UV-Vis spectral changes of the drug solution during loading onto Al-doped ZnO nanoparticles.

In the equation provided Mt shows the concentration of Lenalidomide in the filtered solution, M0 denotes the concentration of Lenalidomide in primary solution, and MN shows amount of Al-ZnO used for the loading process.
Cell culture and cytotoxicity assay
The MTT assay is a common method used to assess cell viability and cytotoxicity in biological research. In this assay, yellow tetrazolium dye MTT (3-(4,5-dimethylthiazol-2-yl)-2,5-diphenyltetrazolium bromide) is converted by metabolically active cells into insoluble purple formazan crystals. The amount of formazan formed is proportional to the number of viable cells. Now, when studying the cytotoxicity of nanoparticles using the MTT assay, researchers would expose cells to varying concentrations of these nanoparticles. If the nanoparticles are toxic to the cells, they would inhibit cell metabolism and reduce the conversion of MTT to formazan, resulting in a decrease in the purple colour formation. This decrease indicates reduced cell viability and increased cytotoxicity. By measuring the absorbance of the purple formazan at a specific wavelength, researchers can quantify the level of cytotoxicity induced by the nanoparticles at different concentrations. This assay provides valuable information on the impact of the nanoparticles on cell health and can help researchers in assessing the safety and potential biomedical applications of these nanoparticles. In the experiment assessing cytotoxicity of Al-ZnO using the cell viability assay, the following steps were performed. At first, cells were plated in a 110 µL medium at a concentration of 1 × 105 cells/mL in 96-well culture plates and incubated for 24 h. Then, Al-ZnO nanoparticles at the concentration up to 300 µg/mL were added (50 µL) and allowed for incubation for 48 h. After incubation, the supernatant was removed and 105 µL of the medium containing 22 µL of MTT solution was added. Then, the mixture incubated for 5.5 h and absorbance was read to assess the cell viability by a spectrophotometer.
Results and discussion
Characterization of Al-ZnO
shows FT-IR spectra of Al-ZnO, Lenalidomide, and Al/ZnO-Lenalidomide (). The FT-IR spectrum of Al-ZnO showed a strong absorption at 590 and 490 cm−1, confirming successful preparation of Al-doped ZnO (). The broad band in the range of 3200–3400 cm−1 is due to hydroxyl stretching vibration of the adsorbed water. exhibits the FT-IR spectrum of Lenalidomide. The intense characteristic bands at 3400 and 1744 cm−1 are attributed to N–H and C=O, respectively. The shifts in the FT-IR spectrum of Al/ZnO-Lenalidomide in confirm interaction of Lenalidomide with the nanoparticle after loading of drug [Citation23]. As a result, the shifts in the FT-IR spectra can provide valuable information about the interactions between the drug molecules and the performed nanoparticle. The presence of the drug molecules on the nanoparticle surface can lead to new intermolecular interactions, such as hydrogen bonding or van der Waals forces, between the drug and the nanoparticle. These interactions can cause changes in the vibrational frequencies of functional groups in both the drug and the nanoparticle, resulting in shifts in the FT-IR spectra. Moreover, when a drug is loaded onto nanoparticles, it may undergo conformational changes or structural rearrangements due to interactions with the nanoparticle surface. These structural changes can alter the vibrational modes of the drug molecules and lead to shifts in the FT-IR peaks. In addition, distribution of drug molecules within the nanoparticle carrier can influence the relative intensities of the FT-IR peaks corresponding to the drug and the nanoparticle components. describes XPS analysis of the synthesized Al-ZnO () as well as the survey spectrum of Zn 2p (), O1s (), and Al2p () to determine the distribution and oxidation state of atoms in the prepared sample. The XPS survey spectra of the nanocomposite revealed only Zn, O, C, and Al in the survey spectrum. The signal C in the survey spectrum could potentially arise from accidental carbon pollution. The Zn 2p binding energy of ZnO nanocomposite displayed two fitting peaks approximately located at 1021.82 and 1044.94 eV, corresponding to Zn 2p3/2 and Zn 2p1/2, respectively, as illustrated in . These findings suggest that the surface oxidation state of Zn in the nanocomposite is + 2 [Citation24]. In , the O 1s peaks are observed at 531.75 eV and 534.38 eV. The peak at 531.75 eV is attributed to O2- ions in the Zn-O bond of the Wurtzite framework of ZnO. The peak situated at 534.38 eV is assigned to the OH− group absorbed on the surface of nanocomposite. The two peaks in the region 72–74 eV are associated with Al-O and Al-Zn-O.
Figure 3. XPS pattern of the synthesized Al-ZnO nanoparticles. (a) survey spectrum; (b) Zn 2p region; (c) O1s region; and (d) Al2p region of the survey spectrum.

Morphological analysis of Al/ZnO nanoparticles using SEM and TEM can provide valuable insights into the physical characteristics, size distribution, surface morphology, and structural properties of the nanoparticles. Understanding these characteristics is crucial for assessing the suitability of nanoparticles for drug delivery applications. Morphological analysis using SEM and TEM can contribute to understanding the potential applications of nanoparticles because of the following reasons. SEM and TEM can provide information about the size distribution and morphology of nanoparticles. In drug delivery applications, nanoparticles with controlled size and uniform distribution are preferred for efficient drug loading, targeted delivery, and cellular uptake. Analyzing particle size and distribution using SEM and TEM helps in determining the suitability of nanoparticles for drug delivery systems. In addition, the surface morphology of nanoparticles plays a critical role in interactions with drug molecules, biological membranes, and cellular uptake mechanisms. SEM images can reveal the surface topography, roughness, and chemical composition of nanoparticles, which can impact their ability to encapsulate drugs, control drug release kinetics, and enhance cellular internalization for targeted drug delivery. TEM imaging provides detailed insights into the internal structure, crystallinity, and defects of nanoparticles at the nanoscale level. Understanding the crystal structure and orientation of nanoparticles is important for optimizing drug loading capacity, stability, and release profiles. Structural analysis using TEM contributes to developing Al/ZnO-based drug delivery systems with tailored properties for specific therapeutic applications. Moreover, SEM and TEM can visualize interactions between nanoparticles and drug molecules, as well as interactions with biological components in the delivery system. Observing these interactions using microscopy techniques helps in designing nanoparticles that can effectively deliver drugs to target sites with minimal side effects. Morphological analysis using SEM and TEM can provide mechanistic insights into the behaviour of nanoparticles in physiological environments, such as drug release mechanisms, cellular uptake pathways, intracellular trafficking, and therapeutic effects. Understanding the nanoparticle-drug interactions at the microscopic level aids in optimizing formulation parameters and improving the performance and safety of drug delivery platforms based on nanoparticles. Therefore, morphological analysis of aluminium nitride nanoparticles using SEM and TEM offers crucial information on particle size, distribution, surface morphology, structural properties, particle interactions, and mechanistic insights that are essential for evaluating their potential applications in drug delivery. By understanding the physical characteristics and behaviour of nanoparticles at the nanoscale, we can design more effective and targeted drug delivery systems for various therapeutic applications. The morphology of Al-ZnO nanoparticle was also examined using SEM () and TEM () [Citation25]. The SEM image exhibited a plate-like morphology, showing some aggregation. The TEM analysis was further determined size and microstructure of Al-ZnO and the particle size around 55 nm was attained. represents the XRD patterns of the synthesized ZnO and Al-ZnO nanostructures. Clear and distinct peaks observed for the synthesized samples confirmed the ideal wurtzite structure of ZnO [Citation26]. The obtained phases were pure and no peak due to impurities was detected. The slight shift of peaks towards lower 2θ values was due to the presence of aluminium content. The XRD pattern of Al-ZnO can be utilized to assess the crystallite size using Scherrer model (EquationEquation 2(2)
(2) ).
The mean crystallite size around 35 nm was determined using EquationEquation 2(2)
(2) , in which λ, β, and θ denote wavelength of x-ray, broadening of diffraction peak, and Bragg diffraction angle, respectively, determined in radian at half-maximum of the full-width. Additionally, presence of intense and sharp diffraction peaks confirmed high crystallinity of the synthesized nanomaterials.
By analysing the cumulative intensity in XRD patterns, we can assess the degree of crystallinity, identify crystalline phases present in the material, monitor phase changes, and evaluate the quality of crystal structures. This information is crucial for understanding the structural properties, stability, and performance of materials in various applications, including in fields like materials science, nanotechnology, pharmaceuticals, and catalysis. The degree of crystallinity (Dc) can be assessed by EquationEquation 3
(3)
(3) from X-ray data.
I(hkl) shows the intensity of the (hkl) diffraction peak and represents the brightness of reflection, while ∑I(hkl) refers to intensity of diffractions. I0 is the reference sample and signifies the intensity of the sample for comparison. Therefore, the degree of crystallinity of Al-ZnO was estimated to be approximately 85%. The estimated crystallinity of prepared nanomaterial can impact various aspects of their performance in drug delivery applications, including controlled drug release, drug loading capacity, stability, targeted delivery, biocompatibility, and toxicity. By tailoring the crystallinity of nanomaterials, we can optimize their properties to meet specific requirements for effective drug delivery systems, leading to improved therapeutic outcomes and enhanced patient care. The chemical composition analysis is crucial for monitoring the dopant concentration. show EDX analysis of Al doped ZnO, confirming the presence of Zn, O, and Al elements in the sample. According to the performed synthetic method and based on the EDX analysis, the atomic ratio of Al/Zn would be around 2.5%. DRS spectroscopy was also used to assess optical features of Al-ZnO () [Citation26]. Notably, a distinct band in the UV-Vis spectrum of nanoparticles is the characteristic band that can be used to determine the band-gap energy of this semiconductor using the Tauc model. This band originates from electron transitions from VB (valence band) to CB (conduction band, O2p → Zn3d). In the case of Al-ZnO, the mentioned peak shifted from 395 to 405 nm compared to pure ZnO, indicating the close proximity of the Al optical component within the nanocomposite. The calculated band gap of 3.34 eV for pure ZnO and 3.21 eV for Al-ZnO were, respectively, attained. The red shift in the absorption band of Al-ZnO compared to ZnO is attributed to the electronic interactions between ZnO and Al.
Loading/Release experiments
Obviously, pH of the environment has a significant impact on the efficiency of the loading process in drug delivery systems. pH can affect the loading process because affect drug solubility, carrier stability and charge, chemical reactions, and more importantly drug–carrier interactions. Therefore, the loading efficiency may be influenced by the pH conditions during the loading process, as they can impact the subsequent release kinetics of the drug. Understanding the pH sensitivity of both the drug and carrier material, and optimizing the loading process under specific pH conditions, can help enhance the efficiency and effectiveness of drug loading in various drug delivery systems. In this study, Lenalidomide loading efficiency on Al-ZnO nanoparticles was found to be approximately 71%. This result indicates that Al-ZnO can act as an efficient nanocarrier for encapsulation of Lenalidomide. Furthermore, the release of Lenalidomide was investigated and it was inferred that release time and pH strongly affected the release pattern (). Under physiological pH 7.4, release process occurred gradually (around 29%) in 48 h. In the lower pH 6.2, the release process was clearly accelerated, reached the value of ~ 73% within the same 48 h. These results suggested that drug-release happened slowly at the normal pH condition, reducing toxicity towards healthy cells. In the presence of metabolic active surrounding for tumour cells (pH 6.2), the release of drug was increased, leading to increased bioavailability of the drug-loaded nanoparticle in targeted cancer tissues. The differential release of drug from nanoparticles with slow release under physiological conditions and accelerated release in tumour environments can enhance the effectiveness of the drug delivery system by providing prolonged therapeutic effects, reducing systemic toxicity, improving tumour targeting, enhancing drug penetration, synergizing with other therapies, and minimizing drug resistance. This tailored release profile can optimize treatment outcomes and improve the overall efficacy of the drug delivery system in cancer therapy.
Three distinct pathways can be explored to understand interactions between Al/ZnO nanoparticles and Lenalidomide drug molecules. They include hydrophobic interactions, hydrogen bonding, and Lewis acid-base interactions (). The hydrophobic interaction presents a fascinating aspect as, despite Lenalidomide showing a stronger inclination towards non-hydrophobicity, it still demonstrates adsorption affinity through its non-hydrophobic groups. Hydrogen bonding is a prevalent occurrence in the absorption of polar drugs, where hydrogen bonding donors of Lenalidomide interact with acceptor centres on Al/ZnO. On the contrary, Lewis acid-base interaction primarily involves the amino groups of the drug molecules. Nevertheless, the tertiary amine in Lenalidomide may lead to steric congestion, therefore, potentially impeding this type of interaction. Which interaction pathway dominates in the adsorption of drug onto nanomaterials can depend on various factors such as the surface chemistry of the nanomaterial, the specific functional groups present on both the nanomaterial and the drug molecule, and the environmental conditions. In general, the dominant pathway can vary for different nanomaterial-drug systems. Some nanomaterials may strongly favour hydrophobic interactions due to their surface properties, while others may exhibit a preference for hydrogen bonding or Lewis acid-base interactions. Overall, the synergistic contributions of hydrophobic interactions, hydrogen bonding, and Lewis acid-base interactions collectively facilitate the adsorption of drug onto nanomaterials. The relative importance of each interaction pathway in determining the overall adsorption behaviour will depend on the specific characteristics of the nanomaterial and the drug molecule involved in the system.
The in-vitro cytotoxicity of al-ZnO
The MTT assay can provide quantitative data on cell viability and metabolic activity, which can support the conclusion that Al/ZnO nanocarriers bearing drug molecules exhibit higher cytotoxicity compared to the free drug. The MTT assay is a widely used colorimetric assay to measure cell viability and proliferation based on the metabolic activity of cells. In the MTT assay, a yellow tetrazolium salt (MTT) is reduced by mitochondrial dehydrogenases in metabolically active cells to form purple formazan crystals, indicating viable cells. When testing cytotoxicity of a drug, both the free drug and the drug loaded in a nanocarrier like Al/ZnO are tested on cell cultures. Cells exposed to the drug-loaded nanocarrier are expected to uptake the nanocarrier, release the drug inside them, and exhibit cytotoxic effects. The assay can help demonstrate the efficacy of the nanocarrier in delivering the drug and enhancing its cytotoxic effects on the target cells. According to the above considerations, the cytotoxicity of nanoparticles was investigated on U266 cells using MTT assay. Thus, both loaded and free drug were tested for cytotoxicity assay in 24 h (). The obtained results demonstrated that drug-loaded Al/ZnO nanoparticles exhibit significantly higher cytotoxicity compared to the free drug against the U266 cell line in the concentration range 0–175 µg/mL. Furthermore, the toxicity of Al/ZnO nanoparticles was evaluated against normal cell-line L929, as presented in . This experiment revealed minimal toxicity of nanoparticles within the examined concentration, indicating that Al/ZnO is more toxic to the performed cancer cells compared to normal cells.
The higher cytotoxicity of Al/ZnO nanoparticles towards cancerous cells compared to normal cells can be attributed to several factors, including increased cellular uptake, differences in cell metabolism, enhanced reactive oxygen species (ROS) production, disruption of cancer cell-specific signalling pathways, and differences in membrane properties. Nanoparticles may exploit these differences to interact preferentially with cancer cell membranes, leading to specific cytotoxic effects. Overall, the complex interplay of these factors can contribute to the higher cytotoxicity of nanoparticles towards cancerous cells compared to normal cells, offering a promising avenue for targeted cancer therapy using nanotechnology.
It should be mentioned that to further validate the efficacy and safety of the drug delivery system using nanoparticles, additional tests and investigations can be conducted including in vivo studies, targeting specificity, mechanism of action, cellular uptake studies, combination therapies, immunotoxicity assessment, long-term safety evaluations, and clinical trials. By implementing these additional tests and investigations, we can enhance the validation of the drug delivery system, providing valuable insights into its efficacy and safety for potential clinical applications in cancer therapy.
Conclusion
This study introduced a straightforward and economic approach for the preparation of Al-ZnO nanomaterials. Then, the nanoparticles were thoroughly characterized by common techniques SEM, TEM, FT-IR, XPS, UV-VIS, EDS, and XRD. Moreover, the synthesized nanoparticles were effectively employed for delivery of Lenalidomide, achieving a loading efficiency around 71%. The drug loading/release capacity was significantly affected by pH and release time. The release profile showed a gradual release under physiological condition with pH 7.4, 29% release in 48 h. Whereas, the release process was accelerated at pH 6.2 (73% release) in 48 h. Finally, an MTT study assessed the in-vitro cellular toxicity of Al-ZnO nanoparticles on U266 cell line and the obtained results confirmed that the drug-loaded Al-ZnO nanoparticles are significantly more toxic compared to the free drug against the performed cell line in the concentration range 0–175 µg/mL. In conclusion, the findings of drug delivery studies involving aluminium-doped zinc oxide nanoparticles have the potential to drive future research and applications in drug delivery and cancer therapy, leading to the development of novel treatment strategies with improved efficacy and safety profiles.
Author contributions
L.Z. and C.Z.: experiments, Validation, and Data Curation. Q.Y.: Conceptualization, Investigation, Writing and editing. M.L.: Supervision, Writing and editing.
Disclosure statement
No potential conflict of interest was reported by the author(s).
Data availability statement
The data that support the findings of this study are available from the corresponding author upon reasonable request.
References
- Sonneveld P, Dimopoulos MA, Boccadoro M, et al. Daratumumab, bortezomib, lenalidomide, and dexamethasone for multiple myeloma. N Engl J Med. 2024 Jan 25;390(4):301–10. doi: 10.1056/NEJMoa2312054
- Schoenbeck KL, Wildes TM. Updated perspectives on the management of multiple myeloma in older patients: focus on lenalidomide. Clin Interventions Aging. 2020 May 4;15:619–633. doi: 10.2147/CIA.S196087
- Alonso R, Cedena MT, Wong S, et al. Prolonged lenalidomide maintenance therapy improves the depth of response in multiple myeloma. Blood Adv. 2020 May 26;4(10):2163–2171. doi: 10.1182/bloodadvances.2020001508
- Armoiry X, Aulagner G, Facon T. Lenalidomide in the treatment of multiple myeloma: a review. J Clin Pharm Therapeutics. 2008 Jun;33(3):219–226. doi: 10.1111/j.1365-2710.2008.00920.x
- Palumbo A, Hajek R, Delforge M, et al. Continuous lenalidomide treatment for newly diagnosed multiple myeloma. N Engl J Med. 2012 May 10;366(19):1759–1769. doi: 10.1056/NEJMoa1112704
- Zhang CW, Wang YN, Ge XL. Lenalidomide use in multiple myeloma. Mol Clin Oncol. 2024 Jan 1;20(1):1–3. doi: 10.3892/mco.2023.2705
- Jafari AM, Morsali A, Bozorgmehr MR, et al. Modeling and characterization of lenalidomide-loaded tripolyphosphate-crosslinked chitosan nanoparticles for anticancer drug delivery. Int j biol macromol. 2024 Mar 1;260:129360. doi: 10.1016/j.ijbiomac.2024.129360
- Merz M, Dechow T, Scheytt M, et al. The clinical management of lenalidomide-based therapy in patients with newly diagnosed multiple myeloma. Ann Hematol.2020 Aug;99(8):1709–1725.
- Attal M, Lauwers-Cances V, Marit G, et al. Lenalidomide maintenance after stem-cell transplantation for multiple myeloma. N Engl J Med. 2012 May 10;366(19):1782–1791. doi: 10.1056/NEJMoa1114138
- Martinez-Høyer S, Karsan A. Mechanisms of lenalidomide sensitivity and resistance. Exp Hematol. 2020 Nov 1;91:22–31. doi: 10.1016/j.exphem.2020.09.196
- Rao SV, Kumar SS. Lenalidomide loaded polymeric nanoparticles for prostate cancer: a review. Res J Pharm Technol. 2021;14(8):4501–4503. doi: 10.52711/0974-360X.2021.00782
- Niknam P, Jamehbozorgi S, Rezvani M, et al. Understanding delivery and adsorption of Lenalidomide drug with ZnONS based on: dispersion-corrected DFT calculations and MD simulations. Physica E Low Dimens Syst Nanostruct. 2022 Jan 1;135:114937. doi: 10.1016/j.physe.2021.114937
- Gong X, Guo L, Zhou R. Exploring the potential use of aluminium nitride nanoparticles in the delivery of lenalidomide anticancer drug. Inorg Chem Commun. 2023 Dec 1;158:111478. doi: 10.1016/j.inoche.2023.111478
- Tan X, Namadchian M, Baghayeri M. Follow up of the prostate cancer treatment based on a novel sensing method for anti-prostate cancer drug (lenalidomide). Environ Res. 2023 Dec 1;238:117261. doi: 10.1016/j.envres.2023.117261
- Harati H, Morsali A, Bozorgmehr MR, et al. β-cyclodextrin-lenalidomide anticancer drug delivery nanosystem: A quantum chemical approach. J Mol Liq. 2021 Dec 15;344:117762. doi: 10.1016/j.molliq.2021.117762
- Jenner MW, Pawlyn C, Davies FE, et al. The addition of vorinostat to lenalidomide maintenance for patients with newly diagnosed multiple myeloma of all ages: results from ‘myeloma XI’, a multicentre, open‐label, randomised, phase III trial. Br J Haematol. 2023 Apr;201(2):267–279. doi: 10.1111/bjh.18600
- Du X, Lin Y, Shuai Z, et al. Nanocomposite hydrogel to deliver the immunomodulator lenalidomide and anti-inflammatory hesperidin locally to joints affected by rheumatoid arthritis. Chem Eng J. 2023 Nov 15;476:146270. doi: 10.1016/j.cej.2023.146270
- Arib C, Spadavecchia J. Lenalidomide (LENA) hybrid gold complex nanoparticles: synthesis, physicochemical evaluation, and perspectives in Nanomedicine. ACS Omega. 2020 Oct 30;5(44):28483–28492. doi: 10.1021/acsomega.0c02644
- Luo Z, Ji L, Liu H, et al. Inhalation lenalidomide-loaded liposome for bleomycin-induced pulmonary fibrosis improvement. AAPS Pharm Sci Tech. 2023 Nov 16;24(8):235. doi: 10.1208/s12249-023-02690-w
- Mai Z, Cao N, Cheng E, et al. Self-assembled Lenalidomide/AIE prodrug nanobomb for tumor imaging and cancer therapy. ACS Appl Nano Mater. 2023 Oct 18;6(21):19807–19817. doi: 10.1021/acsanm.3c03611
- Quach H, Parmar G, Mateos MV, et al. Recent developments in convenience of administration of the anti-CD38 antibody isatuximab: subcutaneous delivery and fast intravenous infusion in patients with multiple myeloma. Clin Lymphoma Myeloma Leukemia. 2024 Feb 12. doi: 10.1016/j.clml.2024.02.004
- Sharifi S, Sheikhi M, Shahab S, et al. DFT study on the interaction of lenalidomide anticancer drug on the Surface of B12N12 Nanocluster. Letters In Organic Chemistry. 2022 Jul 1;19(7):583–595. doi: 10.2174/1570178618666211027102305
- Srinivasan N, Kannan JC. Investigation on room temperature photoluminescence of pure and aluminum doped zinc oxide nanoparticles. Mater Sci-Poland. 2015 Mar 1;33(1):205–212. doi: 10.1515/msp-2015-0021
- Islam MN, Ghosh TB, Chopra KL, et al. XPS and X-ray diffraction studies of aluminum-doped zinc oxide transparent conducting films. Thin Solid Films. 1996 Jul 1;280(1–2):20–25. doi: 10.1016/0040-6090(95)08239-5
- Silva RF, Zaniquelli ME. Morphology of nanometric size particulate aluminium-doped zinc oxide films. Colloids Surf A Physicochem Eng Asp. 2002 Feb 18;198:551–558. doi: 10.1016/S0927-7757(01)00959-1
- Silva RF, Zaniquelli ME. Aluminium doped zinc oxide films: formation process and optical properties. J Non-Crystalline Solids. 1999 Jun 2;247(1–3):248–253. doi: 10.1016/S0022-3093(99)00079-4