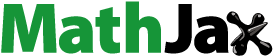
ABSTRACT
This study investigated the impact of precursor solution temperature on the electrodeposition of MnO2 material, which were prepared on a nickel substrate using the electrodeposition method under varying precursor solution temperatures of 25°C, 40°C, 50°C, and 60°C, respectively. These materials were subsequently employed as anodes in lithium-ion batteries to study their electrochemical performance. The results indicate that an increase in temperature induces preferential aggregation and growth of MnO2 nanosheets towards specific orientations, which is advantageous for enhancing the electrochemical performance of MnO2 electrode materials. Notably, the electrode material prepared at a solution temperature of 50°C exhibited optimal performance. Additionally, the charge transfer impedance was lower, which could be attributed to the growth of the highly conductive β-MnO2 main phase under 50°C. This study presents a novel approach for effectively improving electrodeposited nanomaterials, offering insights into the optimisation of electrochemical performance.
Introduction
Nowadays, energy issues have become one of the significant global challenges. The search for efficient and renewable energy solutions, reduction of environmental pollution, and promotion of sustainable development are of paramount importance [Citation1]. With the development of the new energy industry, energy storage technologies face substantial challenges. Lithium-ion batteries, as a mainstream battery technology, possess numerous advantages such as long lifespan, stable performance, and wide operational range. They have facilitated the proliferation of portable electronic devices, gradually replacing traditional batteries like lead-acid and nickel-metal hydride batteries. Lithium-ion batteries have become the preferred choice for mid-to-high-end electronic products and are emerging in the electric vehicle industry and energy storage stations [Citation2,Citation3]. However, issues such as capacity and safety of lithium-ion batteries have become the focus of attention for researchers. Particularly, the improvement of the performance of positive and negative electrode materials is crucial, which is the key to enhancing their overall performance [Citation4–6]. Commercialisation of positive electrode materials has achieved certain results, laying the foundation for the flexible application of lithium-ion batteries through optimised positive electrode materials. In order to further improve the performance of lithium-ion batteries, researchers are gradually exploring measures to improve the positive and negative electrode materials of lithium-ion batteries, and improving their comprehensive performance to meet market demand by developing materials with different electrochemical properties. The commercialisation of cathode materials has achieved certain results, laying the foundation for the flexible application of lithium-ion batteries through optimised cathode materials.
Currently, graphite is predominantly used as the negative electrode material in lithium-ion batteries, with a capacity of approximately 372 mAh g−1. This material exhibits high process maturity, convenient production, and widespread application. However, the limited capacity and rate performance of graphite hinder the continuous improvement of overall battery performance, posing challenges for the development of high-performance batteries [Citation7]. Metal oxide materials, characterised by higher theoretical specific capacities and relatively low costs, have promising prospects. In recent years, there has been continuous and in-depth research in the academic community on transition metal oxides. Among them, manganese oxide (MnO2) is considered an ideal negative electrode material due to its low cost, abundant resources, environmental friendliness, and high theoretical specific capacity. Furthermore, MnO2 possesses excellent electrochemical stability, ensuring prolonged battery cycle life and enhanced safety. This stability is crucial for achieving durable and reliable battery technology. However, challenges persist in its application, such as low conductivity and volume expansion during the insertion/extraction processes. Common strategies to enhance the performance of metal oxide negative electrode materials include nanoscale structuring, porous design, and carbon coating [Citation8].
There are various methods for preparing manganese dioxide (MnO2) negative electrode materials, and MnO2 prepared through different methods exhibits different morphological structures, leading to significant differences in its electrochemical performance. In comparison to traditional electrode preparation methods, the electrochemical deposition (EPD) method offers the advantages of simplicity, convenience, and scalability in production. Moreover, it eliminates the need for additional binders and conductive agents, which is conducive to increasing electrode capacity and reducing volume changes [Citation9]. Researchers have extensively explored various electrochemical deposition strategies for fabricating MnO2-based negative electrodes, including template-assisted electrodeposition, electrochemical deposition, and hydrothermal synthesis. These methods enable the manipulation of MnO2 structures at the nanoscale, enhancing electrochemical performance, such as improving rate capability, capacity retention, and cycling stability.
In this study, the electrochemical deposition method was employed to prepare MnO2 nanomaterials by heating precursor solutions to different temperatures, specifically 25°C (room temperature), 40°C, 50°C, and 60°C. These nanomaterials were deposited on a nickel substrate to investigate the influence of different temperatures on the morphological structure of MnO2 nanomaterials during the electrodeposition growth process. Subsequently, the prepared MnO2 nanomaterials were assembled into half-cells, and their electrochemical performance as electrode materials was studied.
Experimental
Sample preparation
In this experiment, three-dimensional porous nickel foam was employed as the current collector for the electrode material. A mixed solution containing 0.06 mol/L Mn(CH3COO)2 and Na2SO4 was used as the precursor solution. One portion of the solution was kept at room temperature (25℃), while the remaining solutions were heated separately to 40°C, 50°C, and 60°C using a heatable magnetic stirrer. Subsequently, using four different precursor solutions at these temperatures, electrodeposition was carried out for 5 minutes in a three-electrode system at a constant voltage of 0.6 V using a CS350 electrochemical workstation. In the three-electrode system, the working electrode, reference electrode, and counter electrode were made of porous nickel foam, saturated Ag/AgCl, and platinum sheet electrodes, respectively, as illustrated in .
Testing and characterization
All MnO2 electrodes were examined using SEM and TEM to observe their surface morphology and microstructure. To evaluate the electrochemical performance of the prepared MnO2 electrodes, CR2032 coin cells were assembled with metallic lithium as the negative electrode and MnO2 as the positive electrode. Rate capability and cycle performance tests of the batteries were conducted using the CT2001A battery performance testing system in this experiment. Additionally, cyclic voltammetry (CV) and electrochemical impedance spectroscopy (EIS) tests were performed on a CHI660E electrochemical workstation.
Results and discussion
SEM and TEM analysis
The SEM images of MnO2 electrode materials prepared by electrodeposition using solutions of Mn(CH3COO)2 and Na2SO4 at different temperatures are shown in . Firstly, it can be clearly observed that nanoscale MnO2 sheets have grown on the foam nickel substrate at different temperatures, indicating the interconnected growth of these MnO2 nanosheets on the surface of the nickel substrate. This interlaced and parallel special structure possesses a larger porosity and specific surface area, which can enhance the wettability between the electrode and electrolyte. Consequently, it facilitates the transmission of lithium ions, alleviating the high impedance of transition metal defects while providing more active lithium insertion sites [Citation10]. Furthermore, with the increase in precursor solution temperature, the microscopic morphology of the MnO2 electrode exhibits a trend of nanosheets evolving into nano-flower structures. This phenomenon can be attributed to the higher temperature environment providing additional energy for the growth of MnO2 nanomaterials, facilitating rapid ion transfer and promoting the anodic oxidation reaction during the deposition process [Citation11]. Consequently, MnO2 continues to grow along the preferential direction of the existing nanosheets, leading to the aggregation of nanosheet tips, which is also reflected in the electrode loading capacity.
In order to further understand the specific structure of manganese dioxide electrodes prepared at different temperatures of the precursor solution, ) of manganese dioxide prepared at a precursor solution temperature of 50°C was selected as TEM images with different magnifications. The two-dimensional nanosheet morphology with staggered pixels can be clearly observed, which is consistent with the SEM analysis results. ) shows the high-precision crystal structure of the sample in the electron microscope image, where crystal and amorphous structures can be observed in different regions of the sample. The small crystals of metal oxides can significantly increase the specific crystal size of materials, which helps to improve their cycling stability in electrochemical performance [Citation12]. This sample selected three different regions for HR-TEM crystal plane concentration measurement, which were 0.243 nm and 0.251 nm, respectively, corresponding to the (210) and (301) crystal planes.
The electrochemical performances
To investigate the electrochemical performance, all samples were assembled into CR2032 half-cells. Initially, in order to enhance the stability of the batteries, all samples were subjected to three cycles of charge and discharge at 0.1C. This pre-charging step was performed to promote the formation of a more uniform solid electrolyte interface (SEI) film on the electrodes, ensuring better stability of the battery. The charge-discharge voltage window was set between 0.01 V and 3 V. The first charge-discharge curves were recorded, as shown in . The first discharge specific capacities of MnO2 electrodes prepared from precursor solutions at temperatures of 25°C, 40°C, 50°C, and 60°C were 571.6 mAh g−1, 915.5 mAh g−1, 1476.7 mAh g−1, and 1152 mAh g−1, respectively. It can be observed that the MnO2 electrode synthesised at 50°C exhibited the highest discharge specific capacity in the initial discharge cycle, which can be attributed to the additional activation energy gained by the precursor solution at 50°C, which enhanced the transfer rate of Mn2+ ions, consequently increasing the loading of active material on the nickel foam substrate. Furthermore, the moderate temperature of 50°C ensured that the thermal motion did not become excessively vigorous, thereby preserving the deposition process. It is worth noting that in the half-cell configuration, manganese dioxide undergoes an alloying reaction with lithium ions, as represented by EquationEquation (2)(2)
(2) [Citation13].
Figure 4. The electrochemical performance of MnO2 battery. (a) Discharge curves of each battery; (b) Rate performance of each battery; (c) Charging and discharging curves of 50°C MnO2 at different current densities; (d) the cycling performance of each battery at a current density of 1C; (e) CV curves of different samples at 0.2 mV s−1; (f) Nyquist curves of different samples.
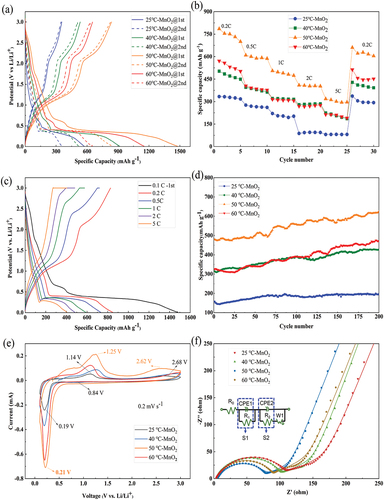
During the initial discharge, MnO2 undergoes a lithium intercalation process, leading to the accumulation of metallic manganese on the high-resistance Li2O matrix. Due to the high resistance of Li2O and the semiconductor nature of MnO2, the phenomenon of discharge specific capacity approaching the theoretical value is observed in the first discharge cycle. Additionally, as evident from the graph, all nano manganese dioxide electrodes prepared from precursor solutions at different temperatures exhibit a voltage change around 0.67 V during the first discharge cycle, which subsequently disappears in the second cycle. Furthermore, a significant decrease in capacity is observed in all electrodes during the second cycle. This phenomenon is attributed to the consumption of active lithium ions and the restriction of ion transport on the electrode surface by the solid electrolyte interface (SEI) film formed during the initial discharge, leading to a substantial capacity decay in the second discharge cycle [Citation14,Citation15].
illustrates the rate performance of the four sets of batteries. It can be observed that the discharge capacity of each sample gradually decreases with the increase in current density, indicating that the charge movement cannot keep up with the changes in current density. Comparatively, the two-dimensional nanosheet MnO2 electrode material synthesised from precursor solution at 50°C exhibits higher discharge specific capacities than the other samples. At 0.2C and 5C, the discharge specific capacities are 745 mAh g−1 and 300 mAh g−1, respectively. Even when the current density is reduced back to 0.2C, it maintains a high discharge specific capacity of 626 mAh g−1, which is twice as high as that at 25°C. This exceptional reversibility and rate capability can be attributed to the increasing film formation on the morphological structure of the electrode with the rise in precursor solution temperature, providing more active sites. However, when the temperature reaches 60°C, the uneven film formation of the active material leads to incomplete lithium ion channels, resulting in a gradual decline in rate performance. Furthermore, the electrochemical deposition method forms porous nano MnO2 shell layers on the nickel substrate, enhancing the utilisation efficiency of MnO2, buffering volume changes, effectively maintaining the integrity of the electrode structure, and ensuring excellent cycling stability. illustrates the charge-discharge curves of the 50°C-MnO2 electrode material under different current densities. It is evident that discharge and charge plateaus appear around 0.3 V and 1.2 V, respectively, corresponding to the redox reactions of MnO2, which is in agreement with findings reported in relevant literature [Citation16,Citation17]. However, at approximately 2.2 V, another discharge plateau persists. According to pertinent studies [Citation18], during the charging process, MnO2 initially loses some oxygen atoms, forming MnO. With continued charging, MnO further accepts electrons and undergoes successive reduction to metallic Mn. Simultaneously, at higher current densities, the reduction reaction becomes more pronounced, leading to a less distinct or even disappearing voltage plateau.
presents the cyclic charge-discharge performance of four sample groups at 1C within a voltage range of 0.01 to 3 V. It is evident that the specific capacities of the other three sample groups after 200 cycles far exceed that of the 25°C-MnO2 sample. Among them, the 50°C-MnO2 cell exhibits the highest specific capacity, reaching 625 mAh g−1, with a Coulombic efficiency close to 99%. This result indicates that MnO2 prepared from precursor solution heated to 50°C possesses superior cyclic performance.Simultaneously, it can be observed that the discharge specific capacities of all samples exhibit a continuous upward trend. On the one hand, it is speculated that MnO2 prepared by electrodeposition possesses a porous structure, enhancing the stability of lithium dendrites growing in the gaps during charge-discharge cycles, allowing them to actively participate in the reaction. Additionally, as the number of
cycles increases, the increased wetting between the electrolyte and the electrode promotes more thorough reactions, leading to a continuous rise in capacity. On the other hand, this phenomenon may be attributed to the unique layered crystal structure of metal oxides, which greatly facilitates the insertion and extraction of lithium ions [Citation19]. It is hypothesised that Li0.98MnO2 is formed and a metal-rich lithium layer may be formed at the interface of the lithium-ion intercalation phase, contributing to the storage of remaining Li+ ions after that.
Furthermore, MnO2 exhibits various crystal forms, and its electrochemical performance varies significantly. During the deposition process from precursor solutions, numerous factors influence the formation of polycrystalline MnO2, including Mn2+ concentration, voltage magnitude, and solution temperature. Since the
lattice energy differences between polycrystals are typically small, solution temperature variations can significantly impact the generation of different MnO2 phases. According to Xin et al. [Citation20], the predominant phase of electrochemically deposited MnO2 in a conventional aqueous electrolyte is ε-MnO2. Among all polycrystalline forms, ε-MnO2 exhibits the lowest electronic conductivity (2.0 mS m−1), making it less favourable for electrochemical reactions. At a temperature of 50°C, the primary phase of MnO2 deposition shifts to γ-MnO2. The electronic conductivity of γ-MnO2 (403.1 mS m−1) is two orders of magnitude higher than that of ε-MnO2 (electronic conductivities for α-MnO2 and β-MnO2 are 111.2 mS m−1 and 974.8 mS m−1, respectively). This inherently low-resistance deposition phase theoretically enables a higher areal capacity loading. Therefore, in conjunction with , we speculate that at a precursor solution temperature of 25°C, the primary phase of MnO2 obtained through electrochemical deposition is ε-MnO2, characterised by the lowest conductivity. As the temperature increases, other phases gradually emerge, leading to variations in conductivity: at 40°C, γ-MnO2 becomes predominant; at 50°C, β-MnO2 becomes the primary phase; at 60°C, β-MnO2 diminishes, giving rise to some α-MnO2. The prevalence of β-MnO2 results in the highest conductivity for 50°C-MnO2, thereby demonstrating superior cyclic performance.
displays the cyclic voltammetry (CV) curves of all cells within a voltage range of 0.01 to 3 V at a scan rate of 0.2 mV s−1. Initially, two sets of oxidation peaks appear around 1.14–1.30 V and 2.62–2.68 V, representing the processes of elemental Mn oxidation to MnO and MnO oxidation to MnO2, respectively. A weak reduction peak at approximately 0.84 V corresponds to the reduction of MnO2 to MnO, while a sharp reduction peak at 0.21 V indicates lithium insertion into manganese dioxide, resulting in the reduction of manganese oxide to elemental Mn [Citation21,Citation22]. Furthermore, with the increase in precursor solution temperature, the intensity values of the peaks gradually increase. The peak intensity and width reach their maximum at a precursor solution temperature of 50°C, and a subsequent increase in the precursor solution temperature from 50°C to 60°C leads to a decreasing trend in peak intensity. presents Nyquist plots of the electrode materials after three cycles at frequencies ranging from 0.01 Hz to 100 kHz. The plots were fitted using the equivalent circuit depicted in the inset, where R0 represents the external circuit resistance (intersection point of the high-frequency semicircle with the x-axis), R1 is the charge transfer resistance at the electrolyte and solid electrolyte interface (SEI) interface (weak semicircle radius in the high-frequency region), R2 is the charge transfer resistance at the electrolyte and electrode material interface (radius of the second semicircle), W1 corresponds to the Warburg impedance representing the diffusion process (straight line in the low-frequency region), CPE1 is the space charge capacitance at the electrolyte and SEI interface, and CPE2 is the space charge capacitance at the electrolyte and electrode material interface [Citation23]. As revealed by the fitting results in , the charge transfer resistance at the interface (R1 + R2) of the 25°C-MnO2 cell is the highest, measuring 101.8 Ω. For the 40°C-MnO2 cell, the charge transfer resistance at the interface is 93.8 Ω, while the 50°C-MnO2 cell exhibits the lowest charge transfer resistance at the interface, at only 70.5 Ω. In contrast, the 60°C-MnO2 cell shows an increased charge transfer resistance at the interface, reaching 80.1 Ω. These observations consistently support the earlier analysis, demonstrating that elevating the temperature appropriately facilitates the formation of the main phase, β-MnO2, with the maximum conductivity, which enhances the electrode material’s conductivity and promotes the aggregation growth of MnO2 nanosheets, providing more active sites for electrochemical reactions, thereby improving the reactivity of the electrode material.
Table 1. Fit values of R0, R1, and R2 for each battery.
Conclusion
In summary, temperature variations in the precursor solution significantly influence the growth of MnO2 nanomaterials during electrodeposition. Utilising these materials as anodes in Lithium-Ion Batteries (LIBs) proves effective in enhancing the electrochemical performance of the cells. SEM results indicate that the microstructure of MnO2 exhibits nanosheet aggregation and forms a nano-flower structure as the precursor solution temperature rises. However, excessively high temperatures weaken this growth process. Electrochemical test results demonstrate that an appropriate increase in precursor solution temperature corresponds to an improvement in the electrochemical performance of the resulting batteries. Specifically, the 50°C-MnO2 electrode displays optimal electrochemical performance, achieving a remarkable initial discharge specific capacity of 1476.7 mAh g−1 at 0.1C. Even after 200 cycles at 0.1C, it maintains a high discharge specific capacity of 625 mAh g−1, showcasing excellent cyclic stability. Its rate performance surpasses that of other samples, being twice as high as the 25°C-MnO2 battery. It maintains a high reversible specific capacity of 304 mAh g−1 even at 5C, concurrently exhibiting the lowest interface charge transfer impedance. These outstanding performances are closely related to the unique layered structure of MnO2. The increase in temperature promotes the growth of the highly conductive β-MnO main phase, while simultaneously causing uniform aggregation and growth of MnO2 nanosheets towards the tips, which provides additional lithium-active sites, significantly enhancing the electrochemical performance of the battery. This observation highlights the critical role of temperature in influencing the morphology and, consequently, the electrochemical properties of MnO2 electrode materials. The findings offer valuable insights into the temperature-dependent optimisation of MnO2 materials for enhanced performance in electrochemical applications.
Disclosure statement
No potential conflict of interest was reported by the author(s).
References
- Baxter J, Bian Z, Chen G, et al. Nanoscale design to enable the revolution in renewable energy. Energy Environ Sci. 2009;2(6):559. doi: 10.1039/b821698c
- Yoon J, Choi W, Kim T, et al. Reaction mechanism and additional lithium storage of mesoporous MnO2 anode in Li batteries. J Energy Chem. 2021;53:276–8. doi: 10.1016/j.jechem.2020.05.029
- Li C, Zhang X, Wang K, et al. Recent advances in carbon nanostructures prepared from carbon dioxide for high-performance supercapacitors[J]. J Energy Chem. 2021;54:352–367. doi: 10.1016/j.jechem.2020.05.058
- Bradley D. Building better batteries[J]. Education In Chemistry. 2010;47(4):124–125.
- Dunn B, Kamath H, Tarascon JM. Electrical energy storage for the grid: a battery of choices. Science. 2011;334(6058):928–935. doi: 10.1126/science.1212741
- Winter M, Brodd RJ. What are batteries, fuel cells, and supercapacitors? Chem Rev. 2004;104(10):4245–4270. doi: 10.1021/cr020730k
- Etacheri V, Marom R, Elazari R, et al. Challenges in the development of advanced Li-ion batteries: a review. Energy Environ Sci. 2011;4:3243.
- Li H. Fundamental sciences of lithium battery[M]. Beijing: Chemical Industry Press; 2021. pp. 22–23.
- Alkali ions pre-intercalation and reduced graphene coating of MnO2 for high-capacity Li-ion battery.
- Luo CG, Chen Y, Tian QH, et al. Ultrathin porous MnO2@C nanosheets for high-performance lithium-ion battery anodes. J Electroanal Chem. 2023;930:117173. doi: 10.1016/j.jelechem.2023.117173
- Yu JX, Wang L, Su L, et al. Temperature effects on the electrodeposition of Zinc. J Electrochem Soc. 2002;150(1):C19.
- Wang XL, Fan XY, Li G, et al. Composites of MnO2 nanocrystals and partially graphitized hierarchically porous carbon spheres with improved rate capability for high-performance supercapacitors[J]. Vol. 93, Carbon; 2015 pp. 258–265.
- Liu JX, Wang JQ, Ni YX, et al. Spinel/lithium-rich manganese oxide hybrid nanofibers as cathode materials for rechargeable lithium-ion batteries. Small Methods. 2019;3(12):1900350. doi: 10.1002/smtd.201900350
- Wu YK, Li XJ, Xiao QZ, et al. The coaxial MnO2/CNTs nanocomposite freestanding membrane on SSM substrate as anode materials in high performance lithium ion batteries. J Electroanal Chem. 2019;834:161–166. doi: 10.1016/j.jelechem.2019.01.001
- Li XL, Zhang YL, Zhong QN, et al. Surface decoration with MnO2 nanoplatelets on graphene/TiO2(B) hybrids for rechargeable lithium-ion batteries. Appl Surface Sci. 2014;313:877–882.
- Min X, Guo MM, Li K, et al. Performance of toluene oxidation on different morphologies of α-MnO2 prepared using manganese-based compound high-selectively recovered from spent lithium-ion batteries. Environ Res. 2022;215:114299. doi: 10.1016/j.envres.2022.114299
- Kong S, Gong YN, Liu P, et al. Synthesis of lithium rich layered oxides with controllable structures through a MnO2 template strategy as advanced cathode materials for lithium ion batteries. Ceram Int. 2019;45(10):13011–13018.
- Zheng LL, Liu Y, Lan JL, et al. Hierarchical heterostructure of interconnected ultrafine MnO2 nanosheets grown on carbon-coated MnO nanorods toward high-performance lithium-ion batteries. Chem Eng J. 2017;330:1289–1296. doi: 10.1016/j.cej.2017.07.153
- Wang H, Hao W, Li T, et al. Elucidating the mechanism underlying the augmented capacity of MoO2 as an anode material in Li-ion batteries. J Mater Chem A. 2023;11(42):23012–23025. doi: 10.1039/D3TA04794F
- Xiao X, Zhang Z, Wu Y, et al. Ultrahigh-loading manganese-based electrode for aqueous battery via polymorph tuning. Adv Mater. 2023;35(33):2211555. doi: 10.1002/adma.202211555
- Min X, Guo MM, Liu LZ, et al. Synthesis of MnO2 derived from spent lithium-ion batteries via advanced oxidation and its application in VOCs oxidation. J Hazard Mater. 2021;406:124743. doi: 10.1016/j.jhazmat.2020.124743
- Yang YL, Ma GZ, Huang JX, et al. Hollow MnO2 spheres/porous reduced graphene oxide as a cathode host for high-performance lithium-sulfur batteries. J Solid State Chem. 2020;286:121297.
- Moazzen E, Kucuk K, Aryal S, et al. Nanoscale MnO2 cathodes for Li-ion batteries: effect of thermal and mechanical processing. J Power Sources. 2020;448:227374. doi: 10.1016/j.jpowsour.2019.227374