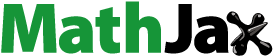
ABSTRACT
Thunderstorm downbursts can impose critical wind loads on long-span transmission line structural system. Existing research has predominantly focused on stationary downbursts, with limited attention given to the analysis of travelling downbursts influenced by environmental wind and parent cloud motion. This paper utilizes the impinging jet method to simulate a travelling downburst at a small Reynolds number, employing the Delayed Detached Eddy Simulation turbulence model for solving the wall-bounded turbulent flow. The radial wind speed distribution of the travelling downburst is subsequently validated against previous experimental, numerical, and observational data. A comparative study is then conducted to discern the differences in the radial wind speed distribution between stationary and travelling downbursts. Finally, a parametric study investigates the influence of jet inlet speed, environmental wind and parent cloud motion on the maximum radial wind speed distribution of the travelling downburst. The findings reveal a significant disparity in the radial wind speed distribution between stationary and travelling downbursts. Notably, parent cloud motion, environmental wind, or their combined effects can alter the height providing the maximum radial wind speed. This variation has implications for the wind load distribution of travelling downburst across the conductor span of the transmission line structural system.
1. Introduction
A downburst is a strong wind capable of causing severe damage to buildings and structures, including long-span transmission line systems. The outflow of the downdraught in a downburst is formed by primary forcing parameters, such as thermodynamic cooling resulting from ice melting and rain evaporation (Vermeire, Orf, and Savory Citation2011). The main vortex of a downburst is caused by the Kelvin – Helmholtz shear instability between the descending airflow and the ambient still air, while the secondary vortex is caused by the friction between the leading edge and the ground (Mason, Wood, and Fletcher Citation2009). Due to these natural characteristics, the downburst wind significantly differs from the traditional synoptic wind in the atmospheric boundary layer.
Downburst can be categorised as stationary and travelling. A stationary downburst is an idealised occurrence that takes place in an undisturbed environment, and its outflow shows a perfect starburst pattern (T. Fujita Citation1985). In contrast, fast-moving downbursts, known as travelling downbursts, occur in a shearing environment. Their outflow becomes deformed due to the movement of the parent cloud, resulting in a shape changing from a circle to an ellipse. Additionally, their outflow exhibits characteristics of environmental wind (T. Fujita Citation1985). Compared with the stationary downburst, the radial wind speed distribution of travelling downburst differs. For example, the wind speed on the front side of the outburst increases, while the wind speed on the rear side of the outburst decreases (T. Fujita Citation1985). Therefore, these characteristics need to be considered when analysing wind loads caused by travelling downbursts.
Researchers conducted many observational studies to analyse the downburst phenomenon. Examples include the previous Northern Illinois Meteorological Research On Downburst (NIMROD), Joint Airport Weather Studies (JAWS) and FAA/Lincoln Laboratory Operational Weather Studies (FLOWS) projects (Wolfson, Distefano, and Fujita Citation1984). Additionally, recent projects such as the Thunderstorm Wind Project in Singapore (Choi Citation2004) and the Ports and Winds project in Italy (Burlando et al. Citation2017) have contributed to our understanding. Despite obtaining accurate local wind speeds, studying downburst phenomenon through field observations remains challenging due to their transient nature, randomness and non-reproducibility. To simulate a flow field, similar to a downburst for research purposes, scholars have developed various experimental and numerical models.
A cloud model (CM) is a large-scale model mainly developed by researchers in the atmospheric science community. It requires a substantial computational domain to encompass the entire cloud domain and its surrounding environment. The model includes complex mathematical parameters, microphysical sources and sink functions to study the life cycle and outflow characteristics of downbursts (Orf et al. Citation2014). Additionally, researchers have developed a simplified subcloud model driven by a negatively buoyant cooling source (CS) to investigate the near-ground flow characteristics of downbursts (Mason, Wood, and Fletcher Citation2009). On the other hand, scholars have proposed an impinging jet (IJ) model, which imposes a vertical flow on the ground to observe the characteristics of a downburst flow that spreads horizontally. Hjelmfelt (Citation1988) demonstrated that the reshaping results of the wall jet experiment (Poreh, Tsuei, and Cermak Citation1967) may be consistent with the downburst outflow profile observed in the JAWS project. Subsequently, many researchers have used IJ or wall jet method in the following research to study flow fields similar to downbursts.
Didden and Ho (Citation1985) utilised the IJ method to analyse the nature of the downburst flow in experimental research. Their conclusion highlights that the quasi-periodic vortex ring produced by the initial Kelvin – Helmholtz instability in the jet shear-layer hits a flat wall or plate, spreading radially in the wall jet region and leading to instability separation. In a separate study, Hall and Ewing (Citation2006) analysed the asymmetric distribution characteristics of downburst outflow through experiments. Their findings suggest that, influenced by the maximum antisymmetric azimuth pattern, the asymmetry distribution is noticeable in the stagnation and the wall jet areas. To capture instability characteristics similar to the downburst flow, Kim and Hangan (Citation2007) employed the IJ method to compare the stationary downburst flow field obtained through steady-state and transient numerical simulations. The comparison results indicate that transient numerical simulation can capture the unsteady separation characteristics of the downburst flow, providing a reasonable velocity distribution and the main vortex structure.
In the past two decades, researchers have extensively utilised the IJ method to study the characteristics of downburst-like flow. This choice is not only due to its applicability but also its suitability and practicality in experimental simulation (Chay and Albermani Citation2005). It is well-known that the outflow produced by the IJ and CS methods represents a similar flow evolution of separation and reattachment. However, the primary vortex produced by the CS method is slower and more gradual. This difference may result in distinct outflow structures in the radial region, primarily determined by the type of initial forcing applied, such as jet impact or negative buoyancy (Demarco et al. Citation2013).
Some researchers have explored the flow field of travelling downburst in addition to focusing on the flow structure and characteristics of stationary downbursts. Orf and Anderson (Citation1999) determined the maximum radial wind speed trajectory and the kinetic energy of travelling downbursts by using the Unsteady Reynolds-averaged Navier–Stokes (URANS) turbulence model. Mason et al. (Citation2010) employed a Scale-Adaptive Simulation (SAS) turbulence model to analyse the influence of environmental wind on the radial wind speed distribution of travelling downbursts. The comparison results indicate that the SAS turbulence model can capture different turbulence length scales of travelling downbursts. In a more recent study, Iida and Uematsu (Citation2019) analysed the wind load effects of travelling downbursts on buildings to obtain detailed information about the flow and pressure fields.
To evaluate near-ground wind load effects or fluid flow characteristics under different conditions, scholars have developed both wall-resolved and wall-modelled methods (Cui, Xiao, and Yuan Citation2020) to characterise and structure wall-bonded turbulent flow. In the case of the wall-resolved method, such as Large Eddy Simulation (LES), the goal is to resolve as many dynamical motion scales as possible, including the dissipative Kolmogorov scale. Consequently, utilising wall-resolved LES in numerical simulation demands a substantial amount of computational resources. The number of grids required is approximately proportional to the Reynolds number raised to the power of 13/7 (), where
represents the Reynolds number of the fluid (Bae et al. Citation2019). However, when using wall-modelled LES to analyse unsteady turbulent flow in engineering applications, larger eddies are directly solved, and only the smaller eddies are simulated (Bose and Park Citation2018).
Wall-modelled methods can be categorised into two main types: single models and hybrid models. When applying a single model, such as LES or an LES-like turbulence model, the governing equations of the turbulence model need to be solved throughout the entire calculation domain (Bae et al. Citation2019). In contrast, when using a hybrid model, such as the Detached Eddy Simulation (DES) turbulence model, the Reynolds-averaged Navier–Stokes (RANS) turbulence model is employed to model small eddies in the near-wall region (G. Wang, Xiao, and Chen Citation2020). Simultaneously, the LES turbulence model is used to solve the large eddies in the outer layer region. In this approach, RANS and LES turbulence models are interconnected, leveraging their respective advantages (Han et al. Citation2020). Moreover, the grid spacing serves as an adjustment scale to analyse the flow separation phenomenon in the boundary layer (Liu et al. Citation2020). To address the flow separation problem caused by grid spacing smaller than the thickness of the boundary layer, scholars modified the DES turbulence model. Specifically, they added a shielding function to the formula to maintain the used RANS layer throughout the entire boundary layer, naming it delayed DES (DDES) (Shi et al. Citation2020).
In prior studies on downburst flow, scholars identified unstable separation‐reattachment of turbulent flow in the near-wall region. Additionally, the choice of Reynolds number value in simulation studies can introduce scale effects, potentially influencing the accuracy of the results obtained (Kim and Hangan Citation2007). When using the LES turbulence model, fine grids and a substantial amount of computing resources in the near-ground region may be necessary to obtain accurate flow characteristics. Conversely, employing the DES turbulence model requires relatively lower computing resources (Jin et al. Citation2020). The range of applicable Reynolds numbers is wider, and the accuracy of the flow field obtained may be comparable to that obtained by the LES turbulence model (Hedges, Travin, and Spalart Citation2002). Therefore, this study will utilise the DDES turbulence model.
Scholars have conducted some experimental studies alongside numerical simulations to analyse the influence of parent cloud motion on the radial wind speed distribution of travelling downbursts. For instance, Letchford and Chay (Citation2002) utilised a travelling wall jet to examine the wind load effects of transient downburst flow on a cubic model. The results indicate that a higher translation speed alters the damage footprint of the downburst into an elongated path. In a similar vein, Sengupta (Citation2007) developed a travelling IJ model to investigate the impact of travelling downbursts on cubic buildings. Simulations of travelling downbursts at a higher translation speed revealed a monotonous increase in peak drag and lift force. More recently, Asano et al. (Citation2019) analysed the flow field of the travelling downburst and its wind load effects on a low-rise building.
In the existing literature, scholars have undertaken both numerical and experimental studies on travelling downbursts. However, there remains a gap in numerical modelling concerning the combined effects of environmental wind and parent cloud motion. The primary objective of this paper is to address this gap. In this study, the IJ method was employed to simulate the stationary downburst within a three-dimensional computational domain. Subsequently, the radial wind speed profile of the stationary downburst was validated using previous experimental, numerical and observational data. Following this, an improved numerical code was utilised to simulate a travelling downburst. A comparative study was then conducted to discern the differences in radial wind speed distribution between stationary and travelling downbursts. Finally, a parametric study was carried out to analyse the influence of jet inlet speed, environmental wind, and parent cloud motion on the radial wind speed distribution of the travelling downburst.
2. Numerical simulation of stationary downburst
This study utilised the non-commercial computational fluid dynamics (CFD) software ANSYS FLUENT 19.0. The Finite Volume Method (FVM) is employed to solve the flow structures and characteristics of stationary downbursts in the form of incompressible flow.
2.1. Model parameters and dimensions
The dimensionless value of the IJ inlet height (H/D) is a key parameter in the IJ model, where H is the height above the flat ground, and D is the diameter of the jet. Wood et al. (Citation2001) recommend setting the IJ inlet height between 0.5 and 5. In this study, the IJ inlet height is set to H/D = 1.5. This choice aligns with the jet inlet height value of H/D = 1.7 used by Letchford and Chay (Citation2002) to investigate the transient flow of the travelling wall jet.
A crucial parameter for the IJ model is the Reynolds number, denoted as =
, where
is the initial flow (air) speed and
is the kinematic viscosity of the flow (air) at 20°C Hall and Ewing (Citation2006) noted that good agreement between the measured mean velocity obtained in the previous experimental work (Gao, Sun, and Ewing Citation2003; Sun Citation2002) and fully established turbulent flow occurs when Reynolds number is in the range of 0.23
to 1.1
. Given that a small
number can be employed in experiments to achieve accurate flow characteristics, in this study, a numerical simulation of stationary downbursts was conducted at a Reynolds number (
) close to the upper limit of the aforementioned range.
Another consideration is that the viscous sublayer of turbulent flow is relatively narrow in a numerical simulation with a large number (Pas Citation2016; Prandtl Citation1925). To ensure the implementation of an adequate number of mesh, Nikitin et al. (Citation2000) and Philippe (Citation2001) suggested adding 11 to 13 extra layers when increasing the Reynolds number by a factor of 10 in numerical simulations. It is evident that applying a higher Reynolds number significantly increases the computational cost to satisfy the requirement that the non-dimensional wall distance of the first cell remains below
= 1 (NIKITIN et al. Citation2000) across the entire surface. Some discussions in the literature indicate that for downburst flows and the flow on the ground surface due to the impinging jet, the implementation of numerical simulations may not strictly adhere to the rule of
= 1 in the wall-normal direction. A potential verification method is used to compare the value of skin friction coefficients obtained from numerical and wind tunnel experimental studies. More discussions on this topic can be found in the work of Nikitin et al. (Citation2000).
Several basic parameters for the numerical simulation of stationary downbursts are derived from the Andrews Air Force Base (AAFB) downburst, as documented by Fujita (Citation1983). The peak radial wind speed is noted as 67 m/s, and the observation time spans approximately 400 s, as detailed in . Previous numerical simulation results by Kim and Hangan (Citation2007) indicate that the maximum radial wind speed is approximately 1.5 times the IJ inlet speed. Consequently, in this study, the IJ inlet speed was set to 44 m/s.
Table 1. Fundamental parameters of the stationary downburst numerical model.
The dimensions of the numerical model are primarily guided by field observation results. Fujita (Citation1981) summarised that observed downburst diameters ranged from 400 m to 4 km. Hjelmfelt (Citation1988) concluded that the downdraught diameter of the downbursts fell within the range of 1.5 km and 3 km. In this study, the downburst diameter (D = 1860 m) observed by Fujita (Citation1983) will be utilised.
Vermeire et al. (Citation2011) employed a calculation domain with a length of 3.5D for two-dimensional IJ modelling. In this study, athree-dimensional computational domain of dimensions 1.5D *8D *7D (height*length*width) with similar length dimensions was used for the numerical simulation of stationary downbursts, as illustrated in .
Sengupta and Sarkar (Citation2008) analysed the influence of nozzle height () ranging from 0.25D to 1D on the radial wind speed profile generated by the stationary downbursts. They concluded that the radial wind speed variation range is less than 2.8% due to the use of differently shaped calculational domains. In this study, a nozzle height of 0.5D was chosen based on the analysis by Sengupta and Sarkar (Citation2008).
2.2. Model scaling
In this study, a length scale of 1/10,000 was employed to simulate a stationary downburst at a small Reynolds number (Re ). To enhance the accuracy of simulation results, optimal algorithms, such as Genetic Algorithm based on H
optimisation algorithm, can be utilised to determine a suitable length scale value for numerical simulations. The time scale 1/1000, as used in the previous study (Li et al. Citation2012), is adopted, resulting in a simulation time of 0.4 s. According to the scaling method introduced in prior research (L. Wang Citation1998), the velocity scale is set to 1/10. Consistent with methods suggested in earlier studies (Kim and Hangan Citation2007; Vermeire, Orf, and Savory Citation2011), the IJ stationary downburst model is designated as the pulse-driven jet, with a constant wind speed of 4.4 m/s to the inlet jet. presents all the model parameters of the stationary downburst.
The scale factor is a key influencing factor in the numerical simulation of the downburst flow field. Jesson et al. (Citation2015) pointed out that the diameter of a full-scale downburst typically ranges between 1 km and 3 km. Consequently, choosing an appropriate scale factor for analysing the building’s response under transient wind loads poses a challenge. In practice, the scaling method proposed by Wang (Citation1998) may be employed to select parameters in experimental research. Asano et al. (Citation2019) utilised an experimental simulator with a diameter (D) of 0.6 m to replicate the AAFB downburst. Assuming the diameter of the AAFB downburst is 1200 m, the length scale can be chosen as 0.6/1200 = 1/2000. Assuming that the IJ inlet speed is 44 m/s, selecting an experimental jet velocity of 7.17 m/s establishes the velocity scale as 1/6. Correspondingly, the time scale (1/2000)/(1/6) = 1/333 aligns with the time scale 1/327 used by Asano et al. (Citation2019) in their experimental research.
2.3. Boundary conditions
For a stationary downburst, all sides of the computational domain are designated as pressure openings (zero in this case), enabling the flow to enter or exit the computational domain based on the relative pressure (Mason, Wood, and Fletcher Citation2009), as shown in .
The wall of the IJ nozzle is chosen as the slip wall, the top face of the numerical domain is set as the interface, and the ground is treated as a rough surface with an aerodynamic roughness length () of 0.002 mm. This value is equivalent to open terrain with
= 0.02 m, corresponding to Terrain Category 2 in AS/NZS 1170.2:2011 (Standards Australia Citation2016).
The equivalent sand grain roughness height () can be derived from EquationEquation (1)
(1)
(1) , where
represents equivalent sand grain roughness height,
is the aerodynamic roughness length, and
is the roughness constant as defined in EquationEquation (1)
(1)
(1) (Blocken, Stathopoulos, and Carmeliet Citation2007).
2.4. Mesh, time step, and turbulence model
All simulations are conducted using a structural grid with hexahedral elements. To emphasise resolution near the ground, the grid expands at a rate of 1.05 from the ground to the top in the wall-normal direction, as illustrated in .
Blocken et al. (Citation2007) propose that the height of the first stretching cell should be less than 1 m (in full scale) and greater than
, where
represents the distance from the adjacent grid cell centre point P of the wall to the wall. Following EquationEquation (1)
(1)
(1) for the equivalent sand grain roughness height, in this study, the height of the first stretching unit is set to
= 2
= 0.08 mm to ensure that the distance
= 1/2
= 0.04 mm is higher than
, as expressed in EquationEquation (2)
(2)
(2) .
Utilising EquationEquations (3(3)
(3) -Equation5
(5)
(5) ), the dimensionless distance value of the centroid of the first element can be determined, as depicted in EquationEquation (6)
(6)
(6) , where
is the skin friction coefficient,
is the density of air,
is the freestream velocity of the flow,
is the wall shear stress, and
is the friction velocity.
According to EquationEquation (7)(7)
(7) , the grid size
(
) used along the X-axis (Z-axis) direction can be derived. Here,
represents the boundary layer thickness of the downburst turbulent flow, and
(
) is the number of cells designed per boundary layer thickness in the X-axis (Y-axis) direction. Given the current computational capacity, the value of
(
) along the X-axis (Y-axis) direction is set to 0.58. Consequently, the corresponding grid size along the X-axis (Z-axis) direction is calculated as
(
) =
/
(
) = 0.0413D, approximately equal to 8 mm. As shown in , the current numerical model of stationary downburst employs approximately 2.8 million cells. On the other hand, using
) = 5 may be an accepted value for implementing the LES turbulence model. However, if this value is adopted, the corresponding grid number of the current stationary downburst numerical model would exceed
million, surpassing the current numerical capacity. Therefore, this article will not delve into an in-depth study of the numerical model’s convergence.
To ensure the resulting stability and satisfy the time step independency requirements, this study adopts a suitable Courant-Friedrichs-Lewy (CFL) value of CFL = 0.8375 (Gant Citation2010). Given that the mesh size along the X-axis directions is 8 mm, the dimensionless time step can be derived using EquationEquation (8)
(8)
(8) , as shown in EquationEquation (9)
(9)
(9) . Each time step necessitates 20 iterations. It is worth noting that this study does not delve into an investigation of time step convergency.
In this study, the author employed a delayed DES turbulence model based on Shear Stress Transport (SST) to investigate the flow field of the downburst, aiming to achieve enhanced performance in adverse pressure gradient flows. The density and viscosity of the flow (air) are 1.25 kg/ and 1.7894
N s/
, respectively. For a stationary downburst, the turbulence intensity of the inlet flow is set to 5%.
2.5. Stationary downburst numerical simulation results and discussions
To investigate the speed contours and radial wind speeds of the stationary downburst flow field, this section utilises the instantaneous u-speed to depict the flow characteristics of the stationary downburst occurring on flat ground at different dimensionless time steps. The u-speed is a component of the baseline simulated wind speed contour, denoted as U = , where u and w represent the radial wind speeds in the X and Z axes (radial and vertical directions), respectively. For simplicity, the u-speed is referred to as the radial wind speed V in the discussion below.
The radial wind speed profiles obtained at various locations along the X-axis are analysed to determine the maximum radial wind speed, denoted as , across the entire numerical domain. The radial wind speed profiles at specific radial locations, such as r/D = 1, r/D = 1.46 and r/D = 2, are plotted in to show the evolution of radial wind speed. Here, ‘r’ represents the measured distance from the centreline of the downdraught to the measurement point along the X-axis. The selected radial locations include the position yielding the maximum radial wind speed, as well as positions discussed in the literature as potential sources for the maximum radial wind speed (Aboshosha, Bitsuamlak, and EL Damatty Citation2015; Jesson et al. Citation2015; Kim and Hangan Citation2007).
Figure 3. Comparison of radial wind speed profiles of current stationary downburst at different radial locations.

The comparative results reveal that at d = 0.197 s, a significant high wind speed of 5.2 m/s forms at the radial position of r/D = 1, with a height of z = 0.03D. This occurrence could be attributed to horizontal flow triggering unstable separation in the boundary layer. Subsequently, at d
= 0.31 s, the maximum wind speed of 7.26 m/s is generated at the radial position of r/D = 1.46, with a height of 0.024D. This maximum wind speed may result from the main vortex descending to the ground after reaching the highest position and merging with consecutive periodic vortices. The evolution of the radial wind speed obtained in the current stationary downburst and its position yielding the maximum radial wind speed aligns with results from previous numerical simulation studies (Aboshosha, Bitsuamlak, and EL Damatty Citation2015; Kim and Hangan Citation2007). Any differences observed could be attributed to variations in numerical simulation settings, such as turbulence models, grid sizes, and Reynolds numbers.
At = 0.31 s, the radial wind speed profile of the current stationary downburst, obtained at the position of r/D = 1.46, is compared with previous experimental data (Iida, Uematsu, and Gavanski Citation2015; Poreh, Tsuei, and Cermak Citation1967; Zhang, Hu, and Sarkar Citation2013), numerical simulations (Zhang, Hu, and Sarkar Citation2013; Orf et al., Citation2014), and observation data (Hjelmfelt Citation1988), as shown in . The radial wind speed V obtained from different heights is normalised by the maximum radial wind speed
, and the height (z) is normalised by the height
providing the maximum radial wind speed.
Figure 4. Comparison of radial wind speed profiles between current stationary downburst and previous results.

A comparative analysis shows that the current stationary downburst results align closely with certain previous experimental findings (Poreh, Tsuei, and Cermak Citation1967) and numerical simulations (Leigh et al.,2014). However, notable discrepancies exist between the current stationary downburst numerical results and certain experimental data (Iida, Uematsu, and Gavanski Citation2015; Zhang, Hu, and Sarkar Citation2013), potentially attributed to variations in Reynolds numbers employed in the respective studies. Additionally, the results of the current stationary downburst fall within the range of previously observed data (Hjelmfelt Citation1988).
3. Numerical simulation of travelling downburst
3.1. Model parameters and dimensions
For the numerical simulation of the travelling downburst, the IJ inlet height (H/D) is set to 1.5 and the simulation is conducted with a small Reynolds number. The parameters from the previously described stationary downburst numerical model are employed with appropriate modifications, such as the inclusion of parent cloud motion and environmental wind. The translational speed of the parent cloud is set to 10.8 m/s. From the ground surface to the top, the mean speed of the environmental wind follows the logarithmic law, as expressed in EquationEquation (10)(10)
(10) . Here,
is the mean horizontal wind component in the surface stress direction,
is the friction velocity, z is the vertical distance from the surface,
is the roughness length,
= 0.4 is the von Kármán constant (Yeo and Shi Citation2018). The turbulence intensity of the formed environmental wind flow at a point with height z is defined as the ratio of the root-mean-square of the velocity fluctuations to the mean wind speed at that height. This is shown in EquationEquation (11)
(11)
(11) , where
is the turbulence intensity,
is the root-mean-square of the fluctuating component of the instantaneous wind speed, and
is the mean wind speed at height z above the ground. The basic wind speed at a height of 10 m is set to 5.14 m/s. The observation time is set to 400 s (T. T. Fujita Citation1983). The diameter (D) is set to 1860 m, and the nozzle height is chosen to be 0.5D. A larger computational domain of 1.5D *10D *7D (height*length*width) is utilised to simulate the travelling downburst, as shown in .
3.2. Model scaling
The length scale and time scale are configured as 1/10,000 and 1/1000, respectively. Consequently, the simulation time is set to 0.4 s. Following the scaling methodology outlined by Wang (Citation1998), the velocity scaling ratio is chosen to be 1/10.
Applying a constant downward speed of 4.4 m/s, the IJ travelling downburst model is characterised as a pulse-driven jet following the approach proposed by Kim and Hangan (Citation2007) (Vermeire, Orf, and Savory Citation2011). To simulate the motion of the parent cloud, a constant translation speed of 1.08 m/s is applied to the IJ. The environmental wind speed at a height of 0.001 m is set to 0.514 m/s. Within the 0.4 s simulation time, the corresponding translation distance of the travelling downburst is approximately 2.3D. summarises the parameters of the travelling downburst numerical model.
Table 2. Fundamental parameters of the travelling downburst numerical model.
3.3. Boundary conditions, turbulence model, mesh, and time step
In the travelling downburst numerical model, the left and right sides of the computational domain are designated as the inlet and outlet for the environmental wind, as illustrated in . All other boundary conditions remain consistent with those of the stationary downburst model.
The travelling downburst turbulent flow is solved using the DDES turbulence model. The density and viscosity of the flowing air are set to 1.25 kg/ and 1.7894
N s/
, respectively.
Due to the translational motion of the IJ, a sliding mesh is used at the interface to simulate a grid that changes over time. Approximately 3.5 M grids are used for the travelling downburst numerical simulation. The non-dimensional time step is chosen to be d = 0.001.
3.4. Travelling downburst numerical simulation results and discussions
To investigate the speed contours and radial wind speeds of the flow field, the instantaneous u-speed is employed to illustrate the flow characteristics of the travelling downburst occurring on flat ground at various non-dimensional time steps. The u-speed is a component of the baseline simulated wind speed contour, denoted as U = , where u and w represent the radial wind speeds in the X and Y axes (radial and vertical) directions, respectively. For convenience, the u-speed is referred to as the radial wind speed V in the following discussion.
By comparing the radial wind speed profiles at different radial locations along the X-axis, the maximum radial wind speed, denoted as , across the entire numerical domain is obtained. illustrates the radial wind speed profiles obtained at specific time steps, namely d
= 0.2 s, d
= 0.279 s and d
= 0.31 s, showcasing the change in radial wind speed. Here, ‘r’ represents the distance from the centreline of the downdraught to the measurement point along the X-axis. These time steps are selected to coincide with the moment when the main vortex separates and reattaches. Furthermore, these specific time steps are chosen to facilitate a comparison between the obtained wind speed profiles of the travelling downburst and the radial wind speed profiles of the stationary downburst.
The comparison results indicate that when d = 0.2 s, a distinct high wind speed (8.976 m/s) forms at the radial location of r/D = 0.84, with a height of 0.12D, possibly due to an unstable separation caused by the main vortex. At d
= 0.279 s, the near-ground maximum wind speed (6.732 m/s) is observed at a radial location of r/D = 1.86, at a height of 0.015D. This occurrence may be attributed to the main vortex moving down to the ground after reaching its highest position and merging with the continuous periodic vortex caused by continuous Kelvin – Helmholtz instability. Additionally, at d
= 0.31 s, a near-ground high wind speed (5.676 m/s) is formed at a radial location of r/D = 2.26, with a height of 0.012D.
Its worth noting that for the current travelling downburst, the height of 25 m (z/D = 0.025D after normalisation to D = 1000 m and H/D = 2) at which the maximum radial wind speed occurs is lower than the height of 30 m that provides the maximum radial wind speed for a stationary downburst, as indicated in studies by Fujita (Citation1981) (Hjelmfelt Citation1988). This difference may be attributed to the influence of parent cloud motion and environmental wind on the propagation at the front side of the outburst.
As shown in , the radial wind speed profile of the current travelling downburst, obtained at the radial location of r/D = 0.84 when = 0.2 s, is compared with previous numerical results (Mason et al., Citation2010). The radial wind speed (V) obtained from different heights is normalised by the maximum radial wind speed (
). The height (z) is normalised by the height (
) providing the maximum radial wind speed. The travelling downburst is denoted as T24.5+E11.7, where T =
represents the ratio of jet translation speed to inlet speed, and E =
represents the ratio of environmental wind speed to jet inlet speed.
Figure 7. Comparison of radial wind speed profiles between current travelling downburst and previous numerical results.

The comparison results demonstrate that the results of the current travelling downburst (T24.5+E11.7) are generally in agreement with the previous numerical results (T50+E28) reported by Mason et al. (Citation2010). Any disparities observed may be attributed to variations in numerical simulation settings, such as different grid sizes and the ratios of travelling speed to environmental wind. Furthermore, distinctions in the main vortex structures formed by the two different driven methods in the IJ and CS numerical models may also attribute to the observed differences.
The time-history results of wind speed, depicted in , are derived from a specific position (1.1495 m, 0.0005 m, 0.02 m) in the numerical domain, equivalent to a specific position (11495 m, 5 m, 200 m) in the field observation. The comparison reveals that the numerical results align closely with the AAFB field measurement results conducted by Fujita (Citation1983). It is essential to note that the extraction location in the numerical simulation may differ from the anemometer’s location in the field measurement, potentially contributing to the observed differences between the numerical and field measurement results.
3.5. Comparison of radial wind speed distribution between stationary and travelling downbursts
As shown in , a comparative analysis is conducted to explore the differences between the radial wind speed profiles of stationary and travelling downbursts. In the case of stationary downburst, the V/ profiles obtained at various radial positions (e.g. r/D = 1, r/D = 1.46 and r/D = 2) are summarised. For travelling downburst, the radial wind speed profiles obtained at specific radial position (r/D = 0.79 and r/D = 2.26) are presented.
As depicted in , when d = 0.197 s or d
= 0.31 s, the maximum radial wind speed of the stationary downburst is observed at the radial position of r/D = 1 or r/D = 1.46. However, for the same time steps, d
= 0.197 s or d
= 0.31 s, the maximum radial wind speed of the travelling downburst occurs at different radial positions and heights. Evidently, a significant disparity exists in the radial wind speed distribution between the stationary and travelling downbursts at the same time step. As shown in , due to actual physical changes induced by the parent cloud motion (Hjelmfelt Citation1988), the radial wind speed of the travelling downburst increases on the front side of the outburst and decreases on the back side of the outburst, compared to the stationary downburst.
3.6. Parametric study
To explore the combined effects of jet inlet speed, environmental wind, and parent cloud motion, this section conducts a comparative study. provides a summary of the pertinent parameters for the travelling downburst numerical model. The height (z) is normalised by the diameter (D) of the travelling downburst. The radial wind speed (V) obtained at various heights at the local position along the X-axis is normalised by the maximum radial wind speed (). The chosen time step corresponds to the moment when the main vortex reattaches in the near-ground area.
Table 3. Parameters of the travelling downburst numerical model.
3.6.1. Travelling downburst with low jet inlet speed
To facilitate comparison with prior numerical simulation results (Masn, Fletcher, and Wood Citation2010), a numerical simulation of travelling downburst is conducted in a three-dimensional domain 2D *10D *7D when the jet inlet speed () is small (
= 2 m/s). examines the impact of jet translation speed and environmental wind speed on the radial wind speed distribution of the travelling downburst in the vertical direction. Increasing the jet translation speed, as observed in T10+E11.7 and T24.5+E11.7, results in an elevation of the height providing the maximum radial wind speed compared to the stationary downburst. This phenomenon aligns with the observations from previous numerical simulations (Mason, Fletcher, and Wood Citation2010), indicating that under the influence of higher environmental wind speed, the height providing the maximum radial wind for a travelling downburst (T50+E28) is slightly higher than that for a stationary downburst. However, a further increase in the jet translational speed leads to a decrease in the height providing the maximum radial wind speed (T49+E11.7).
Figure 10. Comparison of the maximum radial wind speed profiles between stationary and travelling downbursts (=2m/s).

illustrates the effect of environmental wind on the radial wind speed distribution in the vertical direction. Utilising higher environmental wind speed in the numerical simulation, as seen in T24.5+E5.8, T24.5+E11.7, and T24.5+E23.4, results in an increase in the height providing the maximum radial wind speed.
3.6.2. Travelling downbursts with high jet inlet speed
In this section, a relatively large jet inlet speed ( = 4.4 m/s) is employed in the study, and the numerical simulation of the travelling downburst is conducted in a three-dimensional domain of 1.5D*10D*7D.
As depicted in , for T24.5+E6.8, T24.5+E11.7, T24.5+E18, and T24.5+E27, the height providing the maximum radial wind speed for the travelling downburst is lower than the height for the stationary downburst. When utilising a larger jet inlet wind, the changes in environmental wind speed have minimal impact on the radial wind speed distribution in the vertical direction.
Figure 11. Comparison of the maximum radial wind speed profiles between stationary and travelling downbursts ( = 4.4m/s).

In , an increase in the jet travelling speed, such as in T34+E11.7, results in a decrease in the initial slope of the radial wind speed profile and the height providing the maximum radial wind speed.
The comparison results from also suggest that the position providing the maximum radial wind speed can manifest as either a point or a short line with consistent maximum radial wind speed across the entire height range.
It is crucial to note that, owing to the design requirements for transmission line structural systems, conductors must be placed on towers of varying heights. Therefore, changes in wind speed along the vertical direction are a crucial factor in the design of transmission line structural systems (Reinoso et al. Citation2020). For a travelling downburst, parent cloud motion, environmental wind or their combined effects can influence the height providing the maximum radial wind speed. This, in turn, affects the wind load distribution of travelling downburst over the conductor span of the transmission line structural system, warranting in-depth exploration in future research. Additionally, the obtained maximum wind speed of the travelling downburst is in close proximity to the ground and possesses a high wind speed value. This contrasts with the rule identified in the studies of synoptic wind in the atmospheric boundary layer, where, under constant ground roughness, higher wind speeds typically occur at greater heights from the ground. Consequently, the impact of travelling downburst wind loads on low-rise buildings is also deserving of further investigation in future research.
4. Conclusions
This paper employs the impinging jet (IJ) method to simulate a fast-moving downburst, specifically a travelling downburst, influenced by environmental wind and parent cloud motion within a three-dimensional computational domain characterised by a small Reynolds number. Ground roughness is simulated using an equivalent sand grain roughness, scaled based on the aerodynamic length . To model the wall-bounded turbulent flow, the Delayed Detached Eddy Simulation (DDES) turbulence model is utilised. The simulated travelling downburst is validated against previous numerical and observational data. A comparative study is conducted to explore the distinctions in radial wind speed distribution between stationary and travelling downbursts. Finally, an investigation is carried out to assess the impact of jet inlet speed, environmental wind, and the travelling motion of the parent cloud on the radial wind speed distribution of a travelling downburst. Several notable achievements and findings are presented, including:
For a stationary downburst (D = 1680 m, H/D = 1.5, and ground roughness
= 0.02), the maximum near-ground radial wind speed occurs at a height of 0.025D from the ground. In comparison, for a travelling downburst with the same parameters (D = 1680 m, H/D = 1.5 and ground roughness
= 0.02), the maximum near-ground radial wind speed is observed at a slightly lower height of 0.015D.
The evolution of the radial wind speed in a travelling downburst is similar to that of a stationary downburst. Nevertheless, a notable distinction lies in the maximum radial wind speed distribution between the stationary and travelling downbursts. In the horizontal direction, owing to physical changes induced by the motion of the parent cloud, the maximum radial wind speed of the travelling downburst increases on the front side of the outburst and decreases on the rear side. In the vertical direction, the maximum radial wind speed of the travelling downburst manifests at different radial locations and heights. In particular, the motion of the parent cloud, the environmental wind, or their combined effects can either elevate or lower the height, providing the maximum radial wind speed. This variability has implications for the wind loads distribution of a travelling downburst over the conductor span of the transmission line structural system. Given that conductors are designed to be placed on towers with different heights, the potential impact of travelling downburst wind loads on the structural safety of the transmission line system warrants further exploration in future research.
Acknowledgments
This research is supported by an Australian Government Research Training Program (RTP) Scholarship and the Commonwealth Scholarships Program for South Australia. The author would like to sincerely thank Dr. Xing Ma for his suggestions during the completion of this manuscript.
Disclosure statement
No potential conflict of interest was reported by the author.
References
- Aboshosha, H., G. Bitsuamlak, and A. EL Damatty. 2015. “Turbulence Characterization of Downbursts Using LES.” Journal of Wind Engineering & Industrial Aerodynamics 136:44–61. https://doi.org/10.1016/j.jweia.2014.10.020.
- Asano, K., Y. Iida, and Y. Uematsu. 2019. “Laboratory Study of Wind Loads on a Low-Rise Building in a Downburst Using a Moving Pulsed Jet Simulator and Their Comparison with Other Types of Simulators.” Journal of Wind Engineering & Industrial Aerodynamics 184:313–320. https://doi.org/10.1016/j.jweia.2018.11.034.
- Bae, H. J., A. Lozano-Durán, S. T. Bose, and P. Moin. 2019. “Dynamic Slip Wall Model for Large-Eddy Simulation.” Journal of Fluid Mechanics 859:400–432. https://doi.org/10.1017/jfm.2018.838.
- Blocken, B., T. Stathopoulos, and J. Carmeliet. 2007. “CFD Simulation of the Atmospheric Boundary Layer: Wall Function Problems.” Atmospheric Environment 41 (2): 238–252. https://doi.org/10.1016/j.atmosenv.2006.08.019.
- Bose, S. T., and G. I. Park. 2018. “Wall-Modeled Large-Eddy Simulation for Complex Turbulent Flows.” Annual Review of Fluid Mechanics 50 (1): 535–561. https://doi.org/10.1146/annurev-fluid-122316-045241.
- Burlando, M., D. Romanić, G. Solari, H. Hangan, and S. Zhang. 2017. “Field Data Analysis and Weather Scenario of a Downburst Event in Livorno, Italy, on 1 October 2012.” Monthly Weather Review 145 (9): 3507–3527. https://doi.org/10.1175/MWR-D-17-0018.1.
- Chay, M., and F. Albermani. 2005. “A Review of Downburst Wind Models for Dynamic Analysis of Lattice Structures.” In 18th Australasian Conference on the Mechanics of Structures and Materials (ACMSM 18), Sydney, AA Balkema, 353–358.
- Choi, E. C. 2004. “Field Measurement and Experimental Study of Wind Speed Profile During Thunderstorms.” Journal of Wind Engineering & Industrial Aerodynamics 92 (3–4): 275–290. https://doi.org/10.1016/j.jweia.2003.12.001.
- Cui, W., Z. Xiao, and X. Yuan. 2020. “Simulations of Transition and Separation Past a Wind-Turbine Airfoil Near Stall.” Energy 205:118003. https://doi.org/10.1016/j.energy.2020.118003.
- Demarco, G., N. Barrere, G. Sarasúa, A. Marti, O. Acevedo, E. Nascimento, and C. Cabeza. 2013. “Combined Effect of Jet Impingement and Density Perturbation Forcing on the Evolution of Laboratory-Simulated Microbursts.” Journal of Wind Engineering & Industrial Aerodynamics 123:69–76. https://doi.org/10.1016/j.jweia.2013.08.003.
- Didden, N., and C.-M. Ho. 1985. “Unsteady Separation in a Boundary Layer Produced by an Impinging Jet.” Journal of Fluid Mechanics 160:235–256. https://doi.org/10.1017/S0022112085003469.
- Fujita, T. 1985. “The Downburst, Microburst and Macroburst, Satellite and Mesometereology Research (Smrp).” Department of Geophysical Science 36: 75–86. http://hdl.handle.net/10605/262010.
- Fujita, T. T. 1981. “Tornadoes and Downbursts in the Context of Generalized Planetary Scales.” Journal of the Atmospheric Sciences 38 (8): 1511–1534. https://doi.org/10.1175/1520-0469(1981)038<1511:TADITC>2.0.CO;2.
- Fujita, T. T. 1983. Andrews AFB Microburst. Chicago: University of Chicago. http://hdl.handle.net/10605/262003.
- Gant, S. E. 2010. “Reliability Issues of LES-Related Approaches in an Industrial Context.” Flow Turbulence & Combustion 84 (2): 325–335. https://doi.org/10.1007/s10494-009-9237-8.
- Gao, N., H. Sun, and D. Ewing. 2003. “Heat Transfer to Impinging Round Jets with Triangular Tabs.” International Journal of Heat and Mass Transfer 46 (14): 2557–2569. https://doi.org/10.1016/S0017-9310(03)00034-6.
- Hall, J., and D. Ewing. 2006. “On the Dynamics of the Large-Scale Structures in Round Impinging Jets.” Journal of Fluid Mechanics 555:439–458. https://doi.org/10.1017/S0022112006009323.
- Han, X., P. Yu, J. Bai, J. Zhou, and X. Song. 2020. “Hybrid Computational Aeroacoustics Approach Based on the Synthetic Turbulence Model in Eulerian Description.” Aerospace Science and Technology 106:106077. https://doi.org/10.1016/j.ast.2020.106077.
- Hedges, L., A. Travin, and P. Spalart. 2002. “Detached-Eddy Simulations Over a Simplified Landing Gear.” Journal of Fluids Engineering 124 (2): 413–423. https://doi.org/10.1115/1.1471532.
- Hjelmfelt, M. R. 1988. “Structure and Life Cycle of Microburst Outflows Observed in Colorado.” Journal of Applied Meteorology 27 (8): 900–927. https://doi.org/10.1175/1520-0450(1988)027<0900:SALCOM>2.0.CO;2.
- Iida, Y., and Y. Uematsu. 2019. “Numerical Study of Wind Loads on Buildings Induced by Downbursts.” Journal of Wind Engineering & Industrial Aerodynamics 191:103–116. https://doi.org/10.1016/j.jweia.2019.05.018.
- Iida, Y., Y. Uematsu, and E. Gavanski. 2015. “A Study of Downburst-Induced Wind Loading on Buildings.” Journal of Wind Engineering 40 (2): 40–49. https://doi.org/10.5359/jwe.40.40.
- Jesson, M., M. Sterling, C. Letchford, and M. Haines. 2015. “Aerodynamic Forces on Generic Buildings Subject to Transient, Downburst-Type Winds.” Journal of Wind Engineering & Industrial Aerodynamics 137:58–68. https://doi.org/10.1016/j.jweia.2014.12.003.
- Jin, Y., F. Liao, J. Cai, and P. J. Morris. 2020. “Investigation on Rod-Airfoil Noise with High-Order Cell-Centered Finite Difference Method and Acoustic Analogy.” Aerospace Science and Technology 102:105851. https://doi.org/10.1016/j.ast.2020.105851.
- Kim, J., and H. Hangan. 2007. “Numerical Simulations of Impinging Jets with Application to Downbursts.” Journal of Wind Engineering & Industrial Aerodynamics 95 (4): 279–298. https://doi.org/10.1016/j.jweia.2006.07.002.
- Letchford, C., and M. Chay. 2002. “Pressure Distributions on a Cube in a Simulated Thunderstorm Downburst. Part B: Moving Downburst Observations.” Journal of Wind Engineering & Industrial Aerodynamics 90 (7): 733–753. https://doi.org/10.1016/S0167-6105(02)00163-0.
- Li, C., Q. Li, Y. Xiao, and J. Ou. 2012. “A Revised Empirical Model and CFD Simulations for 3D Axisymmetric Steady-State Flows of Downbursts and Impinging Jets.” Journal of Wind Engineering & Industrial Aerodynamics 102:48–60. https://doi.org/10.1016/j.jweia.2011.12.004.
- Liu, K., Y. Wang, W.-P. Song, and Z.-H. Han. 2020. “A Two-Equation Local-Correlation-Based Laminar-Turbulent Transition Modeling Scheme for External Aerodynamics.” Aerospace Science and Technology 106:106128. https://doi.org/10.1016/j.ast.2020.106128.
- Mason, M. S., D. Fletcher, and G. Wood. 2010. “Numerical Simulation of Idealised Three-Dimensional Downburst Wind Fields.” Engineering Structures 32 (11): 3558–3570. https://doi.org/10.1016/j.engstruct.2010.07.024.
- Mason, M. S., G. S. Wood, and D. F. Fletcher. 2009. “Numerical Simulation of Downburst Winds.” Journal of Wind Engineering & Industrial Aerodynamics 97 (11–12): 523–539. https://doi.org/10.1016/j.jweia.2009.07.010.
- Nikitin, N., F. Nicoud, B. Wasistho, K. Squires, and P. R. Spalart. 2000. “An Approach to Wall Modeling in Large-Eddy Simulations.” Physics of Fluids 12 (7): 1629–1632. https://doi.org/10.1063/1.870414.
- Orf, L. G., and J. R. Anderson. 1999. “A numerical study of traveling microbursts.” Monthly Weather Review 127 (6): 1244–1258. https://doi.org/10.1175/1520-0493(1999)127<1244:ANSOTM>2.0.CO;2.
- Orf, L. G., C. Oreskovic, E. Savory, and E. Kantor. 2014. “Circumferential Analysis of a Simulated Three-Dimensional Downburst-Producing Thunderstorm Outflow.” Journal of Wind Engineering & Industrial Aerodynamics 135:182–190. https://doi.org/10.1016/j.jweia.2014.07.004.
- Pas, S. 2016. “The Influence of Y+ in Wall Functions Applied in Ship Viscous Flows.”
- Philippe, R. 2001. “Young-Person’‘s Guide to Detached-Eddy Simulation Grids.”
- Poreh, M., Y. Tsuei, and J. E. Cermak. 1967. “Investigation of a Turbulent Radial Wall Jet.” Journal of Applied Mechanics 34 (2): 457–463. https://doi.org/10.1115/1.3607705.
- Prandtl, L. 1925. “7. Bericht über Untersuchungen zur ausgebildeten Turbulenz.” ZAMM - Journal of Applied Mathematics and Mechanics / Zeitschrift für Angewandte Mathematik und Mechanik 5 (2): 136–139. https://doi.org/10.1002/zamm.19250050212.
- Reinoso, E., M. Niño, E. Berny, and I. Inzunza. 2020. “Wind Risk Assessment of Electric Power Lines Due to Hurricane Hazard.” Natural Hazards Review 21 (2): 04020010. https://doi.org/10.1061/(ASCE)NH.1527-6996.0000363.
- Sengupta, A. 2007. Study of Microburst-Induced Wind Flow and Its Effects on Cube-Shaped Buildings Using Numerical and Experimental Simulations of an Impinging Jet. Ames, IA, USA: Iowa State University.
- Sengupta, A., and P. P. Sarkar. 2008. “Experimental Measurement and Numerical Simulation of an Impinging Jet with Application to Thunderstorm Microburst Winds.” Journal of Wind Engineering & Industrial Aerodynamics 96 (3): 345–365. https://doi.org/10.1016/j.jweia.2007.09.001.
- Shi, W., J. LI, H. Gao, H. Zhang, Z. Yang, and Y. Jiang. 2020. “Numerical Investigations on Drag Reduction of a Civil Light Helicopter Fuselage.” Aerospace Science and Technology 106:106104. https://doi.org/10.1016/j.ast.2020.106104.
- Standards Australia, N. 2016. Structural Design Actions.Part2: Wind Actions, Standards Australia/Standards NewZealand. AS/NZS1170.2: 2011 (R2016). Sydney: standards Australia.
- Sun, H. 2002. The effect of initial conditions on the development of the three-dimensional wall jet. Ph. D. thesis, McMaster University, Hamilton, ON, Canada.
- Vermeire, B. C., L. G. Orf, and E. Savory. 2011. “Improved Modelling of Downburst Outflows for Wind Engineering Applications Using a Cooling Source Approach.” Journal of Wind Engineering & Industrial Aerodynamics 99 (8): 801–814. https://doi.org/10.1016/j.jweia.2011.03.003.
- Wang, L. 1998. “Self-Similarity of Fluid Flows.” Applied Physics Letters 73 (10): 1329–1330. https://doi.org/10.1063/1.121885.
- Wang, G., Z. Xiao, and L. Chen. 2020. “Simultaneous Simulation of Transition and Massive Separation by RANS-LES-Tr Model.” Aerospace Science and Technology 105:106026. https://doi.org/10.1016/j.ast.2020.106026.
- Wolfson, M. M., J. T. Distefano, and T. T. Fujita. 1984. Low-Altitude Wind Shear Characteristics in the Memphis, TN Area Based on Mesonet and LLWAS Data. Chicago, IL, USA: Department of Geophysical Sciences, University of Chicago.
- Wood, G. S., K. C. Kwok, N. A. Motteram, and D. F. Fletcher. 2001. “Physical and Numerical Modelling of Thunderstorm Downbursts.” Journal of Wind Engineering & Industrial Aerodynamics 89 (6): 535–552. https://doi.org/10.1016/S0167-6105(00)00090-8.
- Yeo, D., and L. Shi. 2018. “Computational versus Wind Tunnel Simulation of Atmospheric Boundary Layer Flow for Structural Engineering Applications.” In Wind Engineering for Natural Hazards: Modeling, Simulation, and Mitigation of Windstorm Impact on Critical Infrastructure, 169–191. https://doi.org/10.1061/9780784415153.ch09.
- Zhang, Y., H. Hu, and P. P. Sarkar. 2013. “Modeling of Microburst Outflows Using Impinging Jet and Cooling Source Approaches and Their Comparison.” Engineering Structures 56:779–793. https://doi.org/10.1016/j.engstruct.2013.06.003.