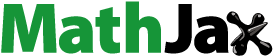
Abstract
Wind-driven single-sided ventilation (SSV) is present in many existing buildings across Europe, and with new near-zero energy building (NZEB) regulations for the refurbishment of the existing building stock, its attractiveness as a noninvasive, low-energy solution is set to continue. As a strategy, however, in addition to its air change rate capacity, the distribution of fresh air is an important evaluation criterion for its performance. Airflow guiding components located in the external opening that enhance the effectiveness of the wind-driven flow in ventilating the occupied zone could improve the quality of indoor environments. To our knowledge, the literature is sparse on the practical implications for ventilation when adopting guiding components such as louvres, an increasingly popular approach. In the present study, the performance of wind-dominant SSV was simulated using RNG and RSM CFD models, with and without louvres at three building orientations, for example, windward, parallel and leeward. The purpose of this study was to investigate whether louvres installed in the opening would improve both the effective ventilation rate and the penetration depth of the flow into the indoor space. The performance of SSV was evaluated using the age of air and interpreting the secondary air circulation inside the room affected by louvres. As a result of these investigations, a newly configured airflow guiding component was designed and compared to the other cases. Results show louvres can play a crucial role in controlling the secondary air circulation inside the room, and they could either improve or worsen the performance of SSV in terms of air-exchange efficiency. It was shown that in most cases, if louvres were the cause of incremental changes in turbulent intensity within the indoor space, then they are effective as an air-exchange efficiency improvement strategy.
1. Introduction
In practice, buildings are vulnerable in terms of reliably providing well-being and thermal comfort for the occupants. They are vulnerable to external disturbances, for example, heat weaves, power outages etc. In a resilient cooling strategy, the building should be able to respond to system failures and recover to its previous equilibrium condition (Attia et al., Citation2021). Therefore, given a building which is fully reliant on mechanical ventilation cannot overcome extreme disturbances such as power outages, natural ventilation can bring more robustness to the design, which leads to a more resilient cooling system. Natural ventilation is a low-energy strategy, whereas mechanical ventilation can constitute 25% of the total energy in such buildings. The disadvantage of natural ventilation is the lack of air-to-air heat recovery in winter (Heiselberg et al., Citation2002; Sacht et al., Citation2016; Schulze et al., Citation2018).
Natural ventilation can be classified as either single-sided ventilation (SSV) or cross ventilation (CV). Although cross ventilation is generally a robust ventilation concept which can provide higher ventilation rates and more adaptive comfort control, the performance of SSV is more sensitive to variability in system conditions, particularly the geometry, compared to that of cross ventilation (Goethals et al., Citation2012; Omrani et al., Citation2017; Wang & Chen, Citation2015; Wei et al., Citation2010a). Nevertheless, cross ventilation can only be used in narrow, open-plan buildings (Wang & Chen, Citation2015). SSV is perhaps the most common form for modern apartment buildings and offices because it has little restriction and can be easily implemented in buildings, thus, it is important to understand the natural ventilation performance of such apartments (Mohamed et al., Citation2011; Wang & Chen, Citation2015). Therefore, evaluating the performance of SSV is of major value as it could be the most vulnerable strategy in natural ventilation, and it is common in most buildings, both dwellings and nonresidential; it is necessary to study how the performance of this system can be improved using either passive or active techniques (Kato et al., Citation2006).
Various AGCs, for example, guide vane, louvre, overhang, and so on can reduce cooling demand and make the airflow more appropriate inside the room. It is indicated that a significant improvement in the indoor thermal comfort condition can be achieved by actuating the window intelligently during summer indoor conditions (Pokhrel et al., Citation2019). Field measurements reported the slot louvre air change rate (ACR) was 6.5% higher compared with the plain opening ACR, and the slot louvre ventilation system has led to steadier ventilation rates (O'Sullivan & Kolokotroni, 2017). Shading louvres lead to an increase in ΔCp for parallel approach flow and a decrease in ΔCp for perpendicular approach flow (Zheng et al., Citation2019). Using Venetian blinds can cause even a little bit higher airflow rate when it is open, in comparison with no shading case (Argiriou et al., Citation2002).
In one aspect, the louvre application can be interpreted as a guide vane, which guides the outside airflow into the room in a preferable manner. In a wind-tunnel study, it was found that for parallel flow locally at the opening, applying a guide vane can improve the normalised airflow rate up to 12 times compared to a plain opening (Kato et al., Citation2006). Overhang drastically enhanced the ventilation rate in the windward direction regardless of the wind speed; however, the ventilation rate slightly decreased for the leeward and side cases (Park et al., Citation2016).
Designing a naturally ventilated building often presents greater challenges than a corresponding mechanically ventilated building (Caciolo et al., Citation2011; Larsen & Heiselberg, Citation2008). Accurately modelling the windows of buildings is important for quantifying airflow in single-sided natural ventilation (Wang et al., Citation2017). Therefore, assessing the complex flow field present in SSV is necessary to realize the flow characteristics in this type of natural ventilation (Najafi Ziarani et al., Citation2023). In the present study, computational fluid dynamics (CFD) is adopted to predict the flow characteristics in the case of wind-dominant SSV. Numerical modelling provides spatial information, for example, predictions of fresh air distribution features of SSV flows (el Telbany et al., Citation1985). It was reported that by adopting the Renormalisation Group (RNG) turbulence model, the discrepancy in the determination of the ventilation rate is acceptable, and the flow distribution inside the building is accurately predicted (Evola & Popov, Citation2006).
With this in mind, the purpose of this study was threefold:
Numerically evaluate the wind-driven near-façade flow in a generic isolated surface-mounted cubic room with a single opening at one side of the building façade.
Numerically investigate the performance of SSV at three building orientations with and without louvres at the opening.
Appraise a novel airflow guiding component at the opening based on near-façade flow features and their interaction with louvres.
As it is mentioned, in order to improve the performance of a system, a detailed evaluation of its characteristics is necessary. In natural ventilation, the main characteristics of the system will be determined by the interaction of the external flow and internal flow. The presence of an isolated surface-mounted cube inside an atmospheric boundary layer will cause phenomena such as vortex shedding, which is transient in essence. The present study uses steady-state simulation, therefore the main focus is on the mean values of the near-facade flow characteristics, and transient features of the flow are out of context of this study albeit they are of major importance.
2. Materials and methods
CFD simulations were conducted using ANSYS FLUENT 2021R1, a finite-volume, general-purpose code. Two types of turbulence models were used: Reynolds-averaged Navier–Stokes (RANS) and Reynolds Stress Model.
The present study used the previous atmospheric boundary layer wind tunnel measurements by Kosutova et al. (Kosutova et al., Citation2019) for CV in a generic surface-mounted scaled cubic building (H = 150 mm × 150 mm × 150 mm) with openings (h = 40 mm × w = 70 mm) at the centre of façades for validation of adopted turbulent methods. The thickness of the building was 10 mm, and three louvres with a thickness of 0.75 mm were applied at the openings with a 15° inclination to the horizontal. Streamwise mean velocities were measured at four vertical lines inside the room () using particle image velocimetry (PIV).
Figure 1. (a, b) Computational domain and boundary conditions in the present study, (c) poly-hederal grid applied in the present study, (d) validation lines provided by previous wind-tunnel study (Kosutova et al., Citation2019).

The inlet velocity profile in the wind tunnel was reported as following a logarithmic equation:
(1)
(1)
where z is the height in the z-direction (vertical direction from the ground),
is the friction velocity,
is the Von Karman constant and
is the roughness parameter (Kosutova et al., Citation2019). The measured stream-wise turbulent intensity, (
), was 10%.
2.1. Computational domain
The computational domain size for the lateral sides (5H), downstream side (15H) and top boundary (5H) was adopted based on the best practice guidelines (Franke et al., Citation2007) as illustrated in and b. The upstream boundary was selected as 3H to accommodate changes in the inlet velocity profile based on the recommendation of previous studies in RANS modelling (Blocken, Citation2015). In the present study, the building height was considered as the reference height, H, and the velocity at building height was considered as the reference velocity ().
2.2. Turbulence modeling
In the present study, it is assumed that the flow is steady state, incompressible, three-dimensional and isothermal (as it is wind-dominant natural ventilation, the effect of buoyancy was ignored). Therefore, the continuity equation for mass transfer and the Navier-Stokes equation for momentum transfer are solved to simulate the flow field. Turbulence was modelled using both a Reynolds Stress Model (RSM) (Pope, Citation2000) and Renormalised-Group (RNG) method (Yakhot et al., Citation1992). Since the
value for building and louvres in all cases was set to be
and this value is higher on the ground surfaces, due to the reduction of computation cost, an enhanced wall function was adopted for both RNG
and RSM models to enable flexibility on near-wall grid resolution.
2.3. Mean age of air, air exchange efficiency and airflow rate calculations
The mean age of air was obtained by solving a transport equation added using a user-defined function (UDF) along with both momentum and continuity equations. The air exchange efficiency, for all cases was then calculated based on EquationEquation (2)
(2)
(2) (Sandberg, Citation1992):
(2)
(2)
where
is the nominal time constant, and
is the mean age of all air present in the room. The simplest method for calculating the airflow rate adopted by many numerical studies (Caciolo et al., Citation2012; Jiang et al., Citation2003), which does not consider turbulent diffusion at the opening, is by integrating the time-averaged normal velocities over the opening area:
(3)
(3)
where the
is the velocity vector normal to the opening, and
is the area of the cell c of the opening.
2.4. Boundary conditions and Discretisation
The logarithmic velocity profile (EquationEquation (1)(1)
(1) ) was applied at the inlet boundary (). In order to apply the turbulent features of the inlet profile to represent 10% measured turbulent intensity, the value of turbulence kinetic energy
was obtained as per EquationEquation (4)
(4)
(4) , assuming
(Kosutova et al., Citation2019) and therefore
(4)
(4)
The value of the turbulence dissipation rate was determined based on the following (Richards & Hoxey, Citation1993):
(5)
(5)
Zero-static gauge pressure was applied at the outlet boundary; no-slip boundary conditions for the building’s walls, louvres and ground and a symmetry boundary condition for lateral and top boundaries were adopted, as demonstrated in . The pressure and velocity distribution coupling is obtained using the SIMPLE algorithm. The second-order linear upwind scheme is adopted as the Discretization method for pressure, momentum, turbulent kinetic energy and turbulent dissipation rate. The convergence criterion of the residuals was set to reduce to less than the threshold of for all equations. The difference between consecutive values of calculated airflow rate at the opening and volume-averaged age of air was monitored to be less than a threshold of
when the residuals reached their determined criterion to ensure that the parameters of interest were stable.
2.5. Grid study
The computational grid was generated by ANSYS FLUENT 2021R1 meshing using polyhederal surface mesh. The building geometry and louvres are modelled according to the wind tunnel sample described above. Three grids with a size of 585,063 (coarse grid), 1,734,404 (basic grid), and 5,039,507 (fine grid), respectively, were generated to study the effect of grid resolution on the results using Grid Convergence Index (GCI) (Roache, Citation1997):
(6)
(6)
where the value of 1.25 is taken for the safety factor,
as recommended, the value of
for
as grid refinement factor, and the value of
for
based on the use of second-order Discretisation schemes for the simulations. The GCI values of 0.98%, 1.68%, 1.69% and 2.5% for lines x/H = 0.2, x/H = 0.4, x/H = 0.6 and x/H = 0.8, respectively () for comparison of the basic and fine grids show that the effect of grid refinement from the basic grid-to-fine grid is not significant; Hence, the present study used the basic grid arrangement for evaluating the case-studies.
2.6. Single-sided natural ventilation cases considered
To assess the effect of airflow guiding components on the performance of SSV in terms of air exchange efficiency, nine separate cases were evaluated; plain opening, louvred opening (as per wind tunnel design [Kosutova et al., Citation2019]), and newly designed airflow guiding components (), when the opening was located at windward (), parallel (), and leeward () façade.
3. Results
3.1. Validation
In order to validate the modelling of the louvre geometry at the opening and the accuracy of the adopted turbulent methods, the mean non-dimensional streamwise velocity profiles at four vertical lines as per obtained using both RNG and RSM models compared with wind-tunnel results (Kosutova et al., Citation2019) is illustrated in , for cross ventilation. The resulting profiles from both the RNG
and RSM models are in good agreement with the experimental measurements, which demonstrates the capability of both models for predicting the airflow distribution in the case of natural ventilation in an isolated surface-mounted cube-shaped room with louvres at the opening. Given the more stable convergence behaviour of the RNG
model compared with the RSM for all the cases of SSV, the RNG
model was adopted as the turbulent model in the present study.
Figure 4. Validation of calculated mean streamwise velocity profiles inside the room compared with wind tunnel study (Kosutova et al., Citation2019).

3.2. Near-façade flow structures
As shown in and b on the windward façade, there exists an upwash flow (from the stagnation point to the upper edge of the building), a downwash flow (from the stagnation point to the ground), and transversal flows (from the stagnation point towards both side edges of the building). Therefore, even in the windward façade, the flow direction is parallel to the opening. This was observed by previous field measurement studies (Caciolo et al., Citation2011; O'Sullivan & Kolokotroni, 2017) and CFD studies (Najafi Ziarani et al., Citation2023). and d demonstrates the upwash near-façade flow as the result of a low-pressure region on the wake of the building, forming a vortex on the wake side of the building by which the indoor flow is affected from this upwash flow locally at the opening. There are transversal flows also on this side of the building façade. On the parallel opening (), however, there is a dominant horizontal parallel flow in the opposite direction of the mainstream as a result of a low-pressure region caused by the separation on the vertical edges of the building. This leads to a different flow structure inside the room compared to leeward and windward openings. It is noteworthy to mention that the illustrations in are streamlines on the surface. However, the flow structures around and inside the room are 3-dimensional, and we always have flow in all directions, which is why even on the parallel side, the streamlines show upwash flow ().
Figure 6. Schematic demonstration of the flow around the surface-mounted cube (Martinuzzi & Tropea, Citation1993).

3.3. Age of air and air-exchange efficiency
shows that the mean age of air reduces by the presence of louvres only on the windward side compared to the plain opening. The age of air significantly worsens by the presence of louvres at the opening for the leeward side. The fact that the wind direction is not a constant parameter in real buildings, and an opening can be on the leeward side, windward side or parallel side intermittently due to different wind directions, demonstrates the necessity of designing airflow guiding components in a way that there will be an improvement in all opening orientations in average. As it is discussed in the previous section, the near-façade flow is dominantly parallel locally at the opening.
On the windward side, the downwash flow is dominant. On the leeward side, the upwash flow is prevailing, and on the parallel side, there is a dominant horizontalflow. In order to enhance the amount of air entering the room on all three sides, the newly designed airflow guiding components with both horizontal and vertical fins () is applied at the opening to allow an examination of the effect of these components on controlling the near façade flow locally at the opening. As it is clear in , the new louvre design in terms of age of air has more stable treatment compared to plain and louvred openings. It is noteworthy to mention that the present study does not claim that the suggested newly designed airflow guiding component (AGC) is the optimum AGC design. It is presented for demonstrating the importance of considering near-façade flow in designing airflow guiding components and effectiveness of this approach on improving the performance of SSV.
3.4. Effect of louvres and airflow guiding components on indoor airflow
The main question in louvre design and the effect they have on the indoor environment is how louvres interact with the near-façade flow and in what way they should be designed to ensure that there will be a positive effect on average for the system's performance. With this approach, the present study looked into the turbulent features of the inlet jet, the relationships between turbulent intensity and air-exchange efficiency, and the jet deflection phenomenon. demonstrates that louvres can cause the jet of air, which enters the room from the bottom side of the plain opening on the windward side, to be deflected and instead enters from the upper side of the opening. This deflection is the cause of the incremental increase in the turbulent intensity of the inlet air and therefore changes in volume-averaged turbulent intensity and air-exchange efficiency (127% improvement), as well as changes in indoor air circulation in this case. The presence of louvres (inclined at an angle of 15 degrees to the horizontal) worsens the air-exchange efficiency on the leeward side compared to the plain opening significantly (50.47% deterioration). The louvres not only cause jet deflection, but they are also causing a decrease in volume-average turbulent intensity in this particular case.
Re-evaluating based on the short-circuiting phenomenon illustrates that when louvres are the cause of separating the inlet and outlet air flow paths at the opening, they can be the cause of improvement in the performance of the system. Although due to the number of cases, the statistically significant relation/correlation between turbulent intensity and AEE cannot be provided in the present study, in most cases when louvres were the cause of increment in turbulent intensity, there is an improvement in AEE ().
4. Conclusions
Given that single-sided natural ventilation is one of the worst-performing ventilation systems to provide comfort and well-being for the occupants, the present study investigated the effect of applying airflow-guiding components at the opening to improve the performance of this system. The possibility of providing a new design for these components through an improved understanding of the near-facade flow characteristics was evaluated. It was demonstrated that the flow structures inside the room is determined by the near façade flow in wind-dominant single-sided natural ventilation, which can be changed and controlled by applying louvres at the opening. The AGCs/louvres not only cause jet deflection but also they are caused a decrease in volume-average turbulent intensity in some particular cases. In most cases, when louvres cause increasing turbulent intensity due to jet deflection inside the room, there is an improvement in air exchange efficiency.
Results show that designing AGCs based on the near-façade flow can improve the performance of the SS1 on average. The average air-exchange efficiency in all three orientations (windward, parallel, leeward) is 11%, 11.9% and 14.43% for plain, louvred, and newly designed AGCs, respectively. This shows a 31.21% improvement on average for the newly designed AGCs compared to the plain opening, while this figure for louvred opening is only 8.2%.
It was found that when AGCs/louvres are the cause of separating the inlet and outlet air flow paths at the opening, they can be the cause of improvement in the performance of the system.
Given the contribution of the newly designed AGCs to the ventilation performance of the building in wind-dominant single-sided natural ventilation, it is noteworthy to mention that these components can affect the windows view, although this effect can be seen as a positive effect due to shading and therefore reduction of cooling load during summer, in addition to increasing the security of the building and providing the secure possibility of night cooling for the enclosed space.
Acknowledgements
The authors wish to acknowledge the Irish Center for High-End Computing (ICHEC) for the provision of computational facilities and support.
Disclosure statement
No potential conflict of interest was reported by the authors.
Correction Statement
This article has been corrected with minor changes. These changes do not impact the academic content of the article.
Additional information
Funding
References
- Argiriou, A. A., Balaras, C. A., & Lykoudis, S. P. (2002). Single-sided ventilation of buildings through shaded large openings. Energy, 27(2), 93–115. https://doi.org/10.1016/S0360-5442(01)00058-5
- Attia, S., Levinson, R., Ndongo, E., Holzer, P., Berk Kazanci, O., Homaei, S., Zhang, C., Olesen, B. W., Qi, D., Hamdy, M., & Heiselberg, P. (2021). Resilient cooling of buildings to protect against heat waves and power outages: Key concepts and definition. Energy and Buildings, 239, 110869. https://doi.org/10.1016/j.enbuild.2021.110869
- Blocken, B. (2015). Computational fluid dynamics for urban physics: Importance, scales, possibilities, limitations and ten tips and tricks towards accurate and reliable simulations. Building and Environment, Elsevier, 91, 219–245. https://doi.org/10.1016/j.buildenv.2015.02.015
- Caciolo, M., Stabat, P., & Marchio, D. (2011). Full scale experimental study of single-sided ventilation: Analysis of stack and wind effects. Energy and Buildings, 43(7), 1765–1773. https://doi.org/10.1016/j.enbuild.2011.03.019
- Caciolo, M., Stabat, P., & Marchio, D. (2012). Numerical simulation of single-sided ventilation using RANS and LES and comparison with full-scale experiments. Building and Environment, 50, 202–213. https://doi.org/10.1016/j.buildenv.2011.10.017
- el Telbany, M. M. M., Mokhtarzadeh-Dehghan, M. R., & Reynolds, A. J. (1985). Single-sided ventilation–Part I. The flow between a cavity and external air stream. Building and Environment, 20(1), 15–24. https://doi.org/10.1016/0360-1323(85)90026-5
- Evola, G., & Popov, V. (2006). Computational analysis of wind driven natural ventilation in buildings. Energy and Buildings, 38(5), 491–501. https://doi.org/10.1016/j.enbuild.2005.08.008
- Franke, J., Hellsten, A., Schlünzen, H., & Carissimo, B. (2007). Best practice guideline for the CFD simulation of flows in the urban environment. COST Action, 732, 1–52.
- Goethals, K., Couckuyt, I., Dhaene, T., & Janssens, A. (2012). Sensitivity of night cooling performance to room/system design: Surrogate models based on CFD. Building and Environment, 58, 23–36. https://doi.org/10.1016/j.buildenv.2012.06.015
- Heiselberg, P., Bjørn, E., & Nielsen, P. v (2002). Impact of open windows on room air flow and thermal comfort. International Journal of Ventilation, Informa UK Limited, Vol, 1(2), 91–100. https://doi.org/10.1080/14733315.2002.11683625
- Jiang, Y., Alexander, D., Jenkins, H., Arthur, R., & Chen, Q. (2003). Natural ventilation in buildings: Measurement in a wind tunnel and numerical simulation with large-eddy simulation. Journal of Wind Engineering and Industrial Aerodynamics, 91(3), 331–353. https://doi.org/10.1016/S0167-6105(02)00380-X
- Kato, S., Kono, R., Hasama, T., Ooka, R., & Takahashi, T. (2006). A wind tunnel experimental analysis of the ventilation characteristics of a room with single-sided opening in uniform flow. International Journal of Ventilation, VEETECH Ltd, 5(1), 171–178. https://doi.org/10.1080/14733315.2006.11683734
- Kosutova, K., van Hooff, T., Vanderwel, C., Blocken, B., & Hensen, J. (2019). Cross-ventilation in a generic isolated building equipped with louvers: Wind-tunnel experiments and CFD simulations. pp. Building and Environment, 154, 263–280. nooctober 2018 https://doi.org/10.1016/j.buildenv.2019.03.019
- Larsen, T. S., & Heiselberg, P. (2008). Single-sided natural ventilation driven by wind pressure and temperature difference. Energy and Buildings, Vol, 40(6), 1031–1040. https://doi.org/10.1016/j.enbuild.2006.07.012
- Martinuzzi, R., & Tropea, C. (1993). The flow around surface-mounted, prismatic obstacles placed in a fully developed channel flow (data bank contribution). Journal of Fluids Engineering, 115(1), 85–92. https://doi.org/10.1115/1.2910118
- Mohamed, M. F., Behnia, M., King, S., & Prasad, D. (2011). The potential of natural ventilation in single-sided ventilated apartment to improve indoor thermal comfort and air quality. ASME 2011 5th International Conference on Energy Sustainability, ES 2011, pp. 71––78.
- Najafi Ziarani, N. J.,Cook, M.,Freidooni, F., &D. O'Sullivan, P. (2023). The role of near-façade flow in wind-dominant single-sided natural ventilation for an isolated three-storey building: An LES study. Building and Environment, 235. 110210 https://doi.org/10.1016/j.buildenv.2023.110230
- Omrani, S., Garcia-Hansen, V., Capra, B. R., & Drogemuller, R. (2017). On the effect of provision of balconies on natural ventilation and thermal comfort in high-rise residential buildings. Building and Environment, 123, 504–516. https://doi.org/10.1016/j.buildenv.2017.07.016
- O'Sullivan, P. D., & Kolokotroni, M. (2017). A field study of wind dominant single sided ventilation through a narrow slotted architectural louvre system. Energy and Buildings, 138, 733–747. https://doi.org/10.1016/j.enbuild.2016.11.025
- Park, J., Choi, J. I., & Rhee, G. H. (2016). Enhanced single-sided ventilation with overhang in buildings. Energies, 9(3), 122. https://doi.org/10.3390/en9030122
- Pokhrel, M. K., Anderson, T. N., & Lie, T. T. (2019). Maintaining thermal comfort of a single-sided naturally ventilated model house by intelligently actuating windows. Proceedings of the 24th CAADRIA Conference - Volume1, Victoria University of Wellington. Wellington, New Zealand, 15–18 Aprl 2019. (pp. 705–714). https://doi.org/10.52842/conf.caadria.2019.1.705
- Pope, S. B. (2000). Turublent flows (12 ed.). Cambridge University Press. https://doi.org/10.1017/CBO9780511840531
- Richards, P. J., & Hoxey, R. P. (1993). Appropriate boundary conditions for computational wind engineering models using the k-ϵ turbulence model. Journal of Wind Engineering and Industrial Aerodynamics, 46-47(C), 145–153. https://doi.org/10.1016/0167-6105(93)90124-7
- Roache, P. J. (1997). Quantification of uncertainty in computational fluid dynamics. Annual Review of Fluid Mechanics, 29(1), 123–160. https://doi.org/10.1146/annurev.fluid.29.1.123
- Sacht, H., Bragança, L., Almeida, M., & Caram, R. (2016). Study of natural ventilation in wind tunnels and influence of the position of ventilation modules and types of grids on a modular façade system. Energy Procedia, Vol, 96, 953–964. Elsevier Ltdhttps://doi.org/10.1016/j.egypro.2016.09.173
- Sandberg, M. (1992). Ventilation effectiveness and purging flow rate–a review. International symposium on room air convection and ventilation effectiveness.
- Schulze, T., Gürlich, D., & Eicker, U. (2018). Performance assessment of controlled natural ventilation for air quality control and passive cooling in existing and new office type buildings. Energy and Buildings, 172, 265–278. https://doi.org/10.1016/j.enbuild.2018.03.023
- Wang, H., & Chen, Q. (. (2015). Modeling of the impact of different window types on single-sided natural ventilation. Energy Procedia. 78, 1549–1555. https://doi.org/10.1016/j.egypro.2015.11.201
- Wang, J., Wang, S., Zhang, T., & Battaglia, F. (2017). Assessment of single-sided natural ventilation driven by buoyancy forces through variable window configurations. Energy and Buildings, Elsevier Ltd, 139, 762–779. https://doi.org/10.1016/j.enbuild.2017.01.070
- Wei, Y., Guo-Qiang, Z., Xiao, W., Jing, L., & San-Xian, X. (2010). Potential model for single-sided naturally ventilated buildings in China. Solar Energy, 84(9), 1595–1600. https://doi.org/10.1016/j.solener.2010.06.011
- Yakhot, V., Orszag, S. A., Thangam, S., Gatski, T. B., & Speziale, C. G. (1992). Development of turbulence models for shear flows by a double expansion technique. Physics of Fluids A: Fluid Dynamics, 4(7), 1510–1520. https://doi.org/10.1063/1.858424
- Zheng, J. W., Tao, Q. H., & Li, L. (2019). Study of influence of shading louvers on wind characteristics around buildings under different wind directions. IOP Conference Series: Earth and Environmental Science, 238, 012031. https://doi.org/10.1088/1755-1315/238/1/012031