Abstract
Biomarkers that describe the severity and progression of COPD and the responses of patients to treatment are a desirable addition to clinical measures of disease. In this review, we describe the current state of knowledge of biomarkers used for the diagnosis, staging and therapeutic response of COPD patients. The nature of these biomarkers is considered in relation to their intended use, and the desirable qualities of such entities are examined. Examples of biased and unbiased discovery platforms for COPD biomarker discovery are given, and the major findings of these studies are discussed. Cutting edge technology used for biomarker discovery, quantitation in biofluids and imaging biomarkers in whole body systems is reviewed.
INTRODUCTION
Much hope has been placed in the discovery of biomarkers to help understand the mechanisms of diseases such as chronic obstructive pulmonary disease (COPD), aid stratification and guide treatment (1–5). It is also hoped that some biomarkers could speed up drug discovery by serving as surrogates of disease that are responsive to novel drugs within a shorter timescale than is the case with current drug trials, in which the main outcome is the spirometric measurement of forced expiratory volume in one second (FEV1). Although studies in patients with COPD have identified several biomarkers, most of these need to be validated and their prognostic value is unclear (6–10). Many of the markers have been identified in blood and, although it is recognised that there are non-pulmonary consequences of COPD, some of which can be viewed as systemic (Citation[10], Citation[11]), biomarkers measured and/or generated in the lungs are likely to be most informative, as they will reflect local changes.
There is no conclusive definition of the nature of biomarkers, but the qualities that they should have are generally agreed since they apply to all diseases (Citation[12], Citation[13]). A common misconception is that biomarkers must be proteins; the Biomarkers Definitions Working Group, seeking to clarify this view, has stated that biomarkers are: “a characteristic that is objectively measured and evaluated as an indicator of normal biologic processes, pathogenic processes, or pharmacologic responses to a therapeutic intervention” (Citation[14]).
Thus, the term also encompasses quantifiable clinical endpoints, genomic data and non-protein chemical entities such as lipids. Biomarkers are, however, generally considered to be substances that can be measured, and, in this respect, protein biomarkers are particularly desirable. Not only are proteins direct effectors of cellular processes, but they also permit measurements relevant to disease in biofluids, where mRNA may be absent. Furthermore, there is often a poor correlation between mRNA levels and protein levels (Citation[15]). The nature of a biomarker depends to some extent upon the purpose for which it is to be used, for example, novel bacterial colonisations are indicative of exacerbation rather than of stable disease (Citation[16]). However, their nature is clearly most strongly influenced by the method by which it is discovered and/or measured.
COPD is, in many ways, a rather special case in that the affected organ is relatively accessible and easy to sample directly rather than indirectly via the peripheral circulation. Aside from clinical endpoints, the single best diagnostic test at present is for alpha-1-antitrypsin deficiency, which is the cause of only a small proportion of cases of COPD. Measures of airways obstruction using spirometry, which is the most common method of diagnosis and is currently used for staging of the disease, can be confounded by co-morbidities such as the co-existence of lung fibrosis and obesity. FEV1, which is the gold standard lung function measurement in COPD diagnosis, is easy to determine and interpret, but correlates poorly with symptom indices such as dyspnoea (Citation[17]). High resolution computed tomography (HRCT) scans can be used to identify the degree of emphysema and presence of tumours, but provide relatively little information on bronchitis, which affects more than half of patients particularly in the early stage of disease. A further weakness of this method is the radiation involved and the expense. Thus, COPD diagnosis depends heavily upon patient history combined with clinical measures of the disease for the vast majority of patients.
There is a recognised need for biomarkers for COPD for several reasons. First, the importance of the disease is well accepted, as it is predicted to be the third major cause of mortality worldwide by 2020 (Citation[18]). Second, while its major trigger, smoking, is declining in the developed world, the incidence of smoking and hence COPD is increasing in the developing world. These locations, which are less likely to be equipped with the specialised equipment necessary for correct diagnosis of the disease, underscore the need for robust and readily assayable biomarkers. Third, existing diagnostic methods are not yet routinely supplemented with further diagnostic information such as that obtained by endobronchoscopic examination and sampling (bronchoalveolar lavage fluid (BALF) or bronchial biopsy/brushing), and/or analysis of inflammatory cell and mediator load in induced sputum. Such methods, while relatively standardised, are not routinely used in the clinic for the diagnosis of COPD. In part, this is because of the lack of biomarkers specific for COPD that can be measured in these samples.
Biomarker discovery methods for COPD
Following a reasonable number of studies searching for biomarkers of COPD, it has become apparent that a number of key factors influence the specificity of the biomarkers discovered. Namely, the clinical criteria used for selection of the patient cohort is critical to enable correct grouping of the cohort and reduce the influence of co-morbidities, and the choice of control groups for such studies (i.e., smokers without disease but with comparable smoking histories are the proper control group in studies of current smokers with COPD) is essential for proper comparisons to account for the widespread effects of smoking itself.
Biased studies
An array of biomarkers have been described for COPD and these have been described in detail in excellent reviews (19–22). Their provenance has generally come about incidentally or by logical extension from the literature. For example, COPD is known to have a systemic inflammatory element, and a number of biomarkers related to systemic inflammation and lung function have now been described with varying specificity for different lung diseases, including CRP (23–25), fibrinogen and IL-6 (Citation[25], Citation[26]). Furthermore, COPD is recognised as having a tissue remodelling element (27–29), either through destruction of the alveolar walls in emphysema, or through thickening of the submucosa in the large and small airways in chronic bronchitis. Consequently, a number of studies have described changes in systemic MMP/TIMP levels (Citation[4], Citation[30]), fibronectin (Citation[24]), collagens (Citation[31]), or SNPs affecting function of cytokines associated with remodelling such as TGF-β (Citation[32], Citation[33]).
Of increasing interest, however, are biomarkers which reflect local pulmonary changes since systemic indicators of disease are inherently more likely to reflect more general responses to ill health or sub-pathological co-morbidities (Citation[34]). Large numbers of biased studies have, therefore, looked for changes in markers produced locally (i.e., the pulmonary environment); sample types such as exhaled breath and its condensate, BALF, induced and spontaneous sputum and even the lung tissue itself have been investigated.
Most of these studies recognise that the discovery of any biomarker is not an end-product in itself since, having identified the target, one has to be able to sample the airways reproducibly and with little invasion to make a biomarker clinically relevant. However, a biomarker discovered by invasive means may turn out to be measurable by non-invasive methods. The principal non-invasive method for sampling the airways remains induced sputum Despite the ease of collection of sputum, the relevance of its derivation (i.e., sampling of the central airways), and the standardisation of methods, remains a challenging medium for the quantification of mediators (Citation[35]).
In an attempt to bridge the gap between biased and unbiased studies, multiplexed analysis of biofluids has been utilised to catalogue changes in disease from a list of potential biomarkers. Limited protein array proteomic analysis of serum biomarkers has been performed and correlated with clinical biomarkers (Citation[7]). Selected biomarkers found to be associated with FEV1 included MMP-9, TNFα and IFN-γ. However, the limitation of such methodologies becomes apparent in that the panel was selected according to prior knowledge and thus cannot identify any novel proteins associated with disease.
A meta-analysis of markers of disease severity in COPD (1) identified only arterial oxygen tension, sputum neutrophils and IL-8, and serum TNF-α and CRP as showing a trend to separating disease stages in COPD. Other measures such as pack years and St. Georges Respiratory Questionnaire separated only health from disease.
Unbiased studies
The greatest potential for novel biomarker discovery lies in unbiased (i.e. true proteomic, genomic, metabolomic and lipidomic) analysis, whether it is targeted at systemic or local biofluids or tissues. This is because, even though a biomarker ideally has biological relevance to disease, in a case such as COPD, the mechanism of susceptibility is currently unknown and therefore useful biomarkers cannot be easily predicted. The limitations of proteomic analysis have so far been related to some degree to restrictions in throughput and the inability to achieve sufficient coverage of the proteome when working with demanding samples, i.e., those with complex cellular make-up (e.g., bronchial biopsies), very dilute samples (e.g., BALF or exhaled breath condensate), samples composed of a few very abundant proteins and relatively low quantities of other potential targets (e.g., serum, plasma) or those with biochemically unfavourable characteristics (e.g., induced sputum). Therefore, initial proteomic studies have focussed on proof of principle analyses, generally through a ‘shotgun’ approach, which generate protein lists describing the known proteome, often with an unvalidated examination of changes in disease in a small cohort of patients. Noel-Georis et al. published a large BALF proteome database determined using 2-dimensional gel electrophoresis (2-DGE) (Citation[36]). A proteomic investigation of changes in the BALF proteome triggered by smoking used a combination of shotgun methods and 2-DGE to extend the known BALF proteome (Citation[37]); however, these authors did not validate any changes observed with smoking using independent tests.
Our group has catalogued the proteome of sputum (Citation[38]) and improved 2-DGE analysis of this biofluid (), while others have described the proteomes of other oropharyngeal compartments, including saliva (39–45), epithelial lining fluid (Citation[46]), nasal lavage fluid (47–51), pulmonary fibroblasts (52–54), pulmonary epithelial cells (55–57), and alveolar macrophages (Citation[58])
Figure 1 A typical 2-DGE profile of human induced sputum from a COPD patient demonstrating the distribution in molecular weight (MW) and isoelectric point (pI) of proteins in this biofluid.
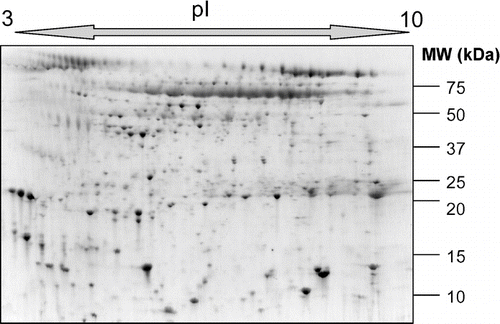
Recently, as technology and thus reproducibility of the analyses has improved, proteomic searches for biomarkers of COPD have been increasingly described. Using surface enhanced laser desorption ionisation-time of flight (SELDI-TOF)-based mass spectrometic analysis, Bozinovski et al. identified serum amyloid protein (SAA) as a novel blood biomarker of acute exacerbations of COPD (Citation[59]) and confirmed their results using ELISA. One caveat of this study is that many of the patients were using steroids to control disease.
The authors found a relationship between SAA and infection, indicating a role in infection/exacerbation rather than purely in COPD itself. This is understandable as SAA is thought to act as an opsonin during microbial infection. SELDI-MS analysis of BALF identified several biomarkers, including Clara Cell Secretory Protein 10 (CCSP10), neutrophil defensins 1 and 2, and calgranulins 1 and 2 (S100A8 and S100A9) as altered in smokers with COPD when compared to asymptomatic smokers (Citation[8]).
Mass spectrometry-based proteomic methods with ever increasing throughput are being developed. One such method utilised Matrix assisted laser desorption ionisation (MALDI) Fourier transform ion cyclotron resonance (FT-ICR) mass spectrometry to identify isoforms of SP-A in BALF-samples from patients with cystic fibrosis, chronic bronchitis and pulmonary alveolar proteinosis (PAP) separated using 2-DGE (Citation[60]). Qualitative differences in SP-A isoforms were observed in patients with PAP compared to the other diseases examined.
SP-A has also been identified in lung tissue as a potential biomarker of COPD using 2D-gel electrophoresis (Citation[61]), results that were confirmed by immunohistochemistry and Western blot. Increases in SP-A were initially observed in severe (GOLD stage 4) patients, but were subsequently shown to also be reflective of changes in milder disease also. Increased SP-A protein expression was also observed in α -1-antitrypsin deficient patients and ex-smokers, indicating a relationship with emphysema and/or bronchitis rather than with smoking.
Importantly, increases in SP-A in sputum from COPD patients were also observed when compared to both non-smokers and healthy smokers, demonstrating the utility of sputum in reflecting changes in the lung tissue with disease.
Casado et al. (Citation[62]) have performed a comparative study of sputum proteomics in health and COPD using liquid chromatography followed by mass spectrometry. They found a number of interesting changes, including a reduction in Clara cell-derived proteins (such as CC10 and Zinc-α 2-glycoprotein), as previously reported (Citation[63]). However, this was not a quantitative study as such, and results were not confirmed by an independent (different) method, so further analysis is required to determine the specificity of these markers.
Gray et al. (Citation[64]) performed probably the most extensive proteomic study of induced sputum samples from patients with cystic fibrosis, bronchiectasis, asthma, COPD and control subjects using SELDI-TOF-MS.
Results were confirmed with either MS/MS or ELISA. Results showed a number of proteins that were altered in COPD, including some found in other proteomic analyses, such as calgranulins a-c, CCSP, lysozyme C, cystatin S and haemoglobin alpha. The most robust of these were the calgranulins, which are neutrophil-derived proteins. Despite not being able to demonstrate a relationship between sputum neutrophilia and calgranulin concentration measured by SELDI, the authors were able to conclude that neutrophils were the likely source. As has been the case with previous proteomic studies using induced sputum, a number of known biomarkers of COPD were not detected as altered in this study. Due to the nature of the investigative platform, this is probably due to technical differences rather than negative results.
Despite improvements in the technology, the SELDI-TOF-MS platform does not yet provide sufficient resolution or reproducibility for use in clinical diagnostics (Citation[65], Citation[66]). As a primary screen, however, it constitutes a useful and relatively high throughput method of proteomic analysis. Lorenz et al. (Citation[67]) have supported the change in calgranulins in airways disease, but also demonstrated their lack of specificity for COPD. summarises the benefits and disadvantages of the current proteomic tools for biomarker discovery in COPD, and demonstrates that no single technology yet combines high throughput with simple quantitation, high resolution and reliable protein identification.
Table 1 Comparison of the relative merits of different methods for biomarker discovery
THE FUTURE OF BIOMARKER DISCOVERY AND QUANTIFICATION
Advances in technology should mean that ultimately, biased and unbiased studies will coalesce as the number of targets (i.e., proportion of the proteome) measurable at one time is increased. Increase in the antibody banks used in microarray studies, particularly for those identifying protein isotypes, means that a significant proportion of proteins present in a proteome can be measured using such methods (Citation[68], Citation[69]), and advances in mass spectrometric design and data handling have led to an increased ability to measure components in a fully quantitative manner using, for example, multiple reaction monitoring, in which ions from selected protein targets are measured using a mass spectrometer in a multiplexed manner (Citation[70], Citation[71]).
There is increasing interest in combining traditional and emerging technologies using proteomic tools to enhance targeting of biomarkers in situ. One example is the use of MALDI MS imaging (Citation[72]) where targets such as existing biomarkers can be definitively identified with reasonable resolution within the tissue itself, either as tissue sections, or, potentially, in vivo. Whole body imaging of compound distribution in whole body tissue sections has already been achieved (Citation[73]) and images reconstructed in 3D (Citation[74]) or combined with MRI (Citation[75]). The advantage of such methods is that they provide definitive protein identifications without the need for intermediate detection reagents such as antibodies, which generally require extensive validation. The quantitative characteristics of such methods remain to be determined.
A further challenge facing proteomic studies, conducted in isolation or in combination with other -omics platforms, is the analysis of very large and complex data. In particular, there is a pressing need for the integration of identified markers into disease mechanism pathways. Such integration with clinical and physiological pathways benefits from a systems biology approach, which provides a powerful way of looking at the whole picture rather than at individual biomarkers (Citation[76], Citation[77]). In the context of a disease with complex mechanisms, this is likely to be very productive.
In summary, proteomic investigations undertaken to find biomarkers of COPD are currently few in number and vary greatly in their approach, both in terms of technology and in the disease criteria used. Thus, comparison of their findings is difficult. The overlap observed, particularly in secretory products of the innate immune system such as calgranulins, however, provides some evidence of the validity of the proteomic approach. Whether proteomic approaches will answer questions related to disease susceptibility, rate of decline of lung function and disease staging, and provides the much needed surrogate markers of therapeutic efficacy in COPD remains to be seen.
Declaration of interest
The authors report no conflict of interest. The authors alone are responsible for the content and writing of the paper.
REFERENCES
- Franciosi L G, Page C P, Celli B R, Cazzola M, Walker M J, Danhof M, Rabe K F, la Pasqua O E. Markers of disease severity in chronic obstructive pulmonary disease. Pulm Pharmacol Ther 2006; 19: 189–199
- Hurst J R, Perera W R, Wilkinson T M, Donaldson G C, Wedzicha J A. Exacerbation of chronic obstructive pulmonary disease: pan-airway and systemic inflammatory indices. Proc Amer Thorac Soc 2006; 3: 481–482
- Wielders P L, Dekhuijzen P N. Disease monitoring in chronic obstructive pulmonary disease: is there a role for biomarkers?. Eur Respir J 1997; 10: 2443–2445
- Aldonyte R, Eriksson S, Piitulainen E, Wallmark A, Janciauskiene S. Analysis of systemic biomarkers in COPD patients. COPD 2004; 1: 155–164
- Casado B, Iadarola P, Luisetti M, Kussmann M. Proteomics-based diagnosis of chronic obstructive pulmonary disease: the hunt for new markers. Expert Rev Proteom 2008; 5: 693–704
- Barnes P J, Stockley R A. COPD: current therapeutic interventions and future approaches. Eur Respir J 2005; 25: 1084–1106
- Pinto-Plata V, Toso J, Lee K, Bilello J, Mullerova H, De S M, Vessey R, Celli B. Use of proteomic patterns of serum biomarkers in patients with chronic obstructive pulmonary disease: correlation with clinical parameters. Proc Amer Thorac Soc 2006; 3: 465–466
- Merkel D, Rist W, Seither P, Weith A, Lenter M C. Proteomic study of human bronchoalveolar lavage fluids from smokers with chronic obstructive pulmonary disease by combining surface-enhanced laser desorption/ionization-mass spectrometry profiling with mass spectrometric protein identification. Proteomics 2005; 5: 2972–2980
- Tzortzaki E G, Lambiri I, Vlachaki E, Siafakas N M. Biomarkers in COPD. Curr Med Chem 2007; 14: 1037–1048
- Bowler R P, Ellison M C, Reisdorph N. Proteomics in pulmonary medicine. Chest 2006; 130: 567–574
- Ashitani J, Mukae H, Arimura Y, Matsukura S. Elevated plasma procoagulant and fibrinolytic markers in patients with chronic obstructive pulmonary disease. Intern Med 2002; 41: 181–185
- Sunderland T, Gur R E, Arnold S E. The use of biomarkers in the elderly: current and future challenges. Biol Psych 2005; 58: 272–276
- Lakhan S E. Schizophrenia proteomics: biomarkers on the path to laboratory medicine?. Diagn Pathol 2006; 1: 11
- Biomarkers Definitions Working G Biomarkers and surrogate endpoints: preferred definitions and conceptual framework. Clin Pharmacol Ther 2001; 69: 89–95
- Pascal L E, True L D, Campbell D S, Deutsch E W, Risk M, Coleman I M, Eichner L J, Nelson P S, Liu A Y. Correlation of mRNA and protein levels: cell type-specific gene expression of cluster designation antigens in the prostate. BMC Genom 2008; 9: 246
- Sethi S, Murphy T F. Bacterial infection in chronic obstructive pulmonary disease in 2000: a state-of-the-art review. Clin Microbiol Rev 2001; 14: 336–363
- Nishimura K, Izumi T, Tsukino M, Oga T. Dyspnea is a better predictor of 5-year survival than airway obstruction in patients with COPD. Chest 2002; 121: 1434–1440
- Murray C J, Lopez A D. Alternative projections of mortality and disability by cause 1990–2020: Global Burden of Disease Study. Lancet 1997; 349: 1498–1504
- Kharitonov S A, Barnes P J. Biomarkers of some pulmonary diseases in exhaled breath. Biomarkers 2002; 7: 1–32
- Jeffery P K, Laitinen A, Venge P. Biopsy markers of airway inflammation and remodelling. Respir Med 2000; 94(Suppl F)S9–15
- Jones P W, Agusti A G. Outcomes and markers in the assessment of chronic obstructive pulmonary disease. Eur Respir J 2006; 27: 822–832
- Cazzola M, MacNee W, Martinez F J, Rabe K F, Franciosi L G, Barnes P J, Brusasco V, Burge P S, Calverley P M, Celli B R, et al. Outcomes for COPD pharmacological trials: from lung function to biomarkers. Eur Respir J 2008; 31: 416–469
- Broekhuizen R, Wouters E F, Creutzberg E C, Schols A M. Raised CRP levels mark metabolic and functional impairment in advanced COPD. Thorax 2006; 61: 17–22
- Man S F, Xing L, Connett J E, Anthonisen N R, Wise R A, Tashkin D P, Zhang X, Vessey R, Walker T G, Celli B R, et al. Circulating fibronectin to C-reactive protein ratio and mortality: a biomarker in COPD?. Eur Respir J 2008; 32: 1451–1457
- Fogarty A W, Jones S, Britton J R, Lewis S A, McKeever T M. Systemic inflammation and decline in lung function in a general population: a prospective study. Thorax 2007; 62: 515–520
- Donaldson G C, Seemungal T A, Patel I S, Bhowmik A, Wilkinson T M, Hurst J R, Maccallum P K, Wedzicha J A. Airway and systemic inflammation and decline in lung function in patients with COPD. Chest 2005; 128: 1995–2004
- Roth M. Pathogenesis of COPD. Part III. Inflammation in COPD. Int J Tuberc Lung Dis 2008; 12: 375–380
- Kranenburg A R, Willems-Widyastuti A, Moori W J, Sterk P J, Alagappan V K, de Boer W I, Sharma H S. Enhanced bronchial expression of extracellular matrix proteins in chronic obstructive pulmonary disease. Am J Clin Pathol 2006; 126: 725–735
- Hogg J C, Chu F, Utokaparch S, Woods R, Elliott W M, Buzatu L, Cherniack R M, Rogers R M, Sciurba F C, Coxson H O, et al. The nature of small-airway obstruction in chronic obstructive pulmonary disease. N Engl J Med 2004; 350: 2645–2653
- Higashimoto Y, Yamagata Y, Iwata T, Okada M, Ishiguchi T, Sato H, Masuda M, Itoh H. Increased serum concentrations of tissue inhibitor of metalloproteinase-1 in COPD patients. Eur Respir J 2005; 25: 885–890
- Szoltysik E, Kucharz E J. Serum markers of collagen metabolism in patients with diseases of the respiratory system. Rom J Intern Med 1993; 31: 119–122
- Celedon J C, Lange C, Raby B A, Litonjua A A, Palmer L J, Demeo D L, Reilly J J, Kwiatkowski D J, Chapman H A, Laird N, et al. The transforming growth factor-beta1 (TGFB1) gene is associated with chronic obstructive pulmonary disease (COPD). Hum Mol Genet 2004; 13: 1649–1656
- Ito M, Hanaoka M, Droma Y, Hatayama O, Sato E, Katsuyama Y, Fujimoto K, Ota M. The association of transforming growth factor beta 1 gene polymorphisms with the emphysema phenotype of COPD in Japanese. Intern Med 2008; 47: 1387–1394
- Sin D D, Man S F. Biomarkers in COPD: are we there yet?. Chest 2008; 133: 1296–1298
- Erin E M, Jenkins G R, Kon O M, Zacharasiewicz A S, Nicholson G C, Neighbour H, Tennant R C, Tan A J, Leaker B R, Bush A, et al. Optimized dialysis and protease inhibition of sputum dithiothreitol supernatants. Am J Respir Crit Care Med 2008; 177: 132–141
- Noel-Georis I, Bernard A, Falmagne P, Wattiez R. Database of bronchoalveolar lavage fluid proteins. J Chromatogr B Analyt Technol Biomed Life Sci 2002; 771: 221–236
- Plymoth A, Yang Z, Lofdahl C G, Ekberg-Jansson A, Dahlback M, Fehniger T E, Marko-Varga G, Hancock W S. Rapid proteome analysis of bronchoalveolar lavage samples of lifelong smokers and never-smokers by micro-scale liquid chromatography and mass spectrometry. Clin Chem 2006; 52: 671–679
- Nicholas B, Skipp P, Mould R, Rennard S, Davies D E, O'Connor C D, Djukanovic R. Shotgun proteomic analysis of human-induced sputum. Proteomics 2006; 6: 4390–4401
- Millea K M, Krull I S, Chakraborty A B, Gebler J C, Berger S J. Comparative profiling of human saliva by intact protein LC/ESI-TOF mass spectrometry. Biochim Biophys Acta 2007; 1774: 897–906
- Hu S, Xie Y, Ramachandran P, Ogorzalek Loo R R, Li Y, Loo J A, Wong D T. Large-scale identification of proteins in human salivary proteome by liquid chromatography/mass spectrometry and two-dimensional gel electrophoresis-mass spectrometry. Proteomics 2005; 5: 1714–1728
- Hu S, Yu T, Xie Y, Yang Y, Li Y, Zhou X, Tsung S, Loo R R, Loo J R, Wong D T. Discovery of oral fluid biomarkers for human oral cancer by mass spectrometry. Cancer Genomics Proteomics 2007; 4: 55–64
- Hardt M, Thomas L R, Dixon S E, Newport G, Agabian N, Prakobphol A, Hall S C, Witkowska H E, Fisher S J. Toward defining the human parotid gland salivary proteome and peptidome: identification and characterization using 2D SDS-PAGE, ultrafiltration, HPLC, and mass spectrometry. Biochemistry 2005; 44: 2885–2899
- Huang C M. Comparative proteomic analysis of human whole saliva. Arch Oral Biol 2004; 49: 951–962
- Xie H, Rhodus N L, Griffin R J, Carlis J V, Griffin T J. A catalogue of human saliva proteins identified by free flow electrophoresis-based peptide separation and tandem mass spectrometry. Mol Cell Proteomics 2005; 4: 1826–1830
- Amado F M, Vitorino R M, Domingues P M, Lobo M J, Duarte J A. Analysis of the human saliva proteome. Expert Rev Proteomics 2005; 2: 521–539
- Kipnis E, Hansen K, Sawa T, Moriyama K, Zurawel A, Ishizaka A, Wiener-Kronish J. Proteomic analysis of undiluted lung epithelial lining fluid. Chest 2008; 134: 338–345
- Debat H, Eloit C, Blon F, Sarazin B, Henry C, Huet J C, Trotier D, Pernollet J C. Identification of human olfactory cleft mucus proteins using proteomic analysis. J Proteome Res 2007; 6: 1985–1996
- Ghafouri B, Irander K, Lindbom J, Tagesson C, Lindahl M. Comparative proteomics of nasal fluid in seasonal allergic rhinitis. J Proteome Res 2006; 5: 330–338
- Casado B, Pannell L K, Viglio S, Iadarola P, Baraniuk J N. Analysis of the sinusitis nasal lavage fluid proteome using capillary liquid chromatography interfaced to electrospray ionization-quadrupole time of flight-tandem mass spectrometry. Electrophoresis 2004; 25: 1386–1393
- Casado B, Pannell L K, Iadarola P, Baraniuk J N. Identification of human nasal mucous proteins using proteomics. Proteomics 2005; 5: 2949–2959
- Bryborn M, Adner M, Cardell L O. Psoriasin, one of several new proteins identified in nasal lavage fluid from allergic and non-allergic individuals using 2-dimensional gel electrophoresis and mass spectrometry. Respir Res 2005; 6: 118
- Malmstrom J, Larsen K, Malmstrom L, Tufvesson E, Parker K, Marchese J, Williamson B, Patterson D, Martin S, Juhasz P, et al. Nanocapillary liquid chromatography interfaced to tandem matrix-assisted laser desorption/ionization and electrospray ionization-mass spectrometry: mapping the nuclear proteome of human fibroblasts. Electrophoresis 2003; 24: 3806–3814
- Malmstrom J, Larsen K, Malmstrom L, Tufvesson E, Parker K, Marchese J, Williamson B, Hattan S, Patterson D, Martin S, et al. Proteome annotations and identifications of the human pulmonary fibroblast. J Proteome Res 2004; 3: 525–537
- Bogatkevich G S, Ludwicka-Bradley A, Singleton C B, Bethard J R, Silver R M. Proteomic analysis of CTGF-activated lung fibroblasts: identification of IQGAP1 as a key player in lung fibroblast migration. Am J Physiol Lung Cell Mol Physiol 2008; 295: L603–L611
- Kelsen S G, Duan X, Ji R, Perez O, Liu C, Merali S. Cigarette smoke induces an unfolded protein response in the human lung: a proteomic approach. Am J Respir Cell Mol Biol 2008; 38: 541–550
- Li C, Zhan X, Li M, Wu X, Li F, Li J, Xiao Z, Chen Z, Feng X, Chen P, et al. Proteomic comparison of two-dimensional gel electrophoresis profiles from human lung squamous carcinoma and normal bronchial epithelial tissues. Genomics Proteomics Bioinformatics 2003; 1: 58–67
- Zhao H, Adler K B, Bai C, Tang F, Wang X. Epithelial proteomics in multiple organs and tissues: similarities and variations between cells, organs, and diseases. J Proteome Res 2006; 5: 743–755
- Jin M, Opalek J M, Marsh C B, Wu H M. Proteome comparison of alveolar macrophages with monocytes reveals distinct protein characteristics. Am J Respir Cell Mol Biol 2004; 31: 322–329
- Bozinovski S, Hutchinson A, Thompson M, Macgregor L, Black J, Giannakis E, Karlsson A S, Silvestrini R, Smallwood D, Vlahos R, et al. Serum amyloid a is a biomarker of acute exacerbations of chronic obstructive pulmonary disease. Am J Respir Crit Care Med 2008; 177: 269–278
- Bai Y, Galetskiy D, Damoc E, Paschen C, Liu Z, Griese M, Liu S, Przybylski M. High resolution mass spectrometric alveolar proteomics: identification of surfactant protein SP-A and SP-D modifications in proteinosis and cystic fibrosis patients. Proteomics 2004; 4: 2300–2309
- Ohlmeier S, Vuolanto M, Toljamo T, Vuopala K, Salmenkivi K, Myllarniemi M, Kinnula V L. Proteomics of human lung tissue identifies surfactant protein A as a marker of chronic obstructive pulmonary disease. J Proteome Res 2008; 7: 5125–5132
- Casado B, Iadarola P, Pannell L K, Luisetti M, Corsico A, Ansaldo E, Ferrarotti I, Boschetto P, Baraniuk J N. Protein expression in sputum of smokers and chronic obstructive pulmonary disease patients: a pilot study by CapLC-ESI-Q-TOF. J Proteome Res 2007; 6: 4615–4623
- Reynolds S D, Reynolds P R, Pryhuber G S, Finder J D, Stripp B R. Secretoglobins SCGB3A1 and SCGB3A2 define secretory cell subsets in mouse and human airways. Am J Respir Crit Care Med 2002; 166: 1498–1509
- Gray R D, MacGregor G, Noble D, Imrie M, Dewar M, Boyd A C, Innes J A, Porteous D J, Greening A P. Sputum proteomics in inflammatory and suppurative respiratory diseases. Am J Respir Crit Care Med 2008; 178: 444–452
- Ekblad L, Baldetorp B, Ferno M, Olsson H, Bratt C. In-source decay causes artifacts in SELDI-TOF MS spectra. J Proteome Res 2007; 6: 1609–1614
- Semmes O J, Feng Z, Adam B L, Banez L L, Bigbee W L, Campos D, Cazares L H, Chan D W, Grizzle W E, Izbicka E, et al. Evaluation of serum protein profiling by surface-enhanced laser desorption/ionization time-of-flight mass spectrometry for the detection of prostate cancer: I. Assessment of platform reproducibility. Clin Chem 2005; 51: 102–112
- Lorenz E, Muhlebach M S, Tessier P A, Alexis N E, Duncan H R, Seeds M C, Peden D B, Meredith W. Different expression ratio of S100A8/A9 and S100A12 in acute and chronic lung diseases. Respir Med 2008; 102: 567–573
- Uhlen M. Affinity as a tool in life science. Biotechniques 2008; 44: 649–654
- Hober S, Uhlen M. Human protein atlas and the use of microarray technologies. Curr Opin Biotechnol 2008; 19: 30–35
- Kuhn E, Wu J, Karl J, Liao H, Zolg W, Guild B. Quantification of C-reactive protein in the serum of patients with rheumatoid arthritis using multiple reaction monitoring mass spectrometry and 13C-labeled peptide standards. Proteomics 2004; 4: 1175–1186
- Anderson L, Hunter C L. Quantitative mass spectrometric multiple reaction monitoring assays for major plasma proteins. Mol Cell Proteomics 2006; 5: 573–588
- McDonnell L A, Heeren R M. Imaging mass spectrometry. Mass Spectrom Rev 2007; 26: 606–643
- Khatib-Shahidi S, Andersson M, Herman J L, Gillespie T A, Caprioli R M. Direct molecular analysis of whole-body animal tissue sections by imaging MALDI mass spectrometry. Anal Chem 2006; 78: 6448–6456
- Andersson M, Groseclose M R, Deutch A Y, Caprioli R M. Imaging mass spectrometry of proteins and peptides: 3D volume reconstruction. Nat Meth 2008; 5: 101–108
- Sinha T K, Khatib-Shahidi S, Yankeelov T E, Mapara K, Ehtesham M, Cornett D S, Dawant B M, Caprioli R M, Gore J C. Integrating spatially resolved three-dimensional MALDI IMS with in vivo magnetic resonance imaging. Nat Meth 2008; 5: 57–59
- Bachi A, Bonaldi T. Quantitative proteomics as a new piece of the systems biology puzzle. J Proteomics 2008; 71: 357–367
- Preisinger C, von K A, Matallanas D, Kolch W. Proteomics and phosphoproteomics for the mapping of cellular signalling networks. Proteomics 2008; 8: 4402–4415