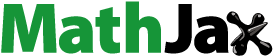
ABSTRACT
In this work, rice straw fibers were modified using NaOH, KBM-403, and tetraethyl orthosilicate (TEOS), and the changes in the surface groups of the straw fibers before and after the modification were analyzed by infrared testing. Straw/polylactic composite were prepared by hot pressing, and the effects of the modifiers used on the properties of these blends were studied by conducting mechanical and thermal performance tests. The results showed that the tensile strength of the straw fiber composites modified by NaOH and KBM-403 increased by 20% and 21.2%, respectively, compared with that of the unmodified composite, whereas the tensile strength of the TEOS-modified fiber did not increase significantly. The flexural strengths of the composites increased by 10.2%, 11.1%, and 13.2%. The impact strength increased by 38.5%, 7.6%, and 7.6%. The results of thermal analysis show that initial and maximum thermal decomposition temperatures of the composite materials increased, indicating that the modified composite materials had a higher thermal stability. These blends are a kind of potential material for structural applications such as interiors, drywell and partitions for furniture.
摘要
本工作使用NaOH、KBM-403和正硅酸四乙酯(TEOS)对稻草纤维进行了改性,并通过红外测试分析了改性前后稻草纤维表面基团的变化. 采用热压法制备了秸秆/聚乳酸复合材料,并通过力学性能和热性能测试,研究了改性剂对共混物性能的影响. 结果表明,NaOH和KBM-403改性的稻草纤维复合材料的拉伸强度分别比未改性的复合材料提高了20%和21.2%,而TEOS改性的纤维的拉伸强度没有显著提高. 复合材料的弯曲强度分别提高了10.2%、11.1%和13.2%. 热分析结果表明,复合材料的初始热分解温度和最高热分解温度分别提高了38.5%、7.6%和7.6%,表明改性后的复合材料具有更高的热稳定性. 这些混合物是一种潜在的结构应用材料,如室内、驾驶舱和家具隔板.
Introduction
In recent years, with the reduction in the use of nonrenewable resources, such as oil, and with increased environmental awareness, the research and manufacturing of composites synthesized from renewable and biodegradable resources have gained attention (Ilyas et al. Citation2022; Mayilswamy and Kandasubramanian Citation2022; Rajeshkumar et al. Citation2021; Ramasubbu and Madasamy Citation2022). Green composite materials of plant fibers (e.g., sisal and bamboo fibers) conform to the concepts of sustainable development, energy conservation, and environmental protection (de Castro et al. Citation2021; Ramasubbu and Madasamy Citation2022). They have broad application prospects, such as in automobiles and furniture (Mahmud et al. Citation2021). Unlike sisal and bamboo fibers, straws are a by-product of agricultural processing. Currently, straws are mainly used in “coal-like” granulation production of straw biomass fuel, preparation of activated carbon, and papermaking (Lan et al. Citation2020; Sun et al. Citation2020; Zhang et al. Citation2020). In recent years, in industrial production, the difficulty in straw bonding has mainly been solved using expensive high-strength adhesives (such as isocyanate adhesives), which are used in the production of chipboards and other types of boards (Sam-Brew and Smith Citation2017; Sun et al. Citation2019). This not only improves the added value of straws, but also promotes sustainable practice (Sun et al. Citation2019). However, problems include high production costs and complex production processes. To reduce the production cost of particle boards, straw composite materials have been prepared using straw fibers (as fillers) and polymer materials through hot pressing and injection molding processes.
As straw contains cellulose, hemicellulose, and lignin, the surface of a straw fiber contains polar groups, such as hydroxyl, which makes the surface polar. The matrix used to prepare a straw composite is mostly a hydrophobic polymer. If the straw and hydrophobic polymer are directly made into a composite, their interface compatibility will be poor (Chen et al. Citation2021). Therefore, the straw should be modified to improve the interfacial compatibility between the straw fiber and polymer. Straws can be generally modified via physical, chemical, and biological processes. Physical modification includes ultrasonic treatment, microwave treatment, and low-temperature plasma treatment. In this approach, a mechanical force or radiation is applied to destroy the microstructure of the crop straw surface, increase the specific surface area, and expose its polar surface layer, so as to improve the wettability and polarity of the straw surface. Hou et al. (Citation2014) treated a cotton straw with steam flash explosion and alkali solution, and this treatment was found to impart the cotton straw/polypropylene (PP) composite with the best mechanical properties and dimensional stability under water immersion conditions. Chemical treatment mainly improves the compatibility of straw with adhesives by breaking down nonpolar substances such as waxy substances on the straw surface and dissolving hemicellulose and lignin through the action of acid (e.g., sulfuric acid, acetic acid, oxalic acid) or alkali (e.g., sodium hydroxide) solutions, making the surface rough. Li et al. (Citation2022) investigated the interfacial compatibility of wheat straw/polylactic acid (WS/PLA) composites with sodium hydroxide was modified, and the results showed that the tensile and flexural strengths of the modified composites were improved. In the biological treatment method, different types of enzyme solutions or micro-organisms are used to remove the corresponding chemical components on the surface of the crop straw, thus effectively enhancing the surface roughness of the crop straw, improving its surface polarity, and increasing the bonding performance between the straw and the adhesive. Yang et al. (Citation2017) treated wheat straw fiber with laccase and successfully prepared an adhesive-free fiberboard. Poonia et al. (Citation2022) found alkali and enzyme treatments resulted in a significant 50% reduction in fiber length and fiber diameter of rice straw, which increased the aspect ratio, thus contributing to the enhancement of mechanical properties of straw composites.
Domestic and foreign scholars have conducted relevant research on modifying plant fibers using the sol-gel method. For example, Zulkifli et al. (Citation2018) prepared a sol-gel-silicified kenaf bast fiber (KBF)-reinforced polypropylene (PP-KBF-S) composite using the sol – gel method to modify KBF. The results showed that the sol-gel modification of KBF reduced the void content in the PP-KBF-S composite and improved the mechanical properties and thermal stability of KBF. Sun and Wu (Citation2019) modified sisal fiber using the sol-gel method and prepared composites with better mechanical and thermal properties.
Currently, there are few studies on the modification of straw fibers using the sol-gel method. In this work, sodium hydroxide, silane coupling agent KBM-403, and tetraethyl orthosilicate were used to modify straw fibers, and the effects of the three different modifiers on the properties of the prepared straw fiber composites were studied.
Experimental
Materials
The PLA (4032D, 1.24 g/cm3) was supplied by Nature Works Company, USA. Straw fiber length was in the range of 5–20 mm, purchased from Anhui Shangyuan household materials Co., Ltd. The silane coupling agent was KBM-403, provided by Shin-Etsu, Japan. NaOH (96%), tetraethyl orthosilicate (TEOS), and anhydrous ethanol were purchased from Shanghai Macklin Biochemical Technology Co., Ltd.
Surface pretreatment of straw fiber
NaOH treatment
The straw fiber was rinsed 4–5 times with clean water to remove the impurities on its surface and was then placed in an oven for drying at 70°C for 12 h. We prepared 8% sodium hydroxide solution by mass fraction and added the straw fiber into this solution and soaked it for 12 h. It was then removed and washed repeatedly with deionized water to a neutral pH, and finally placed in the oven for 12 h.
Silane coupling agent treatment
2% silane coupling agent solution (absolute ethanol-to-deionized water ratio of 8:2) was prepared, added the straw fiber into this solution, and kept it for 1 h and later removed. After the absolute ethanol on the surface volatilized, we placed it in the oven for 12 h.
TEOS treatment
2% TEOS solution was prepared, hydrolyzed it under magnetic stirring for 12 h, added an appropriate amount of ammonia water to the solution to bring its pH to a range of 6–7, and added the straw fiber when it formed a sol and then a gel. Finally, we placed it in the oven for drying for 72 h. TEOS treatment process is shown in .
Preparation of straw fiber/PLA composite
First, the PLA masterbatch was placed in a hot press maintained at 180°C, preheated for 1 min, pressurized to 4 MPa and kept for 1 min, and finally removed to complete the preparation of the PLA film. The film was cut, and the mass ratio of the untreated, NaOH-treated, silane coupling agent-treated, and sol – gel-treated straw fiber to the polylactic acid film was 2:8. The film was preheated for 5 min at 180°C by lamination and then pressurized to 4 MPa for 5 min. After natural cooling, it was removed from the hot press to complete the preparation of the composite materials. The preparation process of straw fiber/PLA composite is shown in .
Fourier-transform infrared (FTIR) spectroscopy test
The FTIR spectroscopy was performed using a FTIR spectrometer (Thermo Scientific Nicolet iS5, USA). After the dry, the samples and the dried potassium bromide powder are milled thoroughly in a quartz dish so that the samples can be mixed uniformly. The sample was tested in the scanning wave number range of 400–4000 cm−1 and at a resolution of 4 cm−1, the number of scans was 32.
Fracture SEM analysis of composites
The cross-sectional morphology of the samples after the impacts were observed using a bench-top scanning electron microscope (S-4800, Hitachi, Japan). The samples were scanned and observed by firing gun at an acceleration voltage of 5.0 kV after 20 s of gold spraying by ion sputtering.
Mechanical properties test
Tensile properties
The tensile test was conducted on a universal tensile testing machine (WCW-20, China) in accordance with the ISO 527–4:1997 standard. The composite samples were cut into dimensions of 250 mm × 25 mm × 4 mm and tested at a tensile speed of 2 mm/min.
Flexural test
The flexural test was performed on an electronic universal material testing machine (WCW-20, China). The samples were cut to 80 mm × 10 mm × 4 mm with a cutter according to ISO-178-2010 standard and measured at 2 mm/min.
Impact test
A 7.5 J simply supported beam impact testing machine (XJJ-50S) was used to conduct a notch-free impact strength test. The sample was cut into dimensions of 80 mm × 10 mm × 4 mm in accordance with the standard ISO 179-1-2006.
Differential Scanning Calorimetry (DSC)
A DSC analyzer (DSC Q2000, TA Instruments, USA) was applied to investigate the thermal history over a temperature range of 30–200°C at 10°C/min under a 20 ml/min N2 flow. The glass-transition temperature (Tg), crystallization temperature (Tc), melting temperature (Tm), crystallization enthalpy (ΔHc), and melting enthalpy (ΔHm) were determined from the second heating. By considering the enthalpy of fusion (ΔHo) of a PLA crystal of infinite size as 93.6 J/g, and χPLA as the PLA content, the degree of crystallinity (χc) can be calculated as follows:
Thermogravimetric Analysis (TGA)
A thermogravimetric analyzer (TGA 550, TA Instruments, USA) was used to determine the thermal stability of the unmodified and modified composites. The test was performed in an N2 environment, in the temperature range of 30–800°C, at a heating rate of 10°C/min, and at an N2 flow rate of 50 ml/min.
Energy Dispersive Spectroscopy (EDS)
Energy dispersive spectroscopy (EDS) was performed on a field emission scanning electron microscope (Hitachi, Tokyo, Japan) to determine the elements and contents on the surface of the unmodified and TEOS-modified straw fibers.
Results and discussions
Treated straw morphology and EDS results
The SEM of the four chemically modified straw fibers is shown in . After the NaOH treatment, impurities such as waxy substances and pectin present on the surface of the straw fibers and lignin and hemicellulose in the fibers were removed, so the surface of the straw fibers became rough (Reddy et al. Citation2019); a water film appeared on the surface of the straw fibers modified by the silane coupling agent, mainly due to the hydrolysis of the coupling agent into silanol, which later combined with the straw fiber surface -OH to form. The surface of straw fiber modified by TEOS has a granular layer, which is due to the formation of monosilicic acid and alcohol after hydrolysis of TEOS, and the formation of SiO2 nanoparticles after the condensation reaction between silicic acid. The above three modifications helped to increase the interfacial compatibility between straw fibers and PLA, NaOH treatment and TEOS treatment increased the roughness of the straw fiber surface, while all three modifications reduced the polarity of the straw fiber surface, which is consistent with the FTIR findings in . The surface elements of the TEOS-modified straw fiber were tested by EDS; presents the element contents. As shown, the Si content on the surface of the unmodified straw fiber is 2.75% and that on the surface of the straw fiber modified by TEOS is increased from 2.75% to 13.77%. The Si content on the unmodified straw fiber surface is mainly due to the ash content in the straw fiber. The increase in the Si content after TEOS modification is due to the formation of nanoSiO2 on the surface of the straw fibers.
Figure 3. Surface morphologies of the straw fiber before and after modification and energy dispersive spectroscopy.

Table 1. Surface elements and content of straw fibers before and after modification.
FTIR analysis modification results
shows the infrared spectra before and after the surface modification of the straw fiber. The peak at 3300 cm−1 can be attributed to the absorption vibration of -OH (Reddy et al. Citation2019). The -OH absorption vibration peak of the unmodified straw fiber is stronger. After the alkali treatment of the straw fiber, the -OH absorption vibration peak is weakened, indicating a decrease in the polarity of the straw fiber.
Near Citation1732 cm−1, there is a C=O stretching vibration peak attributed to the acetyl and carboxyl groups on hemicellulose (Guo et al. Citation2019). The intensity of this peak is smaller for the alkali-treated straw fibers. In addition, the peak associated with the vibrational properties of the lignin benzene ring skeleton was significantly weaker around 1507 cm−1. The C-O stretching vibrational peaks of lignin and hemicellulose near Citation1251 and 1046 cm−1 were also significantly weaker, indicating that the lignin and hemicellulose contents in the straw fibers obtained by alkali treatment were reduced. In the spectrum of KBM-403-modified straw fiber, the Si-O peak appeared at 800 cm−1, mainly because the silane coupling agent KBM-403 hydrolyzed into silanol, and the Si-O-C peak appeared at 1000 cm−1, while the absorption peak intensity of -OH at 3300 cm−1 of straw fiber decreased. This is due to the dehydration reaction of silanol with -OH on the straw fiber surface to form Si-O-C, which also confirms the successful attachment of silane coupling agent on the straw fiber surface (Chen et al. Citation2021; Gogoi and Tyagi Citation2019). The straw fiber modified by TEOS has a Si-O peak at 800 cm−1, and the vibration peak at 1000 cm−1 is stronger than that of the unmodified straw fiber. This is because TEOS generates Si-O with the -OH on the straw fiber surface after hydrolysis, and the vibration peak at 1000 cm−1 is enhanced because of the condensation reaction between the mono-silicic acids to form Si-O-Si (Zulkifli et al. Citation2018).
Mechanical properties
shows the tensile strength and modulus of the straw fiber/PLA composite. The tensile strength of the unmodified straw fiber/PLA composite was 8.5 MPa. The tensile strengths of the composites after alkali treatment, silane coupling agent KBM-403 treatment, and TEOS modification were 10.3 MPa, 10.3 MPa, and 8.6 MPa, respectively. Compared with the unmodified composite, the tensile strength increased by 20%and 21.2%, whereas the tensile strength of the TEOS-modified straw fiber did not increase significantly, this is consistent with previous reports (Sun and Wu Citation2019). shows that the modulus of the unmodified composite is higher than those of the composites subjected to the NaOH and KBM-403 treatments, and the tensile modulus of the TEOS-modified composite is higher than that of the unmodified composite. show the bending strength and modulus of the straw fiber/PLA composite. The bending strength of the unmodified straw fiber/PLA composite was 23.5 MPa. The bending strengths of the composites after alkali treatment, KBM-403 treatment, and TEOS modification treatment were 25.9 MPa, 26.1 MPa, and 26.6 MPa, respectively. Compared with the unmodified composite, the bending strength increased by 10.2%, 11.1%, and 13.2%. shows the flexural modulus of the straw/PLA composites, it can be seen that the flexural modulus of the modified composites all increased. show the impact strength of those blends. The impact strength of the unmodified straw fiber/PLA composite was 1.3 kJ/m2. The impact strengths of the composites treated with NaOH, silane coupling agent KBM-403, and TEOS were 1.8, 1.4, and 1.4 kJ/m2, respectively. Compared with the unmodified composite, the impact strength of the unmodified straw fiber/PLA composite increased by 38.5%, 7.6%, and 7.6%, respectively. The increased mechanical properties of the composites were attributed to the NaOH treatment which imparted a rough fiber surface and increased the mechanical locking with the PLA matrix. KBM-403 treatment formed a Si-O bond with -OH on the straw fiber surface, which reduced the polarity of the straw fiber surface and formed a “molecular layer” between the straw fiber and PLA, enhancing the interfacial compatibility between the straw fiber and PLA matrix. This is consistent with the interfacial compatibility found in FTIR. The reason for the formation of nano-SiO2 on the straw fiber surface in the case of the TEOS modification is that the force between SiO2 and straw fiber was weak, and nanoSiO2 easily fell off from the straw fiber during stretching. On the other hand, the increase in the mechanical properties of the composites was related to the increase in the crystallinity of the composites. From the DSC data, it was found that the crystallinity increased in the modified composites due to the NaOH treatment, KBM-403 treatment and TEOS modification treatment, which provided “heterogeneous nucleation” sites for PLA during the cooling crystallization process. The tensile modulus of the composites decreased with NaOH and KBM-403 treatment, and increased with TEOS treatment. This is because the NaOH treatment helped remove hemicellulose and lignin from the straw fiber, which reduced the modulus of the straw fiber. The reduction in the tensile modulus of the KBM-403-treated composite can be attributed to the reduction in the tensile modulus of the straw fiber in the presence of a flexible molecular layer between the straw fiber and PLA. The increase in the tensile modulus of the TEOS-modified composite can be attributed to the increase in the tensile modulus of the composite owing to the fact that nano-SiO2 is a rigid material. The bending modulus of both modified composites increased, mainly because the sodium hydroxide treatment increased the mechanical locking between straw fiber and PLA matrix, KBM-403 treatment made a flexible molecular layer exist between straw fiber and PLA matrix as a common external force resistance, the increase of bending modulus of TEOS modified composites was due to the introduction of rigid nano-SiO2 in the system, nano-SiO2 can fill into the grooves of straw fibers to repair the defects in them, and when the bending direction receives external forces, nano-SiO2 can evenly disperse the external forces, avoiding the stress concentration of external forces at the defects, thus enhancing the ability of the composite to resist external forces in the bending direction enhanced, thus making the bending modulus of the composite increase (Zuo et al. Citation2020).
DSC and thermogravimetric analysis
displays the glass transition temperature (Tg), melting temperature (Tm), and crystallinity of the corresponding composites were recorded by DSC, including data related to ΔHc, melting enthalpy (ΔHm), and degree of crystallization (Χc). The data are summarized in . As an additive, the straw fiber before and after the modification affects the thermal performance of the composite. shows that, with the addition of the straw fiber, the Tg of the composite increases slightly; nevertheless, both Tg and Tm remain at approximately 60°C. Compared with the unmodified composite, Tg and Tm change little; the reason is that an increase in crystallinity increases the heat required to melt in the crystalline region, making the composite Tg and Tm increase. However, the crystallinity is related to the mechanical properties of the composites. The increase of crystallinity is beneficial to the increase of mechanical properties of composites. As shown in , the crystallinity of the composite after three modifications has increased from 28% to 41.2%, 36.9%, and 40.6%, respectively. By modifying the straw fiber with NaOH and TEOS treatment, it was found that the cooling crystallization peak area was reduced in the composite system, which decreased the activation energy required for PLA molecular chains to rearrange and enter the lattice, indicating on the one hand that the modified straw fiber contributed to the cooling crystallization of PLA, thus contributing to its crystallinity (Liu et al. Citation2019); on the other hand, the surface of NaOH-treated straw fiber On the other hand, the surface of NaOH-treated straw fiber becomes rough and the nano-SiO2 generated on the surface of straw fiber by TEOS modification provides sites for heterogeneous nucleation of PLA; the introduction of KBM-403 on the surface of straw fiber helps to reduce the polarity of the straw fiber surface, and the long chain of epoxy group in the silane coupling agent acts as a plasticizer of PLA, which makes the movement of PLA molecular chains improve and more molecular chains can be arranged by chain segment during the crystallization process. During the crystallization process, more molecular chains can be aligned and formed into crystals through the movement of chain segments, thus enhancing the crystallinity of the composites.
Figure 6. DSC thermograms of PLA, UN, NaOH, KBM-403, TEOS and thermostability of straw/PLA composites.

Table 2. Thermal transition data of PLA and their composites.
The results of the TGA and derivative thermogravimetric analysis (DTG) are shown in and summarized in . As shown in the figure, all the composites are stable at 200°C. The decomposition in the temperature range of 0–100°C is mainly due to the evaporation of water. When the thermal decomposition of the composite decreases by 5%, the corresponding temperature, i.e., Td5%, increases from 262.9°C to 278.1°C, 289.2°C, and 284.3°C, and the maximum thermal decomposition temperature (Tdmax) of the composite increases from 317.5°C to 333.6°C, 345.67°C, and 345.8°C. The reason is that the increased crystallinity of the modified composite requires more heat to melt the crystalline zone during the thermal decomposition process. Therefore, the initial thermal decomposition temperature and the maximum thermal decomposition temperature of the three modified composites are increased, which indicates that the modified composites have better thermal stability. Based on the final residue analysis, the reason for the reduction in the NaOH treatment content is the removal of lignin and hemicellulose at the end of the straw fibers. The reason for the increase in the KBM-403 residue is that KBM-403 could impart the material with a high heat resistance. The lowest residue observed in the case of the TEOS-modified composite is attributed to the good heat insulation performance of nanoSiO2, which hinders the heat transfer and leads to heat concentration and accelerates the thermal decomposition of the material.
Table 3. Thermostability of PLA and PLA-based composites.
SEM results
shows that the unmodified composite (a) has large pores between the straw fiber and the PLA matrix after the impact, indicating a poor bonding at the interface between the unmodified straw fiber and the PLA. After the NaOH treatment, the number of pores between the straw fiber and the PLA matrix decreased, mainly due to the mechanical locking formed between the rough straw fiber and the PLA. There are no evident pores between the straw fiber modified by KBM-403 and PLA, the silane coupling agent improves the interfacial compatibility problem between WS and PLA very well, and also improves the bonding strength between them, so that WS does not separate from PLA easily. The surface of the straw fibers modified by TEOS evidently shows SiO2, and the interface between the two is better.
Conclusions
The rice straw fiber medicated by NaOH, silane coupling agent, and tetraethyl orthosilicate respectively, the surface morphology and element analysis were carried out by SEM, FTIR and EDS. Then these straw fibers were composited into straw/PLA composite using hot pressing process. The mechanical and thermal properties of the composite samples were tested by universal mechanical testers, DSC and TG. The improved strength mechanism was analyzed by observing the impact section through SEM. Since the bio-composite was developed using waste biomass agricultural by-products and environmentally friendly polylactic acid, it ensured the environmental and cost effectiveness and provide an alternative biological solution for house furnishings and automotive interior trim. The following findings were concluded:
After alkali treatment, there were a lot of grooves on the surface of straw fiber, hemicellulose and lignin content decrease. Coupling agent treatment will add the Si-O bond on surface of straw fiber. After the TEOS treatment, more granular materials (SiO2) could be seen on the surface. These changes are conducive to the composite of straw and polylactic acid.
Compared with the unmodified composites, all three modifications were able to improve the tensile, flexural and impact properties of the composites, but the TEOS modifications did not significantly improve the tensile strength of the composites.
The introduce of Si-O bonds and SiO2 granular improves the interface performance between PLA and straw and elevates the mechanical properties of the composite material.
The crystallinity of the straw/PLA composite after straw surface medicated by NaOH, KBM-403, and TEOS has increased from 28% to 41.2%, 36.9%, and 40.6%, respectively, compared with the unmodified composite. Td5% increases from 262.9°C to 278.1°C, 289.2°C, and 284.3°C, and the maximum thermal decomposition temperature (Tdmax) of the composite increases from 317.5°C to 333.6°C, 345.67°C, and 345.8°C.
Highlights
Preparation of straw fiber composites subjected to various treatments
FTIR spectroscopy, SEM, EDS, and TG analyses of the modified composites
Mechanical property analysis in terms of tensile, bending, and flexural strengths
Explanations regarding the mechanisms responsible for improved strength
Author’s contribution
Methodology, Q.Y.; Conceptualization, F.R.; investigation, C.K., H.W. (Hao Wu) and Z.W.; data curation, G.W.; writing-original draft preparation, F.R.; writing-review and editing, H.W. (He Wang); supervision, Z.X.; project administration and funding acquisition, H.W. (Hongjie Wang). All authors have read and agreed to the published version of the manuscript.
Sample availability
The samples are available from the authors.
Acknowledgements
The work is gratefully supported by Central government guide Anhui Province Science and Technology Development Project (202107d06020014), Anhui Province University Excellent Talent Cultivation Project (gxgnfx2021133), Anhui Provincial Key Laboratory of New Energy Vehicle Light weighting Technology Open Projects (AKL202102), National Innovation and Entrepreneurship Training Program for College Students (202210363046).
Disclosure statement
No potential conflict of interest was reported by the author(s).
Data availability statement
The data are contained within the article.
Additional information
Funding
References
- Chen, K., P. Li, X. Li, C. Liao, X. Li, and Y. Zuo. 2021. Effect of silane coupling agent on compatibility interface and properties of wheat straw/polylactic acid composites. International Journal of Biological Macromolecules 182:2108–14. doi:10.1016/j.ijbiomac.2021.05.207.
- de Castro, B. D., M. Fotouhi, L. M. G. Vieira, P. E. de Faria, and J. C. Campos Rubio. 2021. Mechanical behaviour of a green composite from biopolymers reinforced with sisal fibres. Journal of Polymers and the Environment 29 (2):429–40. doi:10.1007/s10924-020-01875-9.
- Gogoi, R., and A. K. Tyagi. 2019. Surface modification of jute fabric by treating with silane coupling agent for reducing its moisture regain characteristics. Journal of Natural Fibers 18 (6):803–12. doi:10.1080/15440478.2019.1658252.
- Guo, L., L. Shen, S. Chen, A. Wei, D. Huang, A. Osaka, and W. Chen. 2019. Naturally derived silk fibroin/gelatin composites as novel sacrificial template for synthesis of silica nanotubes with controllable size and their in vitro biocompatibility. Materials Letters 251:89–93. doi:10.1016/j.matlet.2019.05.058.
- Hou, X., F. Sun, L. Zhang, J. Luo, D. L. Lu, and Y. Yang. 2014. Chemical-free extraction of cotton stalk bark fibers by steam flash explosion. BioResources 9 (4):6950–67. doi:10.15376/BIORES.9.4.6950-6967.
- Ilyas, R. A., M. Y. M. Zuhri, H. A. Aisyah, M. R. M. Asyraf, S. A. Hassan, E. S. Zainudin, S. M. Sapuan, S. Sharma, S. P. Bangar, R. Jumaidin, et al. 2022. Natural fiber-reinforced polylactic acid, polylactic acid blends and their composites for advanced applications. Polymers 14 (1):202. doi:10.3390/polym14010202.
- Lan, X., S. Chai, J. A. Coulter, H. Cheng, L. Chang, C. Huang, R. Li, Y. Chai, Y. Li, J. Ma, et al. 2020. Maize straw strip mulching as a replacement for plastic film mulching in maize production in a semiarid region. Sustainability 12 (15):6273. doi:10.3390/su12156273.
- Li, W., L. Zheng, D. Teng, D. Ge, F. I. Farha, and F. Xu. 2022. Interfacial modified unidirectional wheat straw/polylactic acid composites. Journal of Industrial Textiles 51 (1_suppl):272S–84. doi:10.1177/1528083720918172.
- Liu, Z. Y., Y. X. Weng, Z. G. Huang, Y. J. Jin, J. Hu, D. Chou, and S. X. Shao. 2019. Manufacture of a hydrophobic CaO/polylactic acid composite. Materials and Manufacturing Processes 34 (3):303–11. doi:10.1080/10426914.2018.1512113.
- Mahmud, S., K. M. F. Hasan, M. A. Jahid, K. Mohiuddin, R. Zhang, and J. Zhu. 2021. Comprehensive review on plant fiber-reinforced polymeric biocomposites. Journal of Materials Science 56 (12):7231–64. doi:10.1007/s10853-021-05774-9.
- Mayilswamy, N., and B. Kandasubramanian. 2022. Green composites prepared from soy protein, polylactic acid (PLA), starch, cellulose, chitin: A review. Emergent Materials 5 (3):727–53. doi:10.1007/s42247-022-00354-2.
- Poonia, N., V. Kadam, N. M. Rose, S. Yadav, and N. Shanmugam. 2022. Effect of fiber chemical treatments on rice straw fiber reinforced composite properties. Journal of Natural Fibers 19 (16):14044–54. doi:10.1080/15440478.2022.2114979.
- Rajeshkumar, G., S. Arvindh Seshadri, G. L. Devnani, M. R. Sanjay, S. Siengchin, J. Prakash Maran, N. A. Al-Dhabi, P. Karuppiah, V. A. Mariadhas, N. Sivarajasekar, et al. 2021. Environment friendly, renewable and sustainable poly lactic acid (PLA) based natural fiber reinforced composites – a comprehensive review. Journal of Cleaner Production 310:127483. doi:10.1016/j.jclepro.2021.127483.
- Ramasubbu, R., and S. Madasamy. 2022. Fabrication of automobile component using hybrid natural fiber reinforced polymer composite. Journal of Natural Fibers 19 (2):736–46. doi:10.1080/15440478.2020.1761927.
- Reddy, K. H., R. M. Reddy, M. Ramesh, D. Mohana Krishnudu, B. M. Reddy, and H. R. Rao. 2019. Impact of alkali treatment on characterization of tapsi (sterculia urens) natural bark fiber reinforced polymer composites. Journal of Natural Fibers 18 (3):378–89. doi:10.1080/15440478.2019.1623747.
- Reddy, P. V., D. Mohana Krishnudu, P. Rajendra Prasad, and V. S. R. R. 2019. A study on alkali treatment influence on prosopis juliflora fiber-reinforced epoxy composites. Journal of Natural Fibers 18 (8):1094–106. doi:10.1080/15440478.2019.1687063.
- Sam-Brew, S., and G. D. Smith. 2017. Flax shive and hemp hurd residues as alternative raw material for particleboard production. BioResources 12 (3):5715–35. doi:10.15376/biores.12.3.5715-5735.
- Sun, E., G. Liao, Q. Zhang, P. Qu, G. Wu, and H. Huang. 2019. Biodegradable copolymer-based composites made from straw fiber for biocomposite flowerpots application. Composites Part B: Engineering 165:193–98. doi:10.1016/j.compositesb.2018.11.121.
- Sun, M., X. Xu, C. Wang, Y. Bai, C. Fu, L. Zhang, R. Fu, and Y. Wang. 2020. Environmental burdens of the comprehensive utilization of straw: Wheat straw utilization from a life-cycle perspective. Journal of Cleaner Production 259:120702. doi:10.1016/j.jclepro.2020.120702.
- Sun, Z., and M. Wu. 2019. Effects of sol-gel modification on the interfacial and mechanical properties of sisal fiber reinforced polypropylene composites. Industrial Crops and Products 137:89–97. doi:10.1016/j.indcrop.2019.05.021.
- Yang, Z., W. Song, Y. Cao, C. Wang, X. Hu, Y. Yang, and S. Zhang. 2017. The effect of laccase pretreatment conditions on the mechanical properties of binderless fiberboards with wheat straw. BioResources 12 (2):3707–19. doi:10.15376/biores.12.2.3707-3719.
- Zhang, X., B. Gao, S. Zhao, P. Wu, L. Han, and X. Liu. 2020. Optimization of a “coal-like” pelletization technique based on the sustainable biomass fuel of hydrothermal carbonization of wheat straw. Journal of Cleaner Production 242:118426. doi:10.1016/j.jclepro.2019.118426.
- Zulkifli, M., M. S. Hossain, N. A. Khalil, A. N. Ahmad Yahaya, F. A. M. Yusof, and A. S. Hashim. 2018. Preparation and characterization of sol-gel silica-modified kenaf bast microfiber/polypropylene composites. BioResources 13 (1):1977–92. doi:10.15376/biores.13.1.1977-1992.
- Zuo, Y., K. Chen, P. Li, X. He, W. Li, and Y. Wu. 2020. Effect of nano-SiO2 on the compatibility interface and properties of polylactic acid-grafted-bamboo fiber/polylactic acid composite. International Journal of Biological Macromolecules 157:177–86. doi:10.1016/j.ijbiomac.2020.04.205.