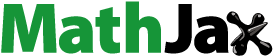
ABSTRACT
Modified coir fiber improves compatibility between fiber and matrix. To explore a better fiber treatment approach and enhance the mechanical properties of composites, this study conducted alkali treatment and acetic anhydride treatment on long and short coir fibers. The effects of different treatments on the properties of coir fibers were characterized using TGA, FTIR, SEM, and stereomicroscopy, and the mechanical properties of the composites were tested. Results showed that the tensile strength of alkali-treated coir fibers (ACF) and acetic anhydride-treated coir fibers (AACF) were remarkably improved. Notably, ACF exhibited a significant 69% increase in tensile strength. The tensile strength of composites with alkali-treated long coir fibers (ALC) and composites with alkali-treated short coir fibers (ASC) reached 34.51 and 21.14 MPa, respectively. The highest flexural strength was found for the acetic anhydride-treated coir fibers, and the flexural strength of composites with acetic anhydride-treated long coir fibers (AALC) and composites with acetic anhydride-treated short coir fibers (AASC) reached 54.38 and 53.46 MPa, respectively. This study presents an improved methodology for enhancing the interfacial bonding ability between coir fibers and the matrix, consequently improving the properties of composites. It offers reference for the investigation of natural fiber composites.
摘要
椰壳纤维增强复合材料具有显著的适用性,但由于椰壳纤维与聚合物基体之间的粘附性较弱,需要进行改性. 为了探索更好的纤维处理方法,提高复合材料的力学性能,本研究对长纤维进行了碱处理,对短纤维进行了醋酸酐处理. 利用热重分析、红外光谱、扫描电镜和立体显微镜表征了不同处理对椰壳纤维性能的影响,并测试了复合材料的力学性能. 结果表明,碱处理的椰壳纤维(ACF)和醋酸酐处理的椰皮纤维(AACF)的抗拉强度均有明显提高. 值得注意的是,ACF的抗拉强度显著提高了69%. 碱处理的椰壳纤维与环氧树脂的界面结合能力最好. 碱处理长椰壳纤维(ALC)复合材料和碱处理短椰壳纤维复合材料的拉伸强度分别达到34.51和21.14MPa. 醋酸酐处理的椰壳纤维的弯曲强度最高,醋酸酐处理长椰壳纤维复合材料(AALC)和醋酸酐处理短椰壳纤维(AASC)复合材料的弯曲强度分别达到54.38和53.46MPa. 本研究提出了一种改进的方法来提高椰壳纤维与基体之间的界面结合能力,从而改善复合材料的性能. 为天然纤维复合材料的研究提供了参考.
Introduction
With increasing awareness on environmental protection, energy efficiency and green development, researchers focus on obtaining and developing environmentally friendly materials from nature (Babu and Narayanankutty Citation2022; Yan et al. Citation2022). Natural fibers, as an extremely accessible and affordable green renewable resource, have received widespread attention due to their affordability, favorable mechanical properties, easy accessibility, biodegradability, and excellent thermal characteristics (Jamshaid et al. Citation2022; Kumar et al. Citation2021; Singh et al. Citation2021). Consequently, they hold immense potential as reinforcing materials in polymers. As a rich biological resource, coir fiber is widely used in the preparation of diverse bio-based materials. This is attributed to its high cellulose content, good comprehensive performance, and renewability. Studies are currently conducted to explore the feasibility of incorporating jute fiber, sisal fiber, sugarcane straw, coir fiber, and bamboo organic waste as reinforcing fibers for bio-composites (Balaji et al. Citation2019; Golova, Magerramova, and Andreeva Citation2020; Kannan and Thangaraju Citation2021; Prabhakar et al. Citation2022; Silviana and Sa’adah Citation2022). Robledo-Ortiz et al. (Citation2021) conducted an evaluation on different sugarcane straw contents as feasible reinforcements/fillers for various biopolymers. The findings reduced the final cost of the potential products while maintaining competitive mechanical properties, thereby expanding possible applications of these materials.
Coir fiber despite being a common cost-effective natural fiber, faces low its utilization rate and limited application prospects. However, in recent years, it has been significant research interest in utilizing fiber as a reinforcing material for composite. This development has led to an improved utilization of coconut shell waste, and the mitigation of environmental problems caused by incineration are solved. Virgin coir fiber, as a solid waste material, virgin coir fiber possesses certain tensile and flexural strength, easy accessibility, and low price and thus is regarded as an excellent reinforcing material for fiber-reinforced composites (Budiman et al. Citation2021). Chowdari and Prasad (Citation2021) investigated the effect of hybrid fiber reinforcement with betel nut fiber and coir fiber powder on the mechanical properties of composites prepared in epoxy resin powder. Gonzalez-Lopez et al. (Citation2019a) evaluated two different surface treatments to improve the compatibility of coir fibers with PLA and compared the effect of both systems on the physical and mechanical properties of PLA-based bio-composites produced by injection molding. shows the modification study of natural fiber.
Table 1. Details of literature review with kind of fiber and treatment method.
Coir fiber possesses favorable mechanical properties; however, its adhesion to the polymer matrix is relatively weak. Inadequate treatment adversely affects the mechanical properties of the composites. Coir fiber modification is crucial to obtain a composite with good performance. Among the various chemical methods employed, alkalization, acetylation, benzoylation, and silane treatment are commonly used. In previous studies, there have been modifications using a single modification method, such as alkalization (Mudoi, Sinha, and Parthasarthy Citation2022), acetylation (Teli and Terega Citation2021), and silane treatment (Vishal et al. Citation2022). Of course, there were also cases where different modification methods were combined for modification, such as, alkalization, bleaching, and benzoylation (Ray et al. Citation2020), and alkalization and silane treatment (Rashid et al. Citation2022). However, there is no comparative analysis of alkali-treated and acetic anhydride-treated. For this experiment, alkali-treated and acetic anhydride-treated were used (Ramachandran et al. Citation2022). In this process, the mechanical properties, surface morphology, and composition of the fiber are changed, and the adhesion of the fiber to the matrix is improved (Ru et al. Citation2022; Subramaniam et al. Citation2022; Wu and Wu Citation2022). Alkali treatment and acetylation are widely employed fiber modification methods in many studies, with NaOH and acetic anhydride being commonly employed solutions for alkali treatment and acetylation modification, respectively.
Previous studies have predominantly focused on utilizing NaOH as the treatment method for coir fibers, examining the impact of factors such as concentration, temperature, and treatment time on fiber properties and the resulting reinforced composites. Nevertheless, while the use of acetic anhydride to modify other natural fibers has been documented (Saidani et al. Citation2022), its use in modifying coir fiber and analyzing its different forms on enhancing the properties of epoxy resin composites, particularly in comparison to NaOH treatment, remains unexplored. Consequently, the aim of this study is to conduct a comparative analysis of the effects of coir fiber treated with NaOH and acetic anhydride on both coir fiber properties and the properties of the resulting reinforced composites. In the current research, untreated coir fibers, NaOH-treated and acetic anhydride-treated, were transformed into long and short fibers and blended with epoxy resin to produce bio-composites. The performance characteristics of coir fibers under different treatments were analyzed by TGA, FTIR, SEM, and stereomicroscopy, and the mechanical properties of the composites were tested, and the tensile, flexural, and hygroscopic properties of the reinforced composites with long and short fiber morphologies were compared. Thus, it is clarified that there is a valuable comparison between acid and alkali treatment for improving the performance of coir fiber composites in paper.
Materials and methods
Materials
Coir fiber was sourced from coconuts grown in Hainan Province, China. The epoxy resin, designated as E-44, was purchased from Guangdong Province, China. Epoxy resin E44 possesses a density of 1150 kg/m3, viscosity of 5000–6000 mPas, and melting point of 145–155°C, and a melting point of 145–155°C. The chemicals used in this experiment were NaOH (Xilong Scientific Co., Ltd., Shantou, China) acetone (Xilong Scientific Co., Ltd., Shantou, China) and acetic anhydride (Xilong Scientific Co., Ltd., Shantou, China).
Acid and alkali modification method of coir fiber
Alkali-treated
Alkali-treated, also known as the mercerization process, is the most commonly used modification method, offering significant effectiveness. Fibers treated with a 5% NaOH solution exhibit better properties, thereby improving the properties of fiber reinforced composites (dos Santos et al. Citation2018; Sahu et al. Citation2023). NaOH reacts with the hydroxyl group of coir fiber, removing hemicellulose, lignin, and impurities on the surface of the coir fiber, reducing diameter of the coir fiber, increasing roughness of the coir fiber, producing new cellulose structure, and increasing hydrophobicity of the coir fiber. Consequently, alkali treatment improves the interfacial adhesion between the fiber and matrix (Hasan et al. Citation2021). The mechanism of alkali-treated can be represented by EquationEquation (1)(1)
(1) .
Acetic anhydride-treated
Acetylation is a well-known esterification method, which can be used for plasticizing natural fibers. The properties of the fiber treated with a 5% acetic anhydride solution are better than that of the untreated coir fiber (Ru et al. Citation2022). When the acetyl group (CH3CO-) in acetic anhydride solution reacts with the hydroxyl group (−OH) in the fiber, the moisture content in the fiber is eliminated. Consequently, the hydrophilic behavior of the fiber is transformed into hydrophobic behavior, which improves the compatibility between the fiber and the matrix and increases the roughness of coir fiber, thus improving the interfacial adhesion of the fiber-matrix (dos Santos et al. Citation2018; Hao et al. Citation2021; Hasan et al. Citation2021; Ru et al. Citation2022). The mechanism of acetic anhydride-treated as shown in EquationEquation (2)(2)
(2) .
Acid and alkali pretreatment of coir fiber
Raw coir fiber was dried by soaking in tap water for 10 h and then in distilled water for 5 h to obtain untreated coir fibers (UCF). Both the long coir fibers (about 100 mm in length) and the short coir fibers (about 5–10 mm in length) are used. As tensile specimens, the long coir fibers (about 130 mm in length) and the short coir fibers (about 5–10 mm in length) were used as flexural specimen. In this experiment, 5% NaOH solution was used for the alkali treatment (Udhayasankar, Karthikeyan, and Balaji Citation2020). The long and short UCFs were placed in an incubator at 30°C for 15 h, taken out, and washed with distilled water to remove the NaOH solution remaining on the coir fibers. The fibers were placed in a drying oven at 60°C for 90 min. Finally, alkali-treated coir fibers (ACF) were obtained. A 5% acetic anhydride solution was used to treat the long and short UCFs. The fibers were then placed in an ultrasonic cleaning apparatus at 30°C for 1 h. The vibration of the apparatus helps facilitate the acetic anhydride treatment. After the treatment, the treated coir fibers were washed with distilled water to remove any residual acetic anhydride solution and then placed in a drying oven at 60°C for 90 min. Finally, acetic anhydride-treated coir fibers (AACF) were obtained.
Preparation of coir fiber composite
Pure epoxy resin block (PEB) was prepared by pouring. The composites were then synthesized by solid solution casting and curing. The epoxy resin and curing agent were mixed in a 1:1 ratio and added with various coir fibers and ensure that each coir fiber surface is coated with a mixture of epoxy resin and curing. The mixture was stirred and poured into molds measuring 100 mm × 12 mm × 4 mm for tensile samples and 135 mm × 13 mm × 3 mm for flexural samples. The curing process took place at room temperature for 24 h. The tensile samples conformed to ASTM D3846, and the flexural samples conformed to ASTM D790 (ASTM D7902010). After the 24-h curing period composites with a filler content of 11.47 wt.% were obtained: composites with untreated long coir fibers (ULC) as reinforcement, composites with untreated short coir fibers (USC) as reinforcement, composites with acetic anhydride-treated long coir fibers (AALC) as reinforcement, composites with acetic anhydride-treated short coir fibers (AASC) as reinforcement, composites with alkali-treated long coir fibers (ALC) as reinforcement, and composites with alkali-treated short coir fibers (ASC) as reinforcement. illustrates the simple production process.
Fiber and composite performance testing
Thermogravimetric analysis
The weight changes of UCF, AACF, and ACF were studied using a thermogravimetric analyzer (Q600, TA, New Castle, DE, USA). Prior to the test, the coir fibers were cut into 1 mm segments, and their specimens were put into the crucible. The test studied the changes in weight loss and derivative weight of coir fibers in testing temperature ranging from 30°C to 600°C. The whole test was conducted under a nitrogen atmosphere, and the heating rate was maintained at 10°C/min.
Fourier-transform infrared spectroscopy
UCF, AACF, and ACF were studied and analyzed with the Fourier-Transform Infrared Spectrometer (FTIR) (T27, Bruker, Billerica, Germany). To prepare the samples, an appropriate quantity of coir fiber powder was mixed and ground with potassium bromide (KBr). The resulting mixture was then pressed into tablets using the tablet pressing method. The data were recorded in the wave numbers ranging from 400 to 4000 cm−1 at a resolution of 4 cm−1. The infrared spectra of each sample could be obtained after scanning.
Distribution and morphological imaging
The morphology of the tensile fracture surfaces of coir fibers and composites under different treatments was studied using scanning electron microscopy (SEM) (Verios G4 UC, Thermo Scientific, Waltham, Massachusetts, USA). Photographs and measurements were captured employing a stereomicroscope (M205 FA, Leica, Wetzlar, Germany). Each fiber was measured once in two mutually perpendicular directions. The average value was taken as the diameter.
Tensile strength test
Tensile test of coir fiber
Tensile strength tests were performed on single fibers using an electronic universal testing machine (3343, INSTRON, Boston, MA, USA). The tensile specimens were stretched at a beam speed of 3 mm/min until fracture transpired. A total of five samples per fiber were tested, and the average value was taken as the tensile strength. The following relationship was used to calculate the tensile strength of the coir fiber (Atmakuri et al. Citation2020).
where A refers to the cross-sectional area, and P refers to the maximum pull-off force.
Tensile test of composite
Different types of tensile tests are extensively used in the polymer industry to determine the optimal strength of reinforced materials. Tensile strength refers to the failure tensile force on a composite under axial load. All specimens were tested on an electronic universal testing machine (3369, INSTRON, Boston, MA, USA) according to ASTM D3846. The tensile specimens were stretched at a beam speed of 5 mm/min until fracture transpired. For each composite, three samples were tested during each trial to obtain the average value. The dimensions of the sample were 100 mm in total length, 70 mm in span length, 12 mm in width, and 4 mm in thickness. The relationship (3) was used to calculate the tensile strength of the composite (Atmakuri et al. Citation2020).
Flexural strength test
Flexural strength is the maximum stress that a material can withstand until it ruptures under load or reaches the specified flexural moment. Such stress is the maximum positive stress in flexural and reflects the ability of a material to resist flexural. It serves as a metric for assessing the flexural performance of the material. The flexural properties of composites were evaluated using the three-point flexural method on an electronic universal testing machine according to ASTM D790 (3369, INSTRON, Boston, Massachusetts, USA). The T-tool was mounted on the fixture, and the specimens were placed in the groove area composed of magnets. The T-tool head was then lowered at a speed of 2 mm/min until the flexural limit was reached. Three samples were tested for each composite to obtain the average value. Flexural strength was tested by placing the composite on a plate with dimensions of 135 mm × 13 mm × 3 mm and a span length of 64 mm. The following formula was used for calculation (Atmakuri et al. Citation2020).
where R is the flexural strength, F is the maximum load, L is the length of the specimen, b is the width, and h is the thickness.
Hygroscopicity test
This test measured the percentage increase in the weight of different types of composites at room temperature. To eliminate moisture content from the samples, heat treatment is conducted by placing them in a drying oven at 60°C for 10 h. The initial mass of the samples was measured, followed by subsequent measurements conducted at 24-h intervals. The size of the specimen was 100 mm × 30 mm × 4 mm. The percentage increase in the weight for each type of composites was calculated over a period of 7 consecutive days. The following formula was used to calculate the hygroscopic property of the specimens.
Results and discussion
Performance characterization of coir fibers
Thermogravimetric analysis results
The thermogravimetric analysis (TGA) curves and the derivative thermogravimetric (DTG) curves of UCF, AACF, and ACF are shown in . The thermal decomposition of natural fiber has three main phases. The first phase corresponds to the evaporation of water in the fiber; the second phase involves the degradation of hemicellulose, pectin, and part of cellulose; the third phase is the decomposition of cellulose, with lignin degradation often considered as a part of the overall process (Yew et al. Citation2019). The DTG curve of UCF is at 60.28°C, 310.85°C, and 377.23°C, which correspond to the three weight losses in the TGA curve of UCF, which are 9.17%, 33.86%, and 25.09%, respectively. The DTG curves of AACF are at 56.26°C, 309.17°C, and 375.21°C, respectively, corresponding to the three weight losses in the TGA curves of AACF, which are 9.17%, 34.35%, and 27.28%, respectively. The DTG curves of ACF show distinct peaks at 64.42°C and 335.66°C, corresponding to the two weight losses in the TGA curves of ACF. These weight losses are approximately 7.84% and 41.74%, respectively. Comparing UCF and AACF, it can be concluded that hemicellulose of coir fiber is removed after alkali-treated, so ACF lacks the second phase (Jiang et al. Citation2019).
Fourier-transform infrared spectroscopy analysis
UCF, AACF, and ACF samples were analyzed using FTIR. The FTIR spectra, as shown in , cover the sample range from 400 cm−1 to 4000 cm−1. provides the positions of absorption peaks and the functional groups. After alkali-treated and acetic anhydride-treated, the functional groups of coir fiber will change after alkali-treated and acetic anhydride-treated. These changes can be characterized by Fourier transform infrared spectroscopy.
Table 2. Peak value of change in FTIR spectrum.
The change of 1738 cm−1 absorption peak corresponds to the C=O stretching vibration in acetyl group of hemicellulose. In ACF, the absorption peak disappears, indicating the removal of most hemicellulose after alkali-treated. In ACCF, the absorption peak slightly increases due to the presence of anhydride groups after acetic anhydride treatment (Putra et al. Citation2020). The absorption peak at 1610 cm−1 is associated with the C=C aromatic stretching vibration in lignin. The reduction in peak intensity indicates the removal of lignin of coir fiber (Fiore et al. Citation2016). The absorption peak of 1379 cm−1 is caused by C-H bending vibration, and the absorption peaks of ACF and AACF are reduced, owing to the removal of some lignin after alkali-treated and acetic anhydride-treated (Ichim et al. Citation2022). The absorption peak at 1265 cm−1 is associated with C-O stretching in acetyl group of lignin. At this position, the absorption peak of ACF and AACF is reduced significantly, indicating that most lignin of coir fiber is removed (Pereira et al. Citation2019). The absorption peak at 898 cm−1 corresponds to the C-H rocking vibration of cellulose. ACF and AACF exhibit an enhanced absorption peak, indicating an increase in the percentage of cellulose after the removal of non-cellulose substances (Ru, Zhao, and Yang Citation2022; Wu et al. Citation2019).
Morphology, diameter, and strength variation of coir fibers
shows that the average diameter of UCF specimen was the largest, followed by AACF specimen, and ACF specimen was the smallest diameter. Similar results were reported by dos Santos et al. (Citation2018), suggesting that alkali-treated coir fibers have a smaller diameter compared to untreated coir fibers. In results, the OH groups of cellulose are converted into ONa groups after alkali-treated, which cellulose is swelled, which promotes the densification of cell walls and reduces the lumen of the elementary fibers. Some hemicellulose, lignin, and impurities covering the surface of the coir fiber are removed after alkali-treated and acetic anhydride-treated, thus reducing the fiber diameter.
The tensile strength of ACF specimen was the largest at 124.94 MPa, followed by that of AACF specimen at 97.85 MPa, and UCF specimens had the lowest tensile strength at 73.83 MPa. Similar to the tensile strength results of Ru, Zhao, and Yang (Citation2022), the tensile strength of treated coir fibers changed from 70.25 MPa to 97.14 MPa compared with untreated coir fibers and an increase of 38.28%. The mechanical properties of coir fiber were improved. However, their modification work only involves alkali treatment without acetylation. The significance of variables is related to p-value, with the values less than 0.05 considered significant. and show the results of ANOVA and Tukey tests for tensile strength, respectively. It can be seen that different methods of fiber treatment have a significant impact. Cellulose provided the main mechanical strength of the fiber because lignin is a fiber component with rigidity and can reduce the mechanical properties of the fiber. When natural fibers are used to make composites, lignin is usually reduced or modified to increase the mechanical strength. ACF specimen showed the largest reduction in lignin content, equipping it with the greatest strength (Istiroyah, Ragil, and Ghufro Citation2021).
Table 3. ANOVA for coir fiber tensile strength.
As components of the skeletal part of the cell wall, a decrease in the content of pectin, lignin, and hemicellulose resulted in a gradual decrease in the flexural strength of coir fibers. Conversely, a notable decrease in the diameter of ACF specimen contributed to an increase in its tensile strength and its flexural resistance.
Surface morphology of coir fibers
show that the presence of impurities on the surface of UCF specimen, with localized nodules being covered by the cuticle layer. In , the removal of the cuticle layer from the AACF specimen resulted in fewer impurities compared to UCF specimen. The knots of fiber surface were observed. indicate that the removed surface cuticle of ACF specimen exhibited the most prominent surface texture and had fewer impurities than compared to the AACF specimens. Udhayasankar, Karthikeyan, and Balaji (Citation2020) reported NaOH solution has reacted with the nodes and strips on the fiber surface, turning the fiber surface a bit smoother than the untreated fiber surface. Many ordered and clear knots were found on the surface of the fiber, consistent with TGA results. Compared with that in UCF specimen, the knot structure on the surface of treated coir fibers facilitated the formation of “mechanical locks” during composite production to improve the interfacial adhesion of coir fibers to epoxy resin blends (Udhayasankar, Karthikeyan, and Balaji Citation2020). Similar to the findings on tensile strength by Ru, Zhao, and Yang (Citation2022), the treated coir fibers have distinct bulges and grooves, which produced mechanical interlocking between the coir fiber and the polymer matrix and effectively improved interface bonding performance, thus improving the mechanical properties of the composites.
Composite properties
Tensile properties
In , the tensile stress–strain curves of PEB, ULC, USC, AALC, AASC, ALC, and ASC depicted. The curves illustrate that the composites have elasticity at low stress, followed by plastic behavior, and finally fail under high stress (Bahners et al. Citation2018; Wang, Yan, and Kasal Citation2022). The stress limit of composite blocks with long reinforcing fibers was all higher surpassing that of PEB. Among all composites with long reinforcing fibers, ALC demonstrates the highest stress limit at approximately 160 MPa; in addition, among all composites with short reinforcing fibers, ASC exhibits the highest stress limit at around 98 MPa. Bahners et al. (Citation2018). reported a similar trend: where the poor fiber – matrix adhesion between unmodified viscose fibers and the matrix restricts the stress transfer between the fiber and the matrix. The result is due to the stress transition between fiber and matrix which is restricted, and the applied load is mainly transferred to the coir fibers, leaving the matrix mainly unstressed.
Figure 7. The tensile stress–strain curves of PEB (a), ULC (b), USC (c), AALC (d), AASC (e), ALC (f), and ASC (g).

presents the tensile strength of different types of composites. The significance of variables is related to p-value, with the values less than 0.05 considered significant. and shows the results of ANOVA and Tukey tests for composite tensile strength, respectively. It can be seen that different types of reinforcing fibers have a significant impact on the tensile properties of composites (De Carvalho et al. Citation2023; Yavuz and Utku Citation2021). The tensile strengths of composite blocks with long reinforcing fibers were all higher than those of PEB, and the tensile strength of composite blocks with treated fibers exceeds that of composite blocks with untreated fibers (Cisneros-Lopez et al. Citation2016). Among them, ALC specimen displays the highest tensile strength at 34.51 MPa, followed by AALC specimen at 31.29 MPa and ULC specimen at 30.48 MPa. The tensile strength of ASC specimen was higher than that of PEB at 21.14 MPa. The tensile strength of AASC and USC specimens was slightly lower than that of PEB due to the presence of many ordered and clear knots on the surface of ACF specimen. Similar to tensile modulus results by Robledo-Ortiz et al. (Citation2020), when the fiber content of agave is 25%, the two times treated agave fiber bio-composites with a tensile strength increase of 75% compared to the untreated agave fiber bio-composites. In work, due to fiber surface modification, which improved the interfacial adhesion and led to better stress transfer from the matrix to the fibers, the tensile strength of composites was improved.
Table 4. ANOVA for composite tensile strength.
The lignin content of coir fiber is reduced, and the hydrogen bond on the coir fiber surface is destroyed after alkali-treated, resulting in increased hydrophobicity and roughness of the fiber, which is beneficial to the compatibility between the fiber and the matrix and improve the interfacial adhesion of the fiber–matrix, thus improving the tensile properties (Hasan et al. Citation2021; Wang, Yan, and Kasal Citation2022). The tensile strength of all composites with long reinforcing fibers surpassed that of composites with short reinforcing fibers. The tensile strength of composites depends on the fiber direction and adhesion between the fiber and matrix for the same fiber content. Only fibers oriented perpendicular to the applied load can effectively reinforce the composite (dos Santos et al. Citation2018; Fiore et al. Citation2016). In all composites with long reinforcing fibers, the distribution of long coir fibers are distributed perpendicular to the applied load. However, the disordered distribution of short coir fibers within the epoxy resin and the lack of continuity between the coir fibers make it difficult for the reinforcing fibers to be pulled out from the matrix. Hence, the tensile strength of composites with short reinforcing fibers is lower than that of composites with long reinforcing fibers.
Flexural properties
In , the flexural stress–strain curves of PEB, ULC, USC, AALC, AASC, ALC, and ASC are displayed. It is approximate to a straight line in the initial part of the flexural stress–strain curve, indicating that the composites are in the elastic deformation in the early stage of initial deformation (Bensalah et al. Citation2021). As the stress increases to the stress limit, the composites undergo plastic deformation without fracturing. Bensalah et al. (Citation2021) reported a similar trend: the composites undergo plastic deformation under high stress. Among all composites with long reinforcing fibers, AALC demonstrates the highest stress limit at approximately 6.63 MPa. AASC has the largest stress limit among all composites with short reinforcing fibers, approximately 6.42 MPa.
Figure 9. The flexural stress–strain curves of PEB (a), ULC (b), USC (c), AALC (d), AASC (e), ALC (f), and ASC (g).

presents the flexural strength of the composites. and show the results of ANOVA and Tukey tests for composite flexural strength, respectively, the p-value in the results of ANOVA is much less than 0.05, it can be seen that different types of reinforcing fibers have a significant impact on the flexural properties of composites. The flexural strength of all composites was higher than that of PEB. However, similar results were reported by Fiore et al. (Citation2016), the flexural strength of composites with treated sisal fibers was all higher than those of composites with untreated sisal fibers. The difference in flexural strength between the long and short fibers treated with acetic anhydride was minimal. Among all long-fiber composites, AALC specimen displays the highest flexural strength at 54.38 MPa, followed by ALC specimen at 50.20 MPa, and ULC specimen exhibits the lowest flexural strength at 48.74 MPa. Among all the short-fiber composites, AASC specimen demonstrates the highest flexural strength at 53.46 MPa, followed by ASC specimen at 35.70 MPa. The flexural strength of USC specimen is slightly higher than that of PEB. Cisneros-Lopez et al. (Citation2016) reported a similar trend: where the treated agave fiber composite and treated coir fiber composite exhibit different improvements in flexural strength compared to the untreated agave fiber composite and untreated coir fiber composite, depending on the fiber content. In their work, for example, when the fiber content is 20%, TAFC and TCFC show flexible strength improvements by up to 17% and 11% compared to UAFC and UCFC, respectively. In the author’s results, the flexural strength of AALC and AASC has increased by 12% and 56% compared to ULC and USC, respectively, these increases can be attributed to the modification treatment improving the fiber – matrix adhesion with better fiber – matrix adhesion, as well as better fiber distribution producing more homogeneous composites with better mechanical properties.
Table 5. ANOVA for composite flexural strength.
The flexural strength of the composites is related to the network system of the fiber in the matrix and the interface bonding ability at the fiber–matrix interface (dos Santos et al. Citation2018). The AACF has a larger diameter compared to ACF, and its network system is stronger than that of ACF, and the fiber–matrix interface bonding ability of AACF is higher than that of UCF. In conclusion, the flexural strength of the composites with AACF is the highest, while the reinforcing effect of the network system of long and short fibers in AACF shows no significant difference.
Tensile cross-sectional analysis of composites
The fibers of the long-fiber-reinforced composites were all pulled off during the tensile test as shown in the cross-sections in . In contrast, the fibers of the short-fiber-reinforced composites remained intact even after extraction as illustrated in . This phenomenon primarily accounts for the significantly higher tensile strength of the long-fiber-reinforced composites compared to the short-fiber-reinforced composites. provide evidence that among the composites reinforced with long fibers, the ACF specimen exhibited the least cuticle on the surface and displayed the tightest interfacial adhesion with the epoxy resin mixture. Almost no fiber pull-out was observed for this composite, indicating the smallest bonding gap between fibers and matrix. AACF specimen ranked second in terms of the tightest interfacial adhesion with the epoxy resin mixture. The cuticle layers on the fiber surface were few, and the fiber was pulled out slightly. The interfacial bonding between UCF specimen and epoxy resin mixture was the lowest. The bonding between the fiber and matrix showed many and large gaps because the cuticle layer on the fiber surface cannot form an effective bond with the matrix (Ru et al. Citation2022). Ru et al. (Citation2022) reported and the surface of untreated coir fiber is wrapped with a large amount of non-cellulose substances, whose structure is loose and interposed between the fiber and the matrix, and debonding can easily occur. In the author’s results, this weakens the interfacial bonding ability between fiber and matrix. Hence, fiber was easily loosened and pulled out during the tensile fracture experiment. Among the composites reinforced with short fibers, the ACF specimen showed the highest interfacial adhesion to the epoxy resin matrix with the smallest gap between the fibers and the matrix. No significant difference in interfacial adhesion with the epoxy resin mixture was observed between AACF and UCF specimens.
Hygroscopicity properties
depicts the percentage increase in the weight of the composites at room temperature. and show the results of ANOVA and Tukey tests for composite hygroscopicity properties, respectively, the p-value under two different factors in the results of ANOVA are much less than 0.05, it can be seen that different types of reinforcing fibers have a significant impact on the hygroscopicity properties of composites. The findings revealed that the percentage increase in the weight of the composites increased with time, with the rate of increase decelerating after 144 h. This finding indicated that the weight of the composite tended to equilibrate after 144 h. No remarkable difference in hygroscopic capacity was found among the composites reinforced with long and short fibers. However, across all cases, the hygroscopic capacity of composite blocks containing treated fibers was lower compared to those with untreated fibers. Similar results were obtained by Gonzalez-Lopez et al. (Citation2019b), when the content of agave fiber is different, compared with untreated agave fiber biocomposites, the water absorption of treated agave fiber biocomposites decreases differently. In the results, water absorption was decreased by up to 39% at 20% of fiber content. In the author’s results, the water absorption of ALC and ASC has increased by 9% and 17% compared to ULC and USC, respectively, the surface treatment promotes better fiber wetting improving their resistance to water uptake because of the polymer layer on the fiber surface decreases their hydrophilicity. ACF specimen exhibited the least hygroscopicity capacity. After 168 h, the percentage increase in the weight was measured at 6.03% for ALC and 5.72% for ASC specimens. The hygroscopic capacity of AACF specimen was similar to that of UCF specimen. The percentage increase in weight was 6.56% for AALC specimen, 6.49% for AASC specimen, 6.61% for ULC specimen, and 6.91% for USC specimen. A low percentage increase in weight indicates that the composite is less hygroscopic but easy to preserve. Conversely, higher hygroscopicity implies greater challenges in preservation. The composites treated with alkali exhibited the weakest hygroscopic capacity but were conducive to long-term preservation. This can be attributed to the removal of hemicellulose of coir fiber after alkali-treated, resulting in reduced hydrophilicity, which not only improves the compatibility of ACF and matrix but also reduces the hygroscopicity of ALC and ASC and improves the moisture resistance (Yew et al. Citation2019). These findings align with the results obtained from FTIR and SEM analyses.
Figure 12. Percentage increase in weight of the composites at room temperature (a), Tukey test for different specimens (b).

Table 6. ANOVA for composite hygroscopicity.
Conclusions
After coir fibers were treated with alkali solution and acetic anhydride solution, an examination of their surface morphology and measurement of their diameter were conducted. Composites can be prepared by mixing various coir fibers with epoxy resin. The mechanical properties of the obtained composites were compared with those of PEB, and both passed the tensile and flexural tests. Hygroscopic performance tests were also conducted. The following conclusions were drawn after these tests. Compared with UCF and AACF specimens, ACF specimen showed many ordered and clear knots on its surface, and the strongest interfacial adhesion to the epoxy resin mixture. Compared with that in PEB, the tensile strength was increased in all composites with long coir fibers as reinforcement. Among which, the tensile strength of ALC was the highest. For the composites with short coir fibers as reinforcement, the tensile strength was higher for ASC specimen than for PEB and slightly lower for USC and AASC specimens than for PEB. Compared with that in PEB, the flexural strength was improved in all composites. Among which, the flexural strength of AALC specimen was the highest. Regarding hygroscopic capacity, no significant differences were found among the composites reinforced with long and short fibers. However, among all the composites, ALC specimen had the weakest hygroscopicity, making it the most easily preservable. This primary objective of this research was to produce and mix hybrid bio-composites using coir fibers as an alternative to industrial synthetic fillers, thereby improving the overall performance of epoxy resin composites.
Highlights
The characteristics of coir fibers treated by different methods are compared and studied.
The principle of the method to improve the interfacial properties between coir fiber and epoxy resin is studied.
The mechanical properties of composites are studied by tensile strength test and flexural strength test.
The moisture resistance of composites is studied by hygroscopicity test.
Acknowledgments
The authors acknowledge the Hainan Provincial Natural Science Foundation of China for supporting this study.
Disclosure statement
No potential conflict of interest was reported by the author(s).
Additional information
Funding
References
- Asumani, O., and R. Paskaramoorthy. 2020. “Fatigue and Impact Strengths of Kenaf Fibre Reinforced Polypropylene Composites: Effects of Fibre Treatments.” Advanced Composite Materials 30 (2): 103–24. https://doi.org/10.1080/09243046.2020.1733308.
- Atmakuri, A., A. Palevicius, M. Siddabathula, A. Vilkauskas, and G. Janusas. 2020. “Analysis of Mechanical and Wettability Properties of Natural Fiber-Reinforced Epoxy Hybrid Composites.” Polymers 12 (12): 2827. https://doi.org/10.3390/polym12122827.
- Babu, S. A., and S. K. Narayanankutty. 2022. “Investigation on the Thermal-Flammability and Mechanical Performance of Coir Fiber Reinforced Biocomposites.” Materials Today: Proceedings 51:2569–2572. https://doi.org/10.1016/j.matpr.2021.12.384.
- Bahners, T., M. Kelch, B. Gebert, X. L. O. Gebert, T. C. Schmidt, J. S. Gutmann, and J. Muessig. 2018. “Improvement of Fibre–Matrix Adhesion in Cellulose/Polyolefin Composite Materials by Means of Photo-Chemical Fibre Surface Modification.” Cellulose 25 (4): 2451–2471. https://doi.org/10.1007/s10570-018-1724-4.
- Balaji, A., K. Sivaramakrishnan, B. Karthikeyan, R. Purushothaman, J. Swaminathan, S. Kannan, R. Udhayasankar, and A. H. Madieen. 2019. “Study on Mechanical and Morphological Properties of Sisal/Banana/Coir Fiber‑Reinforced Hybrid Polymer Composites.” Journal of the Brazilian Society of Mechanical Sciences and Engineering 41 (9): 386. https://doi.org/10.1007/s40430-019-1881-x.
- Ban, Y., W. Zhi, M. E. Fei, W. D. Liu, D. M. Yu, T. F. Fu, and R. H. Qiu. 2020. “Preparation and Performance of Cement Mortar Reinforced by Modified Bamboo Fibers.” Polymers 12 (11): 2650. https://doi.org/10.3390/polym12112650.
- Bensalah, H., M. Raji, H. Abdellaoui, H. Essabir, R. Bouhfid, and A. K. Qaiss. 2021. “Thermo-Mechanical Properties of Low-Cost “Green” Phenolic Resin Composites Reinforced with Surface Modified Coir Fiber.” International Journal of Advanced Manufacturing Technology 112 (7–8): 1917–1930. https://doi.org/10.1007/s00170-020-06535-9.
- Budiman, I., A. Sumarno, A. M. Triastuti, M. Prasetyo, E. Widodo, F. Akbar, B. Subiyanto, A. Nugroho, and A. Nugroho. 2021. “The Properties of Cement Boards Reinforced with Coconut Coir Fiber (Cocos Nucifera) as Building Materials.” IOP Conference Series: Earth and Environmental Science 762 (1): 012074. https://doi.org/10.1088/1755-1315/762/1/012074.
- Chowdari, G. K., and D. V. V. K. Prasad. 2021. “Mechanical Characterization of Areca Fiber and Coconut Shell Powder Reinforced Hybrid Composites.” Materials Science Forum 6042:61–71. https://doi.org/10.4028/WWW.SCIENTIFIC.NET/MSF.1034.61.
- Cisneros-Lopez, E. O., M. E. Gonzalez-Lopez, A. A. Perez-Fonseca, R. Gonzalez-Nunez, D. Rodrigue, and J. R. Robledo-Ortiz. 2016. “Effect of Fiber Content and Surface Treatment on the Mechanical Properties of Natural Fiber Composites Produced by Rotomolding.” Composite Interfaces 24 (1): 35–53. https://doi.org/10.1080/09276440.2016.1184556.
- De Carvalho, A. M. X., M. R. De Souza, T. B. Marques, D. L. De Souza, and E. F. M. De Souza. 2023. “Familywise Type I Error of ANOVA and ANOVA on Ranks in Factorial Experiments.” Ciência Rural 53 (7): e20220146. https://doi.org/10.1590/0103-8478cr20220146.
- dos Santos, J. C., R. L. Siqueira, L. M. G. Vieira, R. T. S. Freire, V. Mano, and T. H. Panzera. 2018. “Effects of Sodium Carbonate on the Performance of Epoxy and Polyester Coir-Reinforced Composites.” Polymer Testing 46:533–544. https://doi.org/10.1016/j.polymertesting.2018.03.043.
- El Boustani, M., G. Lebrun, F. Brouillette, and A. Belfkira. 2017. “Effect of a Solvent-Free Acetylation Treatment on Reinforcements Permeability and Tensile Behaviour of Flax/Epoxy and Flax/Wood Fibre/Epoxy Composites.” The Canadian Journal of Chemical Engineering 95 (6): 1082–1092. https://doi.org/10.1002/cjce.22777.
- Fiore, V., T. Scalici, F. Nicoletti, G. Vitale, M. Prestipino, and A. Valenza. 2016. “A New Eco-Friendly Chemical Treatment of Natural Fibres: Effect of Sodium Bicarbonate on Properties of Sisal Fibre and Its Epoxy Composites.” Composites Part B-Engineering 85:150–160. https://doi.org/10.1016/j.compositesb.2015.09.028.
- Gieparda, W., S. Rojewski, and W. Rozanska. 2021. “Effectiveness of Silanization and Plasma Treatment in the Improvement of Selected Flax Fibers’ Properties.” Materials 14 (13): 3564. https://doi.org/10.3390/ma14133564.
- Golova, T., I. Magerramova, and N. Andreeva. 2020. “Assessing the Application Efficiency of Organic Fiber Filler for Foamed Fiber Concrete.” E3S Web of Conferences 157:06036–06036. https://doi.org/10.1051/e3sconf/202015706036.
- Gonzalez-Lopez, M. E., A. A. Perez-Fonseca, R. Manriquez-Gonzalez, M. Arellano, D. Rodrigue, and J. R. Robledo-Ortiz. 2019a. “Effect of Surface Treatment on the Physical and Mechanical Properties of Injection Molded Poly (Lactic Acid)-Coir Fiber Biocomposites.” Polymer Composites 40 (6): 2132–2141. https://doi.org/10.1002/pc.24997.
- Gonzalez-Lopez, M. E., A. A. Perez-Fonseca, E. O. Cisneros-Lopez, R. Manriquez-Gonzalez, D. E. Ramirez-Arreola, D. Rodrigue, and J. R. Robledo-Ortiz. 2019b. “Effect of Maleated PLA on the Properties of Rotomolded PLA-Agave Fiber Biocomposites.” Journal of Polymers and the Environment 27 (1): 61–73. https://doi.org/10.1007/s10924-018-1308-2.
- Hao, X. L., J. J. Xu, H. Y. Zhou, W. Tang, W. J. Li, Q. W. Wang, and R. X. Ou. 2021. “Interfacial Adhesion Mechanisms of Ultra-Highly Filled Wood Fiber/Polyethylene Composites Using Maleic Anhydride Grafted Polyethylene as a Compatibilizer.” Materials & Design 212:110182. https://doi.org/10.1016/j.matdes.2021.110182.
- Hasan, K. M. F., P. G. Horvath, M. Bak, and T. Alpar. 2021. “A State-Of-The-Art Review on Coir Fiber-Reinforced Biocomposites.” Royal Society of Chemistry 11 (18): 10548–10571. https://doi.org/10.1039/d1ra00231g.
- Hashim, M. Y., A. M. Amin, O. M. F. Marwah, M. H. Othman, M. R. M. Yunus, and N. C. Huat. 2017. “The Effect of Alkali Treatment Under Various Conditions on Physical Properties of Kenaf Fiber.” International Conference on Materials Physics and Mechanics 914:12030. https://doi.org/10.1088/1742-6596/914/1/012030.
- Ichim, M., L. Stelea, I. Filip, G. Lisa, and E. I. Muresan. 2022. “Thermal and Mechanical Characterization of Coir Fibre–Reinforced Polypropylene Biocomposites.” Crystals 12 (9): 1249. https://doi.org/10.3390/cryst12091249.
- Istiroyah, M. B., S. Ragil, and M. Ghufro. 2021. “Effect of Sonification in the Alkalization Process of Coconut Fiber to Improve Fiber Strength.” IOP Conference Series: Earth and Environmental Science 743 (1): 012041. https://doi.org/10.1088/1755-1315/743/1/012041.
- Jamshaid, H., R. K. Mishra, A. Raza, U. Hussain, M. L. Hussain, S. Nazari, V. Chandan, M. Muller, and R. Choteborsky. 2022. “Natural Cellulosic Fiber Reinforced Concrete: Influence of Fiber Type and Loading Percentage on Mechanical and Water Absorption Performance.” Materials 15 (3): 874. https://doi.org/10.3390/ma15030874.
- Jiang, Y., P. H. Deng, L. X. Jing, and T. H. Zhang. 2019. “Tensile Properties and Structure Characterization of Palm Fibers by Alkali Treatment.” Fibers and Polymers 20 (5): 1029–1035. https://doi.org/10.1007/s12221-019-7841-3.
- Kannan, G., and R. Thangaraju. 2021. “Recent Progress on Natural Lignocellulosic Fiber Reinforced Polymer Composites: A Review.” Journal of Natural Fibers 19 (13): 7100–7131. https://doi.org/10.1080/15440478.2021.1944425.
- Kumar, B., D. O. Agumba, D. H. Pham, M. Latif Dinesh, H. C. Kim, J. Alrobei, H. Kim, and J. Kim. 2021. “Recent Research Progress on Lignin-Derived Resins for Natural Fiber Composite Applications.” Polymers 13 (7): 1162. https://doi.org/10.3390/polym13071162.
- Kumar, N., and D. Das. 2017. “Alkali Treatment on Nettle Fibers: Part I: Investigation of Chemical, Structural, Physical, and Mechanical Characteristics of Alkali-Treated Nettle Fibers.” The Journal of the Textile Institute 108 (8): 1461–1467. https://doi.org/10.1080/00405000.2016.1257346.
- Mudoi, M. P., S. Sinha, and V. Parthasarthy. 2022. “Optimizing the Alkali Treatment of Cellulosic Himalayan Nettle Fibre for Reinforcement in Polymer Composites.” Carbohydrate Polymers 296:119937. https://doi.org/10.1016/j.carbpol.2022.119937.
- Pereira, J. F., D. P. Ferreira, J. Bessa, J. Matos, F. Cunha, I. Araujo, L. F. Silva, E. Pinho, and R. Fangueiro. 2019. “Mechanical Performance of Thermoplastic Olefin Composites Reinforced with Coir and Sisal Natural Fibers: Influence of Surface Pretreatment.” Polymer Composites 40 (9): 3472–3481. https://doi.org/10.1002/pc.25209.
- Prabhakar, C. G., K. A. Babu, P. S. Kataraki, and S. Reddy. 2022. “A Review on Natural Fibers and Mechanical Properties of Banyan and Banana Fibers Composites.” Materials Today: Proceedings 54:348–358. https://doi.org/10.1016/j.matpr.2021.09.300.
- Putra, A. E. E., I. Renreng, H. Arsyad, and B. Bakri. 2020. “Investigating the Effects of Liquid-Plasma Treatment on Tensile Strength of Coir Fibers and Interfacial Fiber-Matrix Adhesion of Composites.” Composites Part B-Engineering 183:107722. https://doi.org/10.1016/j.compositesb.2019.107722.
- Ramachandran, A., S. M. Rangappa, V. Kushvaha, A. Khan, S. Seingchin, and H. N. Dhakal. 2022. “Modification of Fibers and Matrices in Natural Fiber Reinforced Polymer Composites: A Comprehensive Review.” Macromolecular Rapid Communications 43 (17): 2100862. https://doi.org/10.1002/marc.202100862.
- Rashid, N. I. H., R. M. Reddy, A. R. Venkataramanan, M. V. D. Poures, A. Thanikasalam, A. Elfasakhany, A. Alemayehu, and K. Raja. 2022. “The Influence of Chemically Treated Hemp Fibre on the Mechanical Behavior and Thermal Properties of Polylactic Acid Made with FDM.” Advances in Materials Science and Engineering 2022:1–9. https://doi.org/10.1155/2022/6953136.
- Ray, R., S. N. Das, A. Mohapatra, and H. C. Das. 2020. “Comprehensive characterization of a novel natural Bauhinia Vahlii stem fiber.” Polymer Composites 41 (9): 3807–3816. https://doi.org/10.1002/pc.25678.
- Robledo-Ortiz, J. R., A. S. Del Campo, J. A. Blackaller, M. E. Gonzalez-Lopez, and A. A. Perez Fonseca. 2021. “Valorization of Sugarcane Straw for the Development of Sustainable Biopolymer-Based Composites.” Polymers 13 (19): 3335. https://doi.org/10.3390/polym13193335.
- Robledo-Ortiz, J. R., M. E. Gonzalez-Lopez, A. S. M. Martin Del Campo, L. Peponi, R. Gonzalez-Nunez, D. Rodrigue, and A. A. Perez-Fonseca. 2020. “Fiber-matrix interface improvement via glycidyl methacrylate compatibilization for rotomolded poly(lactic acid)/agave fiber biocomposites.” Journal of Composite Materials 55 (2): 201–212. https://doi.org/10.1177/0021998320946821.
- Ru, S. F., C. Zhao, and S. M. Yang. 2022. “Multi-Objective Optimization and Analysis of Mechanical Properties of Coir Fiber from Coconut Forest Waste.” Forests 13 (12): 2033. https://doi.org/10.3390/f13122033.
- Ru, S. F., C. Zhao, S. M. Yang, and D. Liang. 2022. “Effect of Coir Fiber Surface Treatment on Interfacial Properties of Reinforced Epoxy Resin Composites.” Polymers 14 (17): 3488. https://doi.org/10.3390/polym14173488.
- Sahu, S., S. Nayak, S. B. B. P. J. Sahu, and M. K. Roul. 2023. “Influence of Various Surface Treatments on Mechanical, Thermal, Morphological, and Water Absorption Properties of Rattan (Calamus Beccarii) Fiber.” Journal of Natural Fibers 20 (1): 30–44. https://doi.org/10.1080/15440478.2022.2125924.
- Saidani, K., G. Racelma, K. Cheraitia, and A. Lounis. 2022. “Elaboration and Study of the Properties of a Polyester Composite Made of Oleaster Fibers Treated and Untreated.” Journal of Natural Fibers 19 (16): 14414–14421. https://doi.org/10.1080/15440478.2022.2064395.
- Senthilraja, R, Sarala, R, Antony, A. G, and Seshadhri. 2020 Effect of acetylation technique on mechanical behavior and durability of palm fibre vinyl-ester composites Materials Today: Proceedings 21 634–637. https://doi.org/10.1016/j.matpr.2019.06.729.
- Siakeng, R., M. Jawaid, M. R. Saba, N. Sanjay, H. Siengchin, S. Fouad, and H. Fouad. 2020. “Alkali Treated Coir/Pineapple Leaf Fibres Reinforced PLA Hybrid Composites: Evaluation of Mechanical, Morphological, Thermal and Physical Properties.” Express Polymer Letters 14 (8): 717–730. https://doi.org/10.3144/expresspolymlett.2020.59.
- Silva, N. G. S., T. F. Maia, and D. R. Mulinari. 2021. “Effect of Acetylation with Perchloric Acid as Catalyst in Sugarcane Bagasse Waste.” Journal of Natural Fibers 19 (13): 5050–5064. https://doi.org/10.1080/15440478.2021.1875352.
- Silviana, S., and A. N. Sa’adah. 2022. “Mechanical and Thermal Properties of Bacterial Cellulose Reinforced With Bamboo Microfibrillated Cellulose and Plasticized With Epoxidized Waste Cooking Oil.” Cellulose Chemistry and Technology 56 (3–4): 331–339. https://doi.org/10.35812/CelluloseChemTechnol.2022.56.29.
- Singh, Y., J. Singh, S. Sharma, A. Sharma, and J. S. Chohan. 2021. “Process Parameter Optimization in Laser Cutting of Coir Fiber Reinforced Epoxy Composite - a Review.” Materials Today: Proceedings 48 (5): 1021–1027. https://doi.org/10.1016/J.MATPR.2021.06.344.
- Subramaniam, B., V. M. Manickavasagam, P. T. Rajkumar I, P. A. C. Raj, V. G. Bharath, J. Madhusudhanan, A. K. Sharma, P. Patil, G. B. Assefa, and V. Vijayan. 2022. “Investigation of Mechanical Properties of Sansevieria Cylindrica Fiber/Polyester Composites.” Advances in Materials Science and Engineering 2022:1–6. https://doi.org/10.1155/2022/2180614.
- Teli, M. D., and J. M. Terega. 2021. “Solvent-Free Acetylation of Ensete Ventricosum Plant Fibre to Enhance Oleophilicity.” The Journal of the Textile Institute 113 (9): 1958–1966. https://doi.org/10.1080/00405000.2021.1957292.
- Udhayasankar, R., B. Karthikeyan, and A. Balaji. 2020. “Comparative Mechanical, Thermal Properties and Morphological Study of Untreated and NaOh-Treated Coconut Shell-Reinforced Cardanol Environmental Friendly Green Composites.” Journal of Adhesion Science and Technology 34 (16): 1720–1740. https://doi.org/10.1080/01694243.2020.1727643.
- Vishal, K., K. Rajkumar, M. S. Nitin, and P. Sabarinathan. 2022. “Kigelia africana fruit biofibre polysaccharide extraction and biofibre development by silane chemical treatment.” International Journal of Biological Macromolecules 209:1248–1259. https://doi.org/10.1016/j.ijbiomac.2022.04.137.
- Wang, B., L. Yan, and B. Kasal. 2022. “A Review of Coir Fibre and Coir Fibre Reinforced Cement-Based Composite Materials (2000–2021).” Journal of Cleaner Production 338:130676. https://doi.org/10.1016/j.jclepro.2022.130676.
- Wu, J., X. Y. Du, Z. B. Yin, S. Xu, S. Y. Xu, and Y. C. Zhang. 2019. “Preparation and Characterization of Cellulose Nanofibrils from Coconut Coir Fibers and Their Reinforcements in Biodegradable Composite Films.” Carbohydrate Polymers 211:49–56. https://doi.org/10.1016/j.carbpol.2019.01.093.
- Wu, C. C., and C.-C. Wu. 2022. “Effective Case Depth and Wear Resistance of Pack Carburized SCM 420 Steel Processed Using Different Concentrations of Natural Shell Waste Powders and Carburizing Duration.” Crystals 12 (2): 296. https://doi.org/10.3390/cryst12020296.
- Yan, X., C. H. Liu, L. Qiao, K. L. Zhu, H. S. Tan, S. H. Dong, and Z. T. Lin. 2022. “Crystallization and Dynamic Mechanical Behavior of Coir Fiber Reinforced Poly (Butylene Succinate) Biocomposites.” Journal of Renewable Materials 10 (4): 1039–1048. https://doi.org/10.32604/jrm.2022.017239.
- Yavuz, H., and D. H. Utku. 2021. “Parametric and Non-Parametric Tests for the Evaluation of Interlaminar Fracture Toughness of Polymer Composites.” Journal of Reinforced Plastics and Composites 40 (11–12): 450–462. https://doi.org/10.1177/0731684420973078.
- Yew, B. S., M. Muhamad, S. B. Mohamed, and F. H. Wee. 2019. “Effect of Alkaline Treatment on Structural Characterisation, Thermal Degradation and Water Absorption Ability of Coir Fibre Polymer Composites.” Sains Malaysiana 48 (3): 653–659. https://doi.org/10.17576/jsm-2019-4803-19.