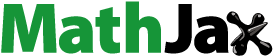
ABSTRACT
Bamboo fiber (BF) has emerged as a promising natural, environmentally friendly material for use in composite materials. The steam explosion process plays a crucial role in preparing BFs suitable for composites. In this study, the effects of solid–liquid ratio, explosion pressure, and explosion holding time on BF preparation were investigated by orthogonal experiments, and the structural and chemical changes occurring during the separation of BF were also investigated. The results showed that the combined method of alkali treatment and steam explosion successfully produced BFs with good morphology. The highest yield of fine fibers (39.07%) was obtained at a solid–liquid ratio of 2:1, explosion pressure of 2.2 MPa, and explosion holding time of 4 min. BFs obtained using the optimal values exhibited dispersed fiber bundles, partial removal of hemicellulose and lignin, and improved crystallinity. Furthermore, the hygroscopicity of BFs decreased, reaching a minimum of 15% under the optimal process conditions. The optimization of steam explosion parameters can help to improve the extraction efficiency of fine fibers for composites and promote the industrialization of BFs prepared by steam explosion.
摘要
竹纤维(BF)已成为复合材料领域中一种前景广阔的天然环保材料. 蒸汽爆破工艺在制备适用于复合材料的BFs中起着至关重要的作用. 本研究采用正交实验研究了固液比、爆破压力和保压时间对BF制备的影响,并研究了BF分离过程中发生的结构和化学变化. 结果表明,碱处理和蒸汽爆破相结合的方法成功地生产出了形态良好的BF. 在固液比为2:1、爆破压力为2.2 MPa、爆破保压时间为4 min的条件下,细纤维的产率最高(39.07%). 使用最佳值获得的BFs为分散的纤维束,部分去除半纤维素和木质素并提高了结晶度. 此外,在最佳工艺条件下,BFs的吸湿性降低,最低可达15%. 蒸汽爆破参数的优化有助于提高适用于复合材料的细纤维的提取效率,促进蒸汽爆破法制备BFs的工业化.
Introduction
Bamboo is a suitable choice for the development of natural fiber composites. As a unique processing product of bamboo, bamboo fiber (BF) is called “natural glass fiber” as the main component and load-bearing unit of bamboo. Compared to other natural fibers, BFs offer several advantages, including low density, high strength, and biodegradability (Gao et al. Citation2022; Lin et al. Citation2022). Therefore, its composites are increasingly used in construction, furniture, energy, automotive interiors, and biomimetics. These applications can substitute or reduce the usage of nonrenewable glass fiber resources (Hu et al. Citation2023; Liu et al. Citation2012; Shi et al. Citation2023). BF grows mainly in the fiber sheaths of vascular bundles, and its preparation is mainly obtained by removing the parenchymal tissues of bamboo and extracting the fiber sheaths from the bamboo vascular bundles.
The current methods for preparing BF bundles are mainly physical methods, chemical methods, biological methods, and combined preparation methods (Gao et al. Citation2022; Liu et al. Citation2012; Zakikhani et al. Citation2014). The physical method is simple to operate, but it often leads to damaged fiber bundles that are unsuitable for direct utilization. The chemical method is a promising technology for the effective removal of hemicellulose and lignin with high efficiency, but it is costly and polluting. The biological method has very high requirements for the process environment and is generally used for the refinement of BFs. Since the complex structure of bamboo and its high lignin content hinder fiber extraction promptly, it is difficult to extract fibers using only one method. Thus, utilizing combined preparation methods that incorporate two or three preparation processes is more efficient and yields better outcomes compared to single methods (Rocky and Thompson Citation2018). The steam explosion method originated in the last century in pulp and paper making. It mainly uses water vapor of high temperature and pressure to treat raw materials and realizes the separation of components and structural changes of raw materials through instantaneous pressure release to achieve the effect of dispersing fibers. As the rapidly developing and most cost-effective fiber separation technology in recent years, the advantage of the steam explosion method is that the steam can penetrate the internal crevices of the fiber and improve the separation and uniformity of BFs. The whole process is pollution-free and energy-efficient, but it also has the disadvantage of only partially degrading and removing hemicellulose and lignin (Chen et al. Citation2022; Sun et al. Citation2014). Therefore, it is necessary to use a combination method of chemical pretreatment and steam explosion, which can produce long fibers with a good separation effect (Zakikhani et al. Citation2014). Alkali pretreatment of bamboo can improve the wetting up of BFs and degrade or dissolve most of the lignin between cell walls (Chen et al. Citation2016). In addition to improving the degree of fiber separation and homogeneity, the alkali pretreatment also improves the roughness of the fiber surface, thereby reducing fiber polarity. This allows for better infiltration of the matrix resin and improves the bonding between the fiber and the resin.
The combined method of alkali pretreatment and steam explosion has been used in industrial applications, such as improving the enzymatic digestibility and ethanol production to facilitate the bioconversion of bamboo biomass into biofuel (Yuan et al. Citation2020); or as a combined degumming method to prepare kudzu fibers (Song et al. Citation2017). Unfortunately, the majority of current studies on the preparation of BFs predominantly focus on a single preparation method, and the combined preparation method of alkali pretreatment and steam explosion has not been paid attention to and further studied. Exploring the process of this method would be beneficial in achieving BFs with optimal separation and enhancing the efficiency of production. Additionally, it would provide valuable theoretical support for the industrial-scale production of BFs using the combined preparation method.
In this study, BF bundles were prepared by combined preparation methods utilizing the orthogonal experiment. The explosion pressure and holding time are the most basic parameters in the preparation method. The former determines the force during the steam explosion pressure relief stage, while the latter represents the processing time of the steam explosion. In addition, the solid–liquid ratio also plays an important role. The solid–liquid ratio refers to the weight ratio between bamboo slivers and the added water in the steam explosion device, which represents the degree of moisture infiltration and affects the infiltration pathway of water molecules. All three parameters have an impact on the degree of steam explosion. Therefore, the effects of different parameters such as solid–liquid ratio, explosion pressure, and explosion holding time were studied, and the optimal values of these parameters were selected to provide a reference for the preparation conditions of fiber bundles. The discrepancy of different steam explosion processes in terms of morphology, microstructure, chemical composition, hygroscopicity, and thermal stability was examined by digital microscope, scanning electron microscopy (SEM), Fourier-transform infrared spectroscopy (FT-IR), X-ray diffraction (XRD), dynamic vapor sorption (DVS), and thermogravimetric analysis (TGA).
Materials and methods
Materials
Three-year-old Moso bamboo (Phyllostachys heterocycla (Carr.) Mitford cv. Pubescens) was harvested from Zhejiang Province, China. The bamboo was cut into bamboo slivers with a length of 60 mm, a width of 20 mm, and a thickness of 5 mm. The sodium hydroxide (NaOH) was analytically pure and supplied by Chengdu Cologne Chemical Co., Ltd. Bamboo slivers were soaked in a 5% NaOH solution and then subjected to steaming in a thermostatic water bath cauldron maintained at 100°C for 1 h. The pretreated bamboo slivers were washed extensively with water after being rolled once in a cycle by a rolling machine with a rolling gap of 2 mm to eliminate the alkali and neutralize them.
Steam explosion and fiber sorting
A certain quality of bamboo slivers was weighed and put into the steam explosion device. The explosion pressure and explosion holding time were set to a certain value, then the heating device was powered on. The saturated steam produced was continuously fed into the explosion device to reach the preset experimental pressure. The valve was then closed, and the timer was started. Upon completion of the set time, BFs in the explosion chamber were sprayed into the receiving device, collected, and subsequently dried. The process flow chart of BF preparation is shown in .
The generated BFs were classified into four categories based on their morphology: fluffy fibers, fine fibers, half separated bamboo strips, and unseparated bamboo strips. The yields of each type of BF were then calculated. Fluffy fibers are identified by their flocculent appearance or resemblance to bamboo powder. Fine fibers refer to fully separated fiber bundles. Half separated bamboo strips are materials where one end and the middle are tightly connected while the other end is separated. Unseparated bamboo strips refer to bamboo strips that remain intact without any separation.
Optimization of steam explosion
The processes of the steam explosion were optimized using orthogonal experiments. The investigation focused on the solid–liquid ratio, explosion pressure, and explosion holding time as the variables, with the yield of fine fibers serving as the reference index. Each factor was examined at three levels using an L9 orthogonal table. The design of the levels of the orthogonal experimental factors and the number of BFs obtained under different steam explosion processes were in and , respectively.
Table 1. The design of the level of orthogonal experimental factor.
Table 2. The number of BFs obtained under different steam explosion processes.
Characterization
Color and surface appearance
Color parameters were measured with a high-precision colorimeter (SR-68, KONICA MINOLTA, Japan). Three replicates were measured for each sample. The differences in brightness (ΔL*), red/green difference (Δa*), yellow/blue difference (Δb*) and color change (ΔE*) were calculated according to the following Eq. (1):
The morphology of BFs was observed by a digital microscope (VHX-7000, KEYENCE, China).
Microstructure and chemical composition
The surface and cross-section of BFs prepared by different steam explosion processes were observed with a field emission scanning electron microscope (S-3400N, Hitachi, Japan). FT-IR analysis was run on a spectrometer (VERTEX 80 V, Bruker, Karlsruhe, Germany). About 1 mg of BFs was milled into small particles and then mixed with KBr and pressed into a small disc about 0.5 mm thick. The FT-IR spectra were recorded at a spectral range of 4000–500 cm−1 at a resolution of 4 cm−1 and 64 scans. Three replicates were measured for each sample. BFs were cut into powder for XRD testing. The crystal structure of cellulose in samples was measured by an X-ray diffractometer (XRD, Ultima IV, Rigaku, Japan) in a range of 2θ = 5–45° with a scanning rate of 10°/min with a CuKα radiation source. The crystalline index (CrI) of BFs was calculated by the commonly employed peak-height method (Segal et al. Citation1959), according to Eq. (2):
where I200 is the intensity of the (200) peak at about 2θ = 22.4° after subtracting the background signal, and Iam represents the intensity of the amorphous region at about 2θ = 18°.
Dynamic water vapor sorption
The obtained curves were analyzed to determine the moisture adsorption characteristics. To ensure consistency in the experimental conditions, the temperature was kept constant throughout the test. Samples weighing between 30 and 50 mg were placed in weighing bottles and dried thoroughly. In this study, the step mass change rate was set to be less than 0.001%/min, and the relative humidity range used was 0% to 95%. The humidity gradient was 0, 10, 20, 30, 40, 50, 60, 70, 80, 90, and 95%.
Thermogravimetric analysis
The thermogravimetric (TG) and derivative thermogravimetric (DTG) analyses of BFs were examined by a thermogravimetric analyzer (TGA, STA409PC, Netzsch, Germany). The experiment was conducted using a constant heating rate of 20°C per minute, starting from room temperature to 800°C. The experiment was carried out under an inert atmosphere (pure nitrogen) with a flow rate of 20 mL/min and 40 mL/min.
Results and discussion
Yield and color
The yields of different types of BFs prepared by different steam explosion processes are shown in . Since the vascular bundles on the inner skin of bamboo slivers are fewer, pretreatment and steam explosion tend to cause more damage to the inner BFs, facilitating the production of fluffy fibers. Conversely, the vascular bundles on the outer skin of bamboo are more, which is difficult to destroy during pretreatment and steam explosion, easy to produce half separated bamboo strips and unseparated bamboo strips with poor separation effect. During the pretreatment process, a significant amount of hemicellulose and lignin in the bamboo slivers was removed by the NaOH solution, resulting in the softening of the bamboo slivers (Chen et al. Citation2021). Then, bamboo slivers were extruded and deformed by the continuous lateral squeezing pressure of the rollers during rolling. The bamboo slivers were in the shape of fiber bundles after rolling, and the fiber separation was improved. During the steam explosion process, the combined effect of water vapor and heat produced acid-like degradation and thermal degradation of fiber materials, the dissolution of low molecular substance, and the polymerization degree of fiber decreased, resulting in the BFs with high separation. When preparing composites with enhanced performance using the steam explosion method, fine fibers with a moderate degree of separation are prioritized, so the yield of fine fibers is particularly important (Zhao et al. Citation2023).
Figure 2. (a) Yields of different types of BFs under different steam explosion processes. (b) Changes in color parameters of BFs under different steam explosion process.

The solid–liquid ratio had a significant effect on the yield of fine fibers, and the highest yield of fine fibers was 39% when the solid–liquid ratio was 2:1. Moisture, as the only working medium in the steam explosion process, is closely related to the explosion effect. However, when the degree of moisture infiltration was too high, it would lead to a reduction in the heat energy released from the steam, thus affecting the explosion effect, so the yield of fine fibers decreased as the water proportion increased. When the solid–liquid ratio was 1:2, the yield of fine fibers was significantly reduced. The yield of fine fibers was reduced to 4%, while the solid–liquid ratio, explosion pressure, and explosion holding time were 1:2, 1.8 MPa, and 1 min, respectively, which was 35% lower than the highest yield. This may be due to the fact that the bamboo slivers were in a water saturated state because we soaked them in water before the steam explosion, the excess water during the steam explosion would prevent the circulation of steam in the rolling gap and internal gap of bamboo slivers, resulting in the inability of steam to penetrate the bamboo slivers, making the low separation of BFs. The degree of separation and uniformity of separation of BFs increased with the increase of steam explosion pressure and time. The yield of fine fibers was highest when the solid–liquid ratio, explosion pressure, and explosion holding time were 2:1, 2.2 MPa, and 4 min, respectively.
The surface morphology of BFs prepared by different steam explosion processes is shown in . When the solid–liquid ratio was 2:1, the material maintained its overall morphology with a low degree of separation. However, when the solid–liquid ratio was 1:1 and 1:2, the material gradually separated into bamboo fiber bundles. The increase in moisture content provided a greater pathway for water infiltration, thus improving the degree of fiber separation. As the explosion pressure and holding time increased, the degree of fiber separation also increased, and the bundled fibers became more filamentous. When the explosion pressure reached 2.2 MPa, the fibers transitioned mostly from bundled to filamentous, indicating that explosion pressure has a significant influence on the degree of fiber separation. The color parameters of the BFs prepared by different steam explosion processes are shown in . ΔL* decreased with the increase of explosion holding time, indicating that the color of BFs darkened significantly with increasing holding time. In addition, Δb* decreased and Δa* had little change, the color of BFs turned bluish. The effect of explosion pressure on the color of BFs was consistent with the explosion holding time, but the change was not as pronounced as the explosion holding time. The color of BFs was mainly affected by the explosion pressure and holding time, and the solid–liquid ratio had less effect on the color of BFs.
Optimization of steam explosion
According to the extreme results presented in , the influence on the content of fine fibers in BFs can be ranked as follows: solid–liquid ratio > explosion pressure > explosion holding time, denoted as A > B > C. Taking the content of fine fibers in the extracted BFs as the index of investigation, the best process combination of fine fiber extraction in BFs was A1B3C2, resulting in a final fine fiber extraction rate of 39.02%. The results of orthogonal experiments showed that the main order influencing the fine fiber content in BFs was solid–liquid ratio > explosion pressure > explosion holding time, which was consistent with the results of the extreme difference analysis. further indicated that the effects of these three factors on the content of fine fibers in BFs were highly significant (P<0.01). Considering all the factors, the recommended process combination for the preparation of BFs is A1B3C2, which involves a solid–liquid ratio of 2:1, an explosion pressure of 2.2 MPa, and an explosion holding time of 4 min. With this combination, the extraction rate of fine fibers in BFs was measured to be 39.02%.
Table 3. Orthogonal experimental combination and results for different steam exploded BFs.
Table 4. Orthogonal experimental ANOVA for different steam exploded BFs.
Size and microstructure
The dimensions of BFs obtained under different steam explosion processes are shown in . The length distribution of the BFs was relatively consistent, mostly falling within the range of 30–90 mm. Notably, when the explosion holding time was set to 1 min, BFs produced with steam explosion pressures of 1.4 MPa and 1.8 MPa exhibited greater lengths exceeding 90 mm. This suggested that minimal damage was incurred to the bamboo slivers when lower explosion pressures and shorter holding times were employed. Additionally, the diameter of the BFs predominantly fell within the range of 0–0.4 mm. It is worth mentioning that the diameter of the BFs decreased as the explosion holding time increased. Hence, it is evident that the steam explosion process can cause slight variations in both the length and diameter ranges of the BFs.
The surface microstructure of BFs of different steam explosion processes is shown in . During the steam explosion process, steam entered the cell walls and the pores between fibers, and formed hydrogen bonds with some of the hydroxyl groups in the cellulose (Tanpichai et al. Citation2019). Under the combined action of steam and heat, the fibers began to soften and degrade. In the process of pressure relief, the high-pressure steam was instantaneously released from the fiber gap, which had a mechanical separation effect on the softened fibers, breaking the hydrogen bonds between the cellulose molecules, reducing the connection between the fibers, and causing the separation between the fiber bundles. A certain degree of wrinkling occurred on the fiber surface, which may be due to the fact that the NaOH solution removed a large amount of lignin and part of hemicellulose during the pretreatment process of bamboo slivers. During the fiber drying process, the lateral distance of microfibrils was obviously reduced, and the fiber showed wrinkling on a macroscopic level. Additionally, granular material appeared on the surface of BFs. This may be caused by the destruction of the structure of cellulose, hemicellulose, and lignin under the action of high temperature and pressure, partial breakage of hydrogen bonded inside cellulose, and formation of free hydroxyl group, which increased the adsorption capacity of cellulose. In the process of instantaneous pressure relief, the dissolved hemicellulose degradation products and lignin accumulated on the fiber surface. The fibers were still fibrous bundles with no tendency to disperse when the steam explosion pressure was 1.4 MPa and 1.8 MPa, which was probably because the hydrogen bond breaking effect of steam on fibers was weak, and the degradation of hemicellulose and lignin as bonding substances was low, resulting in the bonding of fiber (Hongzhang and Liying Citation2007). However, when the explosion pressure was 2.2 MPa, fiber bundles were dispersed with partial single fibers.
Figure 4. (a) Surface and (b) cross-section microstructure of BFs under different steam explosion processes.

The scanning electron microscope of the cross-section of BFs prepared by different steam explosion processes is shown in . When the solid–liquid ratio was 1:1 and 1:2, the fibers were tightly connected to each other in a fibrous bundle under all conditions except C9. The fiber cross-section of C9 separated part of the single fiber, which may be due to the fact that when the steam explosion pressure increased, the penetration of steam into the pores between fibers became larger. As a result, the connection between fibers was weakened, causing separation upon instantaneous pressure relief. At the solid–liquid ratio of 2:1, the cross-section separation of BFs was significantly improved compared to other solid–liquid ratios. The connection between the fibers was weakened and the fiber bundles separated into single fibers at the explosion pressure of 1.8 MPa and 2.2 MPa, which indicated that the solid–liquid ratio and improvement of explosion pressure had a great effect on the fiber separation. Consequently, the steam explosion process could be utilized to regulate the morphology of BFs by manipulating the solid–liquid ratio and pressure.
Physicochemical characteristic analysis
As shown in , there was an evident band at 1730 cm−1, which was attributed to the C=O stretching vibration assigned to hemicellulose and lignin (Tanpichai et al. Citation2019). The disappearance of the band at 1730 cm−1 in the BFs at the explosion holding time of 7 min indicated that hemicellulose and lignin had been partially removed. The absorption peak at 1509 cm−1 was C=C aromatic symmetric stretching and at 1460 cm−1 was C–H bending vibration, and the carbon skeleton peaks were almost unaffected by the parameter changes (Fan, Dai, and Huang Citation2012). The peak at 1240 cm−1 was attributed to C–C, C–O, and C=O stretching vibrations, and G ring stretching. As the explosion holding time reached 7 min and the explosion pressure reached 2.2 MPa, the peak disappeared, indicating the partial removal of hemicellulose and lignin (Maache et al. Citation2017; Rocky and Thompson Citation2021). The pressure and time have a significant impact on the degree of steam explosion, promoting the removal of chemical components and thereby benefiting the preparation of fibers. The band at 895 cm−1 represents the characteristic peak of cellulose, attributed to COC, CCO, and CCH deformation and stretching (Fan, Dai, and Huang Citation2012). The peak here gradually disappeared at the explosion holding time of 7 min, indicating partial degradation of cellulose.
The XRD pattern of BFs prepared by different steam explosion processes is shown in . There are three characteristic peaks in XRD patterns of each BF, located at approximately 15.8°, 22.1°, and 34.7°, which correspond to a composite of both (1–10) and (110), (200), and (004) crystalline planes, indicating the presence of typical cellulose I (French Citation2014). The XRD patterns of BFs prepared by different steam explosion processes were similar, suggesting that the crystalline structure was almost retained regardless of explosion pressure and holding time. The crystallinity of BFs was high at the solid–liquid ratio of 2:1. Explosion pressure is also one of the factors affecting the crystallinity of BFs. The crystallinity increased with the increase of steam explosion pressure, as part of the hydrogen bond broken in the process of steam explosion, which destroyed the amorphous region. The structure of the crystallization region was very tight, and only a small amount of steam entered.
The moisture adsorption and desorption curves of different steam explosion BFs are shown in . All of the isotherms exhibited a typical S-shape and can be classified as type II. All adsorption isotherms bend upward at 70%, while desorption isotherms are linear at similar relative humidity levels. At low relative humidity, chemical attraction is the dominant mechanism for sorption, and water is directly bound to hydroxyl groups in the cell wall. While at high relative humidity, physisorption is the dominant mechanism and water is absorbed by already hydrogen-bonded molecules to form clusters (Hartley, Kamke, and Peemoeller Citation1992; Zhang et al. Citation2018). Explosion pressure and holding time play a role in the moisture absorption of BFs, which decreases with increasing explosion pressure and holding time under the same humidity conditions, because hemicellulose has a large number of free hydroxyl groups, which makes it hydrophilic and has a higher capacity to absorb water (Kelly and Hart Citation1970; Kulasinski et al. Citation2016), while the increase in explosion pressure and holding time can remove more hemicellulose from the bamboo material, thus reducing the hygroscopicity of BFs. The equilibrium moisture content of C3 was lowest at 15% at the highest relative humidity (RH = 95%).
Figure 6. EMC values of BFs under different steam explosion processes plotted against relative humidity during (a) adsorption and (b) desorption.

In addition to the differences in equilibrium water content (EMC) values, there were also differences in the degree of hysteresis. Absolute hysteresis and the hysteresis coefficient are two common means to characterize sorption hysteresis (Olek, Majka, and Czajkowski Citation2013). The hysteresis and hysteresis coefficients for BFs with different steam explosion processes are shown in . BFs exhibited the hysteresis phenomenon over the entire relative humidity region, but the degree of hysteresis varied. Hysteresis for all BFs increased first and then decreased with the increase in relative humidity, which can be attributed to hemicellulose softening (Hou et al. Citation2022). The highest hysteresis values were reached at 70% relative humidity and varied between 3.26% and 4.14% for BFs with different steam explosion processes. The hysteresis coefficient varied significantly (0.37–0.91) for BFs under different steam explosion processes. The absolute hysteresis phenomenon was significantly weakened with the increase of explosion pressure and holding time, which was related to the change in chemical composition. Combined with the results of chemical composition analysis, the increase in explosion pressure and holding time led to a deeper degree of steam explosion, which promoted the removal and degradation of lignin and hemicellulose. Lignin and hemicellulose contents are positively correlated with absolute hysteresis. Higher lignin content leads to more absolute hysteresis in the bamboo cell wall; lignin has many unsaturated groups in its molecular structure, which reduces the flexibility of the lignin molecule and increases hysteresis. Moreover, the complex network formed between hemicellulose and lignin limits the mobility of hemicellulose, and the molecular chains cannot promptly arrange to accommodate the ingress and egress of water molecules, ultimately leading to heightened absolute hysteresis (Hill, Norton, and Newman Citation2009; Hou et al. Citation2022). The depth of the steam explosion was greatest when the pressure reached 2.2 MPa and the time reached 7 min. Additionally, the removal of lignin and hemicellulose led to a substantial reduction in hysteresis, thereby significantly weakening the hysteresis phenomenon.
Thermal properties
As shown in , there were three distinct weight loss stages for BFs under different steam explosion processes at 25–150°C, 200–400°C, and 400–800°C, respectively. The first weight-loss stage was mainly attributed to the evaporation of water and some extractions from the fiber, with negligible mass loss at the beginning of different steam exploded BFs. The TGA curves showed the decomposition of hemicellulose and subsequent cellulose decomposition at this stage (Zakikhani et al. Citation2016). The second weight-loss stage at 200–400°C was mainly assigned to the breaking of glycosidic bonds and unstable cellulose in the hemicellulose structure of BFs and the decomposition of some lignin oxidation. The degradation of cellulose in BFs was at 216°C to 273°C, the degradation of cellulose took place at higher temperatures and was more stable compared to hemicellulose due to its complex structure. The third weight loss stage is mainly the pyrolysis of lignin (AN and N Citation2017). After the degradation of cellulose, the degradation of lignin occurred between 273°C and 405°C. Lignin is a component containing aromatic compounds, unlike hemicellulose and cellulose, which are the main components of the cell wall (Peng et al. Citation2012). It has a complex structure that binds hemicellulose and cellulose together. Therefore, the breakdown of lignin is slow and more difficult throughout the temperature (Alwani et al. Citation2014). The increased mass loss during the third stage of decomposition leads to reduced thermal stability due to the greater amount of degraded lignin. The Tmax referred to the decomposition temperature corresponding to the maximum weight loss and related to maximum decomposition, exhibited slight variations among the different steam explosion processes for the biomass fuels (). This discrepancy may be attributed to differences in the parenchyma cells. However, the carbon residues were similar, suggesting that the thermal stability properties of the biomass fuels did not significantly differ under varying steam explosion conditions.
Table 5. Thermogravimetric data of BFs under different steam explosion processes.
Conclusions
Different steam explosion processes were employed to prepare BFs, and the influence of various parameters such as solid–liquid ratio, explosion pressure, and explosion holding time was investigated. The findings indicated that the combination of these methods yielded fine fibers with desirable morphology. The optimal response was achieved with a fiber yield of 39.07%, using a solid–liquid ratio of 2:1, an explosion pressure of 2.2 MPa, and an explosion holding time of 4 min. At this solid–liquid ratio, the fiber bundles were partially separated, resulting in the formation of single fibers. This emphasizes the significance of the solid–liquid ratio in influencing the degree of separation of the BFs. Additionally, both an increase in explosion pressure and holding time, particularly when the explosion pressure was set to 2.2 MPa, resulted in a higher degree of separation. By manipulating the steam explosion process parameters, the morphology of BFs could be controlled. Furthermore, through the combined effect of pretreatment and steam explosion, part of the hemicellulose and lignin was removed without altering the crystalline structure of BFs. The hygroscopicity of BFs was significantly reduced with the optimal process yielding a minimum hygroscopicity of 15%. Finally, the thermal stability properties of BFs showed similarity under different steam explosion processes. Determining the optimal steam explosion parameters is beneficial for the industrial production of fine fibers, and contributes to the development and application of BF composites.
Highlights
Solid–liquid ratio, explosion pressure, and explosion holding time have significant effects on the preparation of bamboo fibers by steam explosion.
Optimization of the process parameters of steam explosion to improve the yield of bamboo fibers for composites.
Characterization of the morphology, chemical composition, and physical and thermal properties of bamboo fibers prepared under different levels of steam explosion.
Author contributions
Wenjuan Zhao: Investigation, Writing – original draft. Yanping Zou: Investigation, Writing – review & editing. Wenfu Zhang: Formal analysis, Conceptualization. Hong Chen: Validation, Conceptualization. Jian Zhang: Supervision. Anqi Wu: Data curation. Shaofei Yuan: Methodology, Supervision. Ying Zhao: Investigation. All authors read and approved the final manuscript.
Acknowledgments
The work was supported by the National Key R&D Program of China [No. 2023YFD2202100], the “Pioneer” and “Leading Goose” R&D·Program of Zhejiang [No. 2022C04019].
Disclosure statement
The authors declare that they have no known competing financial interests or personal relationships that could have appeared to influence the work reported in this study.
Data availability statement
The data that support the findings of this study are available from the corresponding author upon reasonable request.
Additional information
Funding
References
- Alwani, M. S., H. P. S. Abdul Khalil, O. Sulaiman, M. N. Islam, and R. Dungani. 2014. “An Approach to Using Agricultural Waste Fibres in Biocomposites Application: Thermogravimetric Analysis and Activation Energy Study.” BioResources 9 (1): 218–15. https://doi.org/10.15376/biores.9.1.218-230.
- AN, B., and K. J. N. 2017. “Characterization of Alkali Treated and Untreated New Cellulosic Fiber from Saharan Aloe Vera Cactus Leaves.” Carbohydrate Polymers 174:200–208. https://doi.org/10.1016/j.carbpol.2017.06.065.
- Chen, X., F. Chen, G. Wang, X. Ma, J. Wang, and J. Deng. 2022. “Eco-Friendly, Disposable Bamboo Fiber Dishware: Static and Dynamic Mechanical Properties and Creep Behavior.” Industrial Crops & Products 187:115305. https://doi.org/10.1016/j.indcrop.2022.115305.
- Chen, H., J. Wu, J. Shi, W. Zhang, and G. Wang. 2021. “Strong and Highly Flexible Slivers Prepared from Natural Bamboo Culm Using NaOh Pretreatment.” Industrial Crops & Products 170:113773. https://doi.org/10.1016/j.indcrop.2021.113773.
- Chen, J. H., J. K. Xu, P. L. Huang, and R. C. Sun. 2016. “Effect of Alkaline Pretreatment on the Preparation of Regenerated Lignocellulose Fibers from Bamboo Stem.” Cellulose 23 (4): 2727–2739. https://doi.org/10.1007/s10570-016-0983-1.
- Fan, M., D. Dai, and B. Huang. 2012. “Fourier transform infrared spectroscopy for natural fibres.” Intech 3:45–68. https://doi.org/10.5772/35482.
- French, A. D. 2014. “Idealized Powder Diffraction Patterns for Cellulose Polymorphs.” Cellulose 21 (2): 885–896. https://doi.org/10.1007/s10570-013-0030-4.
- Gao, X., D. Zhu, S. Fan, M. Z. Rahman, S. Guo, and F. Chen. 2022. “Structural and Mechanical Properties of Bamboo Fiber Bundle and Fiber/Bundle Reinforced Composites: A Review.” Journal of Materials Research and Technology 19:1162–1190. https://doi.org/10.1016/j.jmrt.2022.05.077.
- Hartley, I. D., F. A. Kamke, and H. Peemoeller. 1992. “Cluster Theory for Water Sorption in Wood.” Wood Science and Technology 26 (2): 83–99. https://doi.org/10.1007/BF00194465.
- Hill, C. A., A. Norton, and G. Newman. 2009. “The Water Vapor Sorption Behavior of Natural Fibers.” Journal of Applied Polymer Science 112 (3): 1524–1537. https://doi.org/10.1002/app.29725.
- Hongzhang, C., and L. Liying. 2007. “Unpolluted Fractionation of Wheat Straw by Steam Explosion and Ethanol Extraction.” Bioresource Technology 98 (3): 666–676. https://doi.org/10.1016/j.biortech.2006.02.029.
- Hou, S., J. Wang, F. Yin, C. Qi, and J. Mu. 2022. “Moisture Sorption Isotherms and Hysteresis of Cellulose, Hemicelluloses and Lignin Isolated from Birch Wood and Their Effects on Wood Hygroscopicity.” Wood Science and Technology 56 (4): 1087–1102. https://doi.org/10.1007/s00226-022-01393-y.
- Hu, J., J. Y. Wu, Y. X. Huang, Y. Q. He, J. Lin, Y. M. Zhang, Y. H. Zhang, Y. L. Yu, and W. J. Yu. 2023. “Super-Strong Biomimetic Bulk Bamboo-Based Composites by a Neural Network Interfacial Design Strategy.” Chemical Engineering Journal 475:146435. https://doi.org/10.1016/j.cej.2023.146435.
- Kelly, M. W., and C. A. Hart. 1970. “Water Vapor Sorption Rates by Wood Cell Walls.” Wood and Fiber Science 1 (4): 270–282.
- Kulasinski, K., L. Salmén, D. Derome, and J. Carmeliet. 2016. “Moisture Adsorption of Glucomannan and Xylan Hemicelluloses.” Cellulose 23 (3): 1629–1637. https://doi.org/10.1007/s10570-016-0944-8.
- Lin, Q. Q., R. N. Gao, D. H. Li, Y. Lu, S. Q. Liu, Y. Yu, Y. X. Huang, and W. J. Yu. 2022. “Bamboo-Inspired Cell-Scale Assembly for Energy Device Applications.” Npj Flexible Electronics 6 (1): 13. https://doi.org/10.1038/s41528-022-00148-w.
- Liu, D., J. Song, D. P. Anderson, P. R. Chang, and Y. Hua. 2012. “Bamboo Fiber and Its Reinforced Composites: Structure and Properties.” Cellulose 19 (5): 1449–1480. https://doi.org/10.1007/s10570-012-9741-1.
- Maache, M., A. Bezazi, S. Amroune, F. Scarpa, and A. Dufresne. 2017. “Characterization of a Novel Natural Cellulosic Fiber from Juncus Effusus L.” Carbohydrate Polymers 171:163–172. https://doi.org/10.1016/j.carbpol.2017.04.096.
- Olek, W., J. Majka, and Ł. Czajkowski. 2013. “Sorption Isotherms of Thermally Modified Wood.” Holzforschung 67 (2): 183–191. https://doi.org/10.1515/hf-2011-0260.
- Peng, P., F. Peng, J. Bian, F. Xu, and R. C. Sun. 2012. “A Comparative Study of Bamboo (Phyllostachys Incarnata Wen) Milled Wood Lignin and the Successively Alkali-Fractionated Lignins.” Wood Science and Technology 46 (5): 871–885. https://doi.org/10.1007/s00226-011-0451-x.
- Rocky, B. P., and A. J. Thompson. 2018. “Production of Natural Bamboo Fibers-1: Experimental Approaches to Different Processes and Analyses.” The Journal of the Textile Institute 109 (10): 1381–1391. https://doi.org/10.1080/00405000.2018.1482639.
- Rocky, B. P., and A. J. Thompson. 2021. “Analyses of the Chemical Compositions and Structures of Four Bamboo Species and Their Natural Fibers by Infrared, Laser, and X-Ray Spectroscopies.” Fibers and Polymers 22 (4): 916–927. https://doi.org/10.1007/s12221-021-0303-8.
- Segal, L., J. J. Creely, A. E. Martin, and C. M. Conrad. 1959. “An Empirical Method for Estimating the Degree of Crystallinity of Native Cellulose Using the X-Ray Diffractometer.” Textile Research Journal 29 (10): 786–794. https://doi.org/10.1177/004051755902901003.
- Shi, J., W. Zhang, S. Gu, S. Yuan, and J. Zhang. 2023. “Physical and Mechanical Properties of Bamboo Fiber/Glass Fiber Mesh Reinforced Epoxy Resin Hybrid Composites: Effect of Fiber Stacking Sequence.” Journal of Natural Fibers 20 (1):2167145. https://doi.org/10.1080/15440478.2023.2167145.
- Song, Y., G. Han, M. Li, W. Jiang, X. Li, Y. Zhang, and R. Yu. 2017. “Performance Analysis of Kudzu Fiber Prepared by Using Combined Steam Explosion and Chemical Degumming.” Journal of Natural Fibers 14 (6): 759–768. https://doi.org/10.1080/15440478.2016.1212770.
- Sun, S. L., J. L. Wen, M. G. Ma, and R. C. Sun. 2014. “Enhanced Enzymatic Digestibility of Bamboo by a Combined System of Multiple Steam Explosion and Alkaline Treatments.” Applied Energy 136:519–526. https://doi.org/10.1016/j.apenergy.2014.09.068.
- Tanpichai, S., S. Witayakran, Y. Srimarut, W. Woraprayote, and Y. Malila. 2019. “Porosity, Density and Mechanical Properties of the Paper of Steam Exploded Bamboo Microfibers Controlled by Nanofibrillated Cellulose.” Journal of Materials Research and Technology 8 (4): 3612–3622. https://doi.org/10.1016/j.jmrt.2019.05.024.
- Yuan, Z., G. Li, W. Wei, J. Wang, and Z. Fang. 2020. “A Comparison of Different Pre-Extraction Methods Followed by Steam Pretreatment of Bamboo to Improve the Enzymatic Digestibility and Ethanol Production.” Energy 196:117156. https://doi.org/10.1016/j.energy.2020.117156.
- Zakikhani, P., R. Zahari, H. Sultan, and M. T. Majid. 2016. “Thermal Degradation of Four Bamboo Species.” BioResources 11 (1): 414–425. https://doi.org/10.15376/biores.11.1.414-425.
- Zakikhani, P., R. Zahari, M. T. H. Sultan, and D. L. Majid. 2014. “Extraction and Preparation of Bamboo Fibre-Reinforced Composites.” Materials & Design 63:820–828. https://doi.org/10.1016/j.matdes.2014.06.058.
- Zhang, X., J. Li, Y. Yu, and H. Wang. 2018. “Investigating the Water Vapor Sorption Behavior of Bamboo with Two Sorption Models.” Journal of Materials Science 53 (11): 8241–8249. https://doi.org/10.1007/s10853-018-2166-y.
- Zhao, W., Y. Zou, W. Zhang, and H. Chen. 2023. “Effect of Fiber Separation Degree on the Properties of Bamboo Fiber Composites.” European Journal of Wood and Wood Products 81 (5): 1249–1259. https://doi.org/10.1007/s00107-023-01928-5.