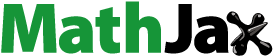
ABSTRACT
Cotton is a crucial raw material for the textile industry, and the quality of its initial product greatly affects the properties of the yarn and fabric. However, the impact of low temperature and moisture regain during ginning on the mechanical properties has not been extensively studied. This paper aims to address this gap by designing and analyzing a strategy for evaluating the mechanical properties of machine-harvested cotton fibers pretreated at different moisture regain levels and temperatures. Regression models for the relationship between temperature and mechanical properties under different moisture regain conditions were also established, showing good consistency. Additionally, the study examines the mechanical damage mechanism of cotton fibers during the harvesting and ginning process by observing the fiber fracture interface of different samples. Especially, those with low moisture regain exhibit a V-type fracture, while those with high moisture regain display significant fibrils exposed on the fiber body, resulting in a tearing morphology at the fracture interface. In conclusion, this study provides theoretical support for optimizing the harvesting process in cotton processing enterprises in terms of moisture regain and low temperature and makes a significant toward sustainable cotton fiber harvesting and processing technology.
摘要
棉花是纺织工业的重要原材料,其初始产品的质量极大地影响纱线和织物的性能。然而,轧棉过程中的低温和回潮对机械性能的影响尚未得到广泛研究。本文旨在通过设计和分析评估在不同回潮率水平和温度下预处理的机采棉纤维的机械性能的策略来解决这一差距。还建立了不同回潮率条件下温度与力学性能关系的回归模型,表现出良好的一致性。此外,该研究通过观察不同样品的纤维断裂界面来研究棉纤维在收获和轧花过程中的机械损伤机制。特别是,回潮率低的纤维表现出V型断裂,而回潮率高的纤维则表现出明显的原纤暴露在纤维体上,导致断裂界面处出现撕裂形貌。综上所述,本研究为棉花加工企业在回潮率和低温等方面优化采收流程提供了理论支撑,对可持续棉纤维采收加工技术具有重要意义。
Introduction
Cotton is a crucial component of our daily lives, directly impacting the comfort and durability of our clothing. The quality of cotton has always been a topic of concern. The ginning process, an essential part of cotton production, reflects the initial quality of cotton fiber. Despite advancements in ginning technology and equipment, cotton fiber defects caused by environmental factors and equipment strikes still need attention. Therefore, it is crucial to control temperature and moisture levels during harvesting and ginning (Andrews, Honold, and Grant Citation1963; Barker et al. Citation2001).
China is a major cotton producer and plays a significant role in the international market. The expansion of cotton planting areas requires more labor for cotton picking. However, the shortage of labor and high costs has become bottle-necks hindering the development of the cotton industry. To address this issue, mechanical cotton picking has been implemented since 1996, with Xinjiang popularizing it in 2001. By 2020, over 67.5% of cotton in Xinjiang was machine-harvested, and in 2021, the area exceeded 20,000 km2, with a machine-harvested rate exceeding 80%. In 2022, this rate further increased to 94%. Machine-harvested solves problems such as low efficiency, waste, and the need for manual picking of different-colored fibers (Cao and Hong Citation2022; Cao et al. Citation2021; Wang and Hong Citation2011). However, machine-harvested cotton fiber has a high impurity content, requiring increased cleaning during the ginning process to improve lint cotton quality (Bel et al. Citation1991; Li et al. Citation2012). Research has shown that each lint cleaning process reduces the length of cotton fiber by about 0.2–0.5 mm and decreases the content of short fibers by 0.8–1.5% (Xu et al. Citation2014). Additionally, the number of neps increases exponentially, and the mechanical properties of fibers are damaged (Tian et al. Citation2017; M. H. Van der Sluijs Citation2015). Temperature and moisture regain also significantly impact the properties of cotton fiber. Temperature is a crucial ecological factor that limits cotton yield and quality (Haigler et al. Citation1991; Martin and Haigler Citation2004). Decreased night temperatures slow down cellulose synthesis (Gipson and Joham Citation1969b), resulting in underdeveloped fibers with poor physical and chemical properties (Gipson and Joham Citation1968, Citation1969a). Excessive drying temperatures reduce the moisture regain of cotton fiber, making it brittle and prone to breakage. Therefore, the ginning process temperature should be controlled between 15°C-35°C to ensure the length and mechanical properties of the fiber (Andrews, Honold, and Grant Citation1963; Barker et al. Citation2001; Leitgeb and Wakeham Citation1954). Moisture regain also fluctuates during the ginning process, and these changes can affect the cellulose structure (Andrews, Honold, and Grant Citation1963). Ginning at moisture levels below or above 12% can cause fiber damage, neps, and a reduction in color grade (Skelton Citation1975; M. H. J. Van der Sluijs Citation2021; M. H. Van der Sluijs and Delhom Citation2017; M. H. J. Van der Sluijs and Long Citation2016). Thus, maintaining an optimum moisture regain range of 6%-8% during the ginning process ensures fiber quality (Chun and Brushwood Citation1998; Lawson, Ramey, and Krowicki Citation1976; Pandey and Iyengar Citation1970; Skelton Citation1975). While extensive research has been conducted on the ginning process in typical environments to ensure the initial quality of the fiber, further investigation is needed to understand how cotton fiber is mechanically damaged in low-temperature, high-moisture conditions. Field investigations and research have shown that fiber damage is a concern when the temperature drops below 0°C and reaches −20°C with rain, snow, high moisture regain (>12%), and freezing conditions (Cao and Hong Citation2022; Cao et al. Citation2021). Therefore, more research is required to address the issue of mechanical damage to cotton fibers during processing under low-temperature and high-moisture conditions.
Heretofore, there has been limited published research on the synergistic effects of low temperature and moisture regain on cotton fibers. This makes it challenging to analyze the reduction of mechanical properties and damage to fibrils in machine-harvested cotton at low temperatures. In this study, we conducted research at temperatures below 0°C, with moisture regain levels of 20% and 3.5% for high and low moisture regions, respectively. Through an innovative experimental design involving temperature and moisture regain, we attempted to establish a strength prediction model at low temperatures using MATLAB. The results of these experiments will help assess and quantify the impact of environmental conditions during the ginning process on fiber quality in high-production systems. It is important to note that we combined the characteristics of a low temperature environment with cotton processing damage in actual production. By analyzing how the environment affects the mechanical qualities of cotton fiber, our findings provide valuable insights for the practice of cotton ginning processes.
Materials and methods
Experimental materials
For this study, machine-harvested cotton samples were obtained from cotton ginning enterprises, with a total fiber weight of 20 kg. The samples were divided into five groups, each containing 15 samples, and the cotton variety used was Xinluzao 13. The cotton fibers had a mean fineness of 1.55dtex, a mean fiber length of 28.9 mm, and single fiber fracture strength, elongation at break, and initial modulus values of 2.52 cN/dtex, 8.0%, and 0.99 cN/dtex, respectively. To avoid the impact of trash on the results, the trash was removed by manual selection methods. shows the breaking force curve of a single fiber, and the illustration depicts the fracture morphology of a fiber under an optical microscope. We used machine-harvested cotton single fiber samples to observe the SEM fracture interface at different temperatures and moisture regains.
Experimental method and process
All sample tests in this paper were conducted in a standard laboratory with a temperature of 20 ± 2°C and a relative humidity of 65 ± 5%. The experimental steps are illustrated in . Firstly, impurities and free fibers were removed from the sample fibers of the machine-harvested cotton, and the cotton bundles were straightened. Then, 30 mg cotton bundles were evenly formed on one end of the fiber. Five sample numbers were assigned: normal temperature high moisture regains (N-HMR), normal temperature low moisture regains (N-LMR), low temperature high moisture regains (L-HMR), low temperature low moisture regains (L-LMR), and normal control sample. Each sample consisted of 15 samples. Next, the samples underwent humidification pre-treatment. Based on the experimental requirements, the actual moisture regain was initially tested, and the samples were divided into two groups: low moisture regain and high moisture regain. The high moisture regain samples were pre-humidified. The actual moisture regain of machine-harvested cotton samples from the initial cotton enterprises ranged from 4% to 5%, which falls under the low moisture regain category. Therefore, the low moisture regain was set to 3.5% using an oven. In accordance with actual production conditions, the moisture regain of cotton fiber can reach 20% in the case of snow during cold winters. Thus, the moisture regain of cotton fiber samples was pre-treated to 20%. All experimental samples were placed in a sample bag in the laboratory for 48 hours to achieve moisture absorption balance. The third step involved low temperature treatment. The L-HMR and L-LMR samples from the previous step were placed in refrigerators set to different low temperatures for 24 hours. The temperatures used were −20°C, −15°C, −10°C, −5°C, and 0°C. Subsequently, different samples were subjected to hooking, twisting, and bending using tweezers under the corresponding environmental conditions, including normal temperature N-HMR and N-LMR samples. Finally, the mechanical properties of the bundle fibers were tested in a standard laboratory, and single samples were taken out to characterize the fracture interface morphology using scanning electron microscopy.
Characterization
In this study, the mechanical properties of cotton fiber were mainly tested and the morphology of the fracture interface fiber was characterized. All fiber samples were tested in a standard laboratory environment, where the relative humidity and temperatures were 65 ± 5% and 20 ± 2°C, respectively. Using the calibration YG(B) 162D electron fiber strength meter, the mechanical properties of the cotton bundle fiber are measured, and the distance and the stretching rate are 10 mm and 10 mm/min, respectively. Bundle fiber test reference to GB1103.1–2012 Cotton Part One-Sawtooth Processing of Fine Staple Cotton, each sample was tested 15 times, and the results were averaged. The fracture interface morphology analysis of fibers was initially observed using XSP-3CA optical microscopy. Specifically, the fracture morphology of the as-prepared N-HMR, N-LMR, L-HMR, and L-LMR samples were characterized by scanning electron microscopy (SEM; TM3000, Hitachi Group, Japan).
Results and discussion
Results of mechanical properties of bundle fiber
The mechanical properties of the fibers were obtained using the YG(B) 162D system. presents the mechanical properties of the fibers under high moisture regain, low moisture regain, and normal moisture regain (65 ± 5%) at a normal temperature (20 ± 2°C).
Table 1. Tensile mechanical properties result in normal machine-harvested cotton fibers.
displays the changing trends of the main mechanical properties of machine-harvested cotton bundle fibers, including breaking force, fracture strength, and elongation at break, under different moisture regain levels and temperatures. These results will be introduced and analyzed in detail.
Table 2. Tensile mechanical properties result in low temperature machine-harvested cotton fibers.
Analysis of the breaking force of bundle fiber
The results in indicate a significant difference between the three moisture regain levels. The breaking force values of cotton fibers at normal temperatures were higher than those at low temperatures. Under the same normal temperature, the breaking force values decreased in the order of N-HMR > Normal > N-LMR. Additionally, under high moisture regain conditions, the breaking force of the N-HMR sample was 4731.08cN, which was 14.02% higher than that of the N-LMR sample. This demonstrates the influence of moisture regain on fiber strength. Furthermore, under the same moisture regain conditions, as shown in and , the breaking force of the L-LMR and L-HMR samples gradually increased with temperatures ranging from −20°C to 0°C, indicating an increase in the breaking capacity of cotton fibers. The breaking force of the L-LMR sample at −20°C was 3002.66cN, which was 30.6% lower than that of the control sample. This suggests that low temperatures can weaken the fiber structure and cause molecular conformational changes and slippage. These findings are consistent with previous studies that have shown higher moisture levels increase mechanical properties (Hamann Citation2012; Jaime, McKamey, and Cotty Citation2013). Therefore, the impact of low temperatures on the tensile strength of cotton fibers should not be overlooked. To better understand the changing trend of machine-harvested cotton bundle fibers, a second-order polynomial fitting collaboration figure was used to simulate the consequences of various moisture regain and low temperature settings. As shown in , the effect of temperature on strength was fitted for the L-LMR and L-HMR samples, and the fitted curve showed good consistency with the experimental values, with R2 values of 91.17% and 93.24% for the L-HMR and L-LMR samples, respectively. These results indicate that the fitting equation has good predictability for the experiment.
Figure 3. Fiber breaking force curve of machine-harvested cotton in low temperature environment. (a) L-HMR fiber experimental curve and fitting curve, (b) L-LMR fiber experimental curve, and fitting curve.

As shown in , in both L-HMR and L-LMR the fitting equation R2 were exceed 90%, which shows that the breaking force has a quadratic function relationship with the temperature of −20°C, −15°C, −10°C, −5°C and 0°C. Herein, assuming that the breaking force is y, x1 represents the low temperature, and the values are −20°C, −15°C, −10°C, −5°C, and 0°C, respectively. x2 is also a variable representing high and low moisture regain of 20% and 3.5%, respectively.
The multivariate nonlinear regression model of EquationEq. (1)(1)
(1) is used to establish the breaking strength models of different moisture regains at low temperatures:
Use the command regress of the MATLAB statistical toolbox to solve the problem, and the format is: [b, bint, r, rint, stats] =regress (y, x, α)
Where y is the breaking force x is the data matrix [1,x1,x12,x2] of the regression coefficient a=(a0,a1,a2,a3), α is the confidence level (default is 0.05), the output b is the estimated value of the coefficient a, bint is the confidence interval of b, r is the residual vector, rint is the confidence interval of r, stats is the test statistic of the regression model, the first value is the coefficient of determination R2 of the regression equation, the second value is the F statistic, and the third value is the probability value p corresponding to the F statistic.
By running MATLAB, we get the running results of the parameter values in , and also obtain the model determination coefficient R2, statistic F, and the corresponding p-value, which are R2 = 0.93, F = 28.64, and p = 5.9 × 10−4 <0.05, respectively. The analysis of the model results shows that R2 = 0.93 means that 93% of the dependent variable y can be determined by the model, the F-value is much larger than the critical value, and the p-value is much smaller than 0.05, so the model is available.
Table 3. MATLAB parameters and results.
Therefore, the regression model of the breaking force of machine-harvested cotton is obtained as:
The model in EquationEq. (2)(2)
(2) can predict all situations including the breaking force of machine-harvested cotton fibers in at different moisture regains and temperatures. When the temperature changes, the values in the model relationship also change. shows the confidence degree is 97.5%, which indicates the confidence level of the conclusion of the sample, within the confidence interval [3778.084 4210.18, 26.78 111.72].
Analysis of the fracture strength of bundle fibers
The relationship between the single fiber strength and the average bundle fiber strength is measured by the fiber strength tester as follows (Fang Citation2018; J. W. Hearle and Morton Citation2008; Yu Citation2018):
In the EquationEq. (3)(3)
(3) :
and
is the average force of the fibers bundle and single fiber, respectively, the unit is cN; n is the number of fibers in the bundle; NtB and Ntf are the linear density of bundle and single fibers, respectively, the unit is tex, k is the coefficient of fiber, under the standard conditions of China, the coefficient is 1.412–1.481.
Based on the reference standard for bundle fiber strength “GB1103.1–2012 Cotton Part 1-Sawtooth Processing Fine Staple Cotton,” the fracture strength is divided into five grades. By using EquationEq. (3)(3)
(3) and the fracture strength data in , the strength and elongation test values of different bundle fibers were obtained, and the fracture strength of machine-harvested cotton bundle fiber samples was graded. At normal temperature, the fracture strength of the high moisture regain sample was 3.11cN/tex greater than that of the low moisture regain sample, while the control sample fracture strength was 1.36cN/tex higher. All of the samples met the C1 “Very strong” grade at standard temperature, indicating a higher fracture strength compared to low temperature environments. The fracture strength of the L-LMR sample at −20°C was less than 24 cN/tex, classified as “Very Poor” C5 grade. However, as the temperature increased from −20°C to 0°C, the fracture strength grades of the L-HMR and L-LMR samples improved. At 0°C, the fracture strength of the L-LMR sample reached 31.37cN/tex, categorized as “Very strong.” The L-LMR sample’s fracture strength value was also 2.5% higher than that of the L-HMR sample (30.62cN/tex). At −10°C, the fracture strength of the L-HMR sample (25.35cN/tex) was C4, and that of the L-LMR sample (26.57cN/tex) was C3. Under high moisture regain conditions, the fracture strength value decreased. At −20°C and −15°C, the L-LMR sample was lower than the L-HMR sample, possibly due to increased brittleness caused by low temperature and high moisture regain. When the temperature increased from −10°C to 0°C, the fracture strength of both L-HMR and L-LMR samples increased rapidly, indicating that low temperature led to a decrease in the force strength of the fiber. In particular, water freezing is the process of ice crystallization from supercooled water (Zhang and Liu Citation2018), and the mechanical damage treatment results were observed at normal temperature and were minor compared to low temperature conditions. This could be explained by the ice-crystals sandwiched between macromolecules destroying the fibrils in low temperature environments, especially in the case of high moisture regain. Another reason is that as the temperature increases, the conformation of macromolecules changes, resulting in an increase in the number of macromolecular chains that can effectively carry stress.
Analysis of elongation at break of bundle fibers
The mechanical parameters in were used to assess the impact of low temperature and moisture regain on elongation at break. The quantitative indicator of elongation at break for HVI bundle fibers was used. At normal temperature, the N-HMR sample was 0.7% longer than the N-LMR sample, while the normal control sample was 7.7% longer than the N-LMR sample and closer to its values (7.4%). All elongation at break measurements at standard temperature were classified as “High,” with N-HMR and N-LMR values higher than those measured in low temperature conditions. Below −15°C, the elongation at break falls into the “Low” range, indicating that the fiber is greatly impacted by significant moisture recovery. The elongation at break of the L-HMR and L-LMR samples transitions to “General” and “High” grades as the temperature increases from −10°C to 0°C, with values increasing as the temperature rises. This could be explained by water molecules entering the fiber and changing the binding state between the molecules of the fiber (Peirce Citation1929; Singh et al. Citation1969).
In order to express the trend of the elongation at break of the moisture regains in the temperature range of −20°C to 0°C, the regular trends of L-HMR and L-LMR samples are shown in .
Figure 5. Elongation at break variation trend of machine-harvested cotton in low temperature environment.

In , the elongation at break of the L-HMR sample and the L-LMR sample can be observed to change over time. When the ambient temperature rises from −20°C to 0°C, both the L-LMR and L-HMR samples show an increase in elongation at break, with the L-LMR sample exhibiting higher values than the L-HMR sample. The L-HMR sample is particularly unstable in low temperature environments, which may be attributed to the combination of low temperature and high moisture retention when water molecules penetrate the fiber interstices. Both the L-HMR and L-LMR samples exhibit significant dispersion in low temperature environments. These observations suggest that as temperature rises, macromolecules become more flexible, leading to higher moisture retention and increased intermolecular secondary valence. This, in turn, facilitates the loosening and slipping between molecular chains, resulting in greater elongation at break and more bond breakage (Lawson, Ramey, and Krowicki Citation1976).
Analysis of fracture interface of bundle fibers
The water exchange process significantly alters the physical characteristics of the fiber, which is consistent with the well-known ability of cotton fiber to interchange water with its surroundings. These findings are comparable to previous studies that have shown how relative moisture regain can have a significant impact on the fracture morphology of cotton fiber bundles (Cintrón and Ingber Citation2013), especially at extremely low temperatures and high water content. In this study, fractured samples were initially screened using an optical microscope, with 10 samples observed for each group. A representative sample was then selected for further characterization of the fracture morphology using SEM. This analysis helps validate and analyze the damage to the internal fibrils of the fibers in low temperature environments. The fracture interface morphology of machine-harvested cotton fibers is shown in .
Figure 6. Fracture morphologies of machine-harvested cotton fibers in different environments. (a) N-HMR sample fracture interface morphology, (b) N-LMR fracture interface morphology, (c) −20°C L-HMR sample fracture interface morphology, (d) −20°C L-LMR sample fracture interface morphology.

depicts the fracture interface morphology of the N-HMR fiber. Under the influence of stretch tension, the fiber exhibits a high degree of axial orientation, leading to a significant increase in the inherent crimp pitch of the cotton fiber. The broken fibrils in are identified as protruding from the fiber body, displaying uneven breakage and rips. These findings suggest that as moisture regain increases, the interactions between microfibers decrease, resulting in a large separation of fibrils at the fracture site. The fiber fracture is also highly uneven, with a rough surface and a shear-slip deformation zone (Sarna and Włochowicz Citation2003).
In , the fracture interface morphology of the N-LMR fiber is shown. This fiber, stretched under the same high degree of orientation as the N-HMR sample, serves as a reference. The fiber end surface splits due to a brittle fracture caused by the low moisture regain of the fiber, making it more prone to brittleness. According to the statistics in , which can be confirmed by observing the fiber shape, the high moisture regain strength is higher than the low moisture regain strength. Furthermore, there are obvious cracks on the fiber surface, and the fiber fracture section rarely shows fibril extraction. The central cavity of the fiber breaking interface is more apparent during the process of cotton bundle fiber breaking, along with the separation of fibrils. This breaking is not simultaneous and occurs on the same section. When the fibers are stretched and stretched, there are evident fibrils that separate from each other, leading to the formation of cracks (Zhao et al. Citation2007). These cracks may be caused by the low moisture regain and weak bonding between macromolecules.
shows the fracture interface morphology of L-HMR cotton fibers at −20°C. The enlarged image in the lower-left corner reveals twisted and fractured fibers, which align with previous studies by Professor Hearle (J. W. S. Hearle and Sparrow Citation1971, Citation1979). The fibrils and damaged fiber cavity, visible in the frame mark, exhibit the morphology of the fiber fracture interface. The fibrils are dislocated, the main body of the fiber is torn, and there are numerous scratches, splits, and tears in the area indicated by the arrow. Additionally, there are large cracks on the surface of the fiber, which are caused by the formation of ice crystals in low-temperature environments that scratch the internal fibrils of the fibers. Compared to the fiber fracture of L-LMR, the fracture interface is not as sharp.
displays the morphology of the fracture interface of the L-LMR fiber at −20°C. It is evident that the cotton fiber end fracture surface has a sharp V-shape and a cavity in the middle, with no obvious shrinkage on the cotton fiber surface. However, there are no obvious exposed fibrils in the main body of the fiber (Dweltz and Sparrow Citation1978). In a low-temperature and low moisture regain environment, the fiber morphology becomes dried out, indicating internal dehydration and a reduction in the interaction force between the fibrils.
Conclusion
It is widely recognized that cotton fibers collected by machines can be properly harvested at standard temperatures and moisture regains. Herein, the mechanical properties and microstructural damage of cotton fibers harvested by machines that were subjected to various moisture regains at different low temperatures were investigated. Cotton fibers mechanical properties decreased and the fibrils suffered damage at low temperatures, meanwhile with increasing temperature (−20°C to 0°C), the values of breaking force, fracture strength, and elongation at break all increased, which the trend was especially visible for the L-HMR samples. In addition, by fitting the breaking force of L-HMR samples and L-LMR samples, a multivariate nonlinear regression model relevant for the breaking force of machine-harvested cotton bunch fibers at different moisture regains and different low-temperatures was developed, and 93% of the dependent variables could be determined by this model. Moreover, a structural morphology investigation of the fiber fracture interface revealed that it had extremely consistent fracture morphology and pull-up properties throughout various moisture regains. In summary, it is crucial to take into account how a complex environment affects the quality of the fiber, and this study might offer cotton processors theoretical support and effective pre-control for ginning at various temperatures and moisture regains.
Highlights
Creative research on the properties of cotton fiber at extremely low temperatures. Specifically, it examines the impact of low-temperature processing and high humidity on the mechanical properties of cotton during the ginning process. It is well-known that cotton fibers are prone to damage in such environments. Therefore, this research proposes a fiber damage model at different low temperatures, aiming to provide primary processing enterprises in the global cotton industry with valuable insights into the environmental effects on the mechanical properties of cotton fibers. This data can serve as a practical reference for improving processing practices.
The breaking force of the regression model was established. Under the same normal temperature, the breaking force values decreased in the order of N-HMR > Normal > N-LMR. Additionally, the regression model of breaking force with machine-harvested cotton in low-temperature environments is obtained, the model confidence degree is 97.5%, which indicates the confidence level of the conclusion of the sample, within the confidence interval [3778.084 4210.18, 26.78 111.72].
Detailed morphological damage analysis of the fibrils fracture interface during low temperature environment. The ice-crystals sandwiched between macromolecules destroying the fibrils in low temperature environments, especially in the case of high moisture regain. Additionally, there are large cracks on the surface of the fiber, which are caused by the formation of ice crystals in low-temperature environments that scratch the internal fibrils of the fibers.
Author’s contributions
Jiqiang Cao: Methodology, Software, Testing, Data curation, Writing-Original draft preparation. Lu Cheng: Visualization, Investigation Testing. Limin Zhang: Data handling, sample analysis. Xiang Liu: Software, Supervision, Validation, Writing- Reviewing. Hong Xu: Conceptualization, Writing- Reviewing and Editing, Funding acquisition.
Consent to publish
I confirm that any participants (or their guardians if unable to give informed consent, or next of kin, if deceased) who may be identifiable through the manuscript (such as a case report), have been given an opportunity to review the final manuscript and have provided written consent to publish.
Ethical approval
I confirm that all the research meets ethical guidelines and adheres to the legal requirements of the study country.
Ethics declarations
I confirm that all the research meets ethical guidelines and adheres to the legal requirements of the study country.
Supplemental Material
Download MS Word (910.3 KB)Acknowledgments
We gratefully acknowledgements the financial support of the National Natural Science Foundation of China, the authors also thank College of Textile and Fashion, and the Ginning Company providing cotton sample, field investigation, and fiber testing.
Disclosure statement
No potential conflict of interest was reported by the author(s).
Supplemental data
Supplemental data for this article can be accessed online at https://doi.org/10.1080/15440478.2024.2309913.
Additional information
Funding
References
- Andrews, F. R., E. Honold, and J. N. Grant. 1963. “Heating, Cleaning, and Mechanical Processing Effects on Cotton: Part II: Equilibrium Moisture and Density.” Textile Research Journal 33 (8): 609–13. https://doi.org/10.1177/004051756303300803.
- Barker, G. L., J. W. Laird, M. G. Pelletier, and G. A. Holt. 2001. “Relative Velocity, Density, and Temperature Effects on Cotton Moisture Transfer Rates.” Journal of Cotton Science 5:243–251.
- Bel, P. D., E. P. Columbus, C. K. Bragg, and K. Q. Robert. 1991. “Effects of Mechanical Cleaning on Cotton Fibers: Part I: Ginning.” Textile Research Journal 61 (2): 83–88. https://doi.org/10.1177/004051759106100205.
- Cao, J., and X. Hong. 2022. “Development Status and Quality Improvement Countermeasure of Xinjiang Cotton.” Cotton Textile Technology 50 (6): 71–74.
- Cao, J., C. Lu, Z. Chunyu, Y. Shaoqi, and X. Hong. 2021. “Current Status and Countermeasures of Machine-Picked Cotton Primary Processing Research.” Cotton Textile Technology 49 (2): 75–79.
- Chun, D. T. W., and D. Brushwood. 1998. “High Moisture Storage Effects on Cotton Stickiness.” Textile Research Journal 68 (9): 642–648. https://doi.org/10.1177/004051759806800904.
- Cintrón, M. S., and B. F. Ingber. 2013. “Preliminary Examination of the Effects of Relative Humidity on the Fracture Morphology of Cotton Flat Bundles.” Textile Research Journal 83 (10): 1044–1054. https://doi.org/10.1177/0040517512470194.
- Dweltz, N. E., and J. T. Sparrow. 1978. “A SEM Study of Abrasion Damage to Cotton Fibers.” Textile Research Journal 48 (11): 633–636. https://doi.org/10.1177/004051757804801103.
- Fang, D. D. 2018. Cotton Fiber: Physics, Chemistry and Biology, 52–55. New Orleans, LA, USA: Springer.
- Gipson, J. R., and H. E. Joham. 1968. “Influence of Night Temperature on Growth and Development of Cotton (Gossypium birsutum L.). II Fiber Properties 1.” Agronomy Journal 60 (3): 296–298. https://doi.org/10.2134/agronj1968.00021962006000030015x.
- Gipson, J. R., and H. E. Joham. 1969a. “Influence of Night Temperature on Growth and Development of Cotton (Gossypium Hirsutum L.). III. Fiber Elongation 1.” Crop Science 9 (2): 127–129. https://doi.org/10.2135/cropsci1969.0011183X000900020004x.
- Gipson, J. R., and H. E. Joham. 1969b. “Influence of Night Temperature on Growth and Development of Cotton (Gossypium Hirsutum L.) IV. Seed Quality 1.” Agronomy Journal 61 (3): 365–367. https://doi.org/10.2134/agronj1969.00021962006100030008x.
- Haigler, C. H., N. R. Rao, E. M. Roberts, J.-Y. Huang, D. R. Upchurch, and N. L. Trolinder. 1991. “Cultured Ovules as Models for Cotton Fiber Development Under Low Temperatures.” Plant Physiology 95 (1): 88–96. https://doi.org/10.1104/pp.95.1.88.
- Hamann, M. T. 2012. Impact of Cotton Harvesting and Storage Methods on Seed and Fiber Quality. Texas, USA: Texas A & M University.
- Hearle, J. W., and W. E. Morton. 2008. Physical Properties of Textile Fibres, 276–280. Cambridge, England: Elsevier.
- Hearle, J. W. S., and J. T. Sparrow. 1971. “The Fractography of Cotton Fibers.” Textile Research Journal 41 (9): 736–749. https://doi.org/10.1177/004051757104100905.
- Hearle, J. W. S., and J. T. Sparrow. 1979. “Further Studies of the Fractography of Cotton Fibers.” Textile Research Journal 49 (5): 268–282. https://doi.org/10.1177/004051757904900505.
- Jaime, R., J. McKamey, and P. J. Cotty. 2013. “Module Storage Time, Leaf Grade and Seed Moisture Influence Fiber Quality and Aflatoxin Contamination of Cotton in South Texas.” Journal of Cotton Science 17 (1): 60–68.
- Lawson, R., H. H. Ramey, and R. S. Krowicki. 1976. “Cotton Fiber Tenacity and Elongation in Rapidly Changing Relative Humidity.” Textile Research Journal 46 (10): 715–719. https://doi.org/10.1177/004051757604601004.
- Leitgeb, D. J., and H. Wakeham. 1954. “Cotton Quality and Fiber Properties: Part III: Effects of Drying at High Temperatures Prior to Ginning.” Textile Research Journal 24 (12): 1047–1057. https://doi.org/10.1177/004051755402401203.
- Li, C., D. Thibodeaux, A. R. Knowlton, and J. Foulk. 2012. “Effect of Cleaning Treatment and Cotton Cultivar on Cotton Fiber and Textile Yarn Quality.” Applied Engineering in Agriculture 28 (6): 833–840. https://doi.org/10.13031/2013.42468.
- Martin, L. K., and C. H. Haigler. 2004. “Cool Temperature Hinders Flux from Glucose to Sucrose During Cellulose Synthesis in Secondary Wall Stage Cotton Fibers.” Cellulose 11 (3): 339–349. https://doi.org/10.1023/b:cell.0000046420.10403.15.
- Pandey, S. N., and R. L. N. Iyengar. 1970. “A Study on Wax Content and Its Relation to Moisture Regain and Tensile Strength of Cotton Fiber.” Textile Research Journal 40 (12): 1053–1058. https://doi.org/10.1177/004051757004001201.
- Peirce, F. T. 1929. “16—A Two-Phase Theory of the Absorption of Water Vapour by Cotton Cellulose.” Journal of the Textile Institute Transactions 20 (6): T133–T50. https://doi.org/10.1080/19447022908661486.
- Sarna, E., and A. Włochowicz. 2003. “Changes in the Superstructure and Surface Morphology of Cotton During Spinning.” Textile Research Journal 73 (3): 216–220. https://doi.org/10.1177/004051750307300305.
- Singh, B., C. L. Jain, A. Pande, and V. B. Chipalkatti. 1969. “Moisture Sorption of Cross-Linked Cotton Cellulose in Relation to Swelling.” Textile Research Journal 39 (12): 1117–1125. https://doi.org/10.1177/004051756903901207.
- Skelton, J. 1975. “Interfiber Forces During Wetting and Drying.” Science 190 (4209): 15–20. https://doi.org/10.1126/science.190.4209.15.
- Tian, J., X. Zhang, Y. Yang, S. Xu, W. Zuo, W. Zhang, H. Dong, X. Jiu, Y. Yu, and Z. Zhao. 2017. “How to Reduce Cotton Fiber Damage in the Xinjiang China.” Industrial Crops and Products 109:803–811. https://doi.org/10.1016/j.indcrop.2017.09.036.
- Van der Sluijs, M. H. 2015. “Impact of the Ginning Method on Fiber Quality and Textile Processing Performance of Long Staple Upland Cotton.” Textile Research Journal 85 (15): 1579–1589. https://doi.org/10.1177/0040517515569524.
- Van der Sluijs, M. H. J. 2021. “The Influence of Row Unit Settings and Ground Speed of Modern Spindle-Type Harvesters on Cotton Fibre and Seed Quality.” The Journal of the Textile Institute 112 (12): 1904–1912. https://doi.org/10.1080/00405000.2020.1848116.
- Van der Sluijs, M. H., and C. Delhom. 2017. “The Effect of Seed Cotton Moisture During Harvesting On: Part 2 Yarn and Fabric Quality.” Textile Research Journal 87 (15): 1841–1847. https://doi.org/10.1177/0040517516659381.
- Van der Sluijs, M. H. J., and R. L. Long. 2016. “The Effect of Seed Cotton Moisture During Harvesting on – Part 1 – Fiber Quality.” Textile Research Journal 86 (18): 1925–1936. https://doi.org/10.1177/0040517515617426.
- Wang, Z., and X. Hong. 2011. “Survey and Development Proposal of Machine-Picked Cotton in Xinjiang.” China Cotton 38 (6): 10–14.
- Xu, H., J. Cao, Y. Wei, and Z. Xie. 2014. “Influence of Saw Type Lint Cleaning on Performance of Machine Stripped Cotton.” Journal of Textile Research 35 (1): 35–39.
- Yu, W. 2018. Textile Materials Science, 109–115. Beijing: China Textile Press.
- Zhang, Z. S., and X. Y. Liu. 2018. “Control of ice nucleation: freezing and antifreeze strategies.” Chemical Society Reviews 47 (18): 7116–7139. https://doi.org/10.1039/c8cs00626a.
- Zhao, H. B., J. H. Kwak, Z. C. Zhang, H. M. Brown, B. W. Arey, and J. E. Holladay. 2007. “Studying Cellulose Fiber Structure by SEM, XRD, NMR and Acid Hydrolysis.” Carbohydrate Polymers 68 (2): 235–241. https://doi.org/10.1016/j.carbpol.2006.12.013.