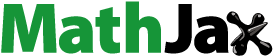
ABSTRACT
Obtaining suitable reinforcement of the polymer matrix depends largely on the appropriate connection at the fiber/polymer interface. Therefore, the main aim of the research was to assess the impact of silanization treatment of Himalayan nettle fibers on the properties of bio epoxy composites. Two types of silanes with different functional groups were used: 3-chloropropylmethyldimethoxysilane and N-(2-aminoethyl)-3-aminopropyltrimethoxysilane at concentrations of 1 and 2%. The effectiveness of the fiber silanization process was confirmed using Fourier transform infrared spectroscopy (FTIR) and scanning electron microscopy (SEM). It was found that treatment with silane with amine groups provided more effective adhesion between the fiber and the epoxy matrix than treatment with silane with chlorine groups. In addition, the thermal stability, impact strength, bending strength, impact resistance, density and water absorption of the composites were determined. It should be emphasized that silane treatment had a positive effect on the impact strength, impact resistance and flexural modulus of the tested layered composites.
摘要
聚合物基体的适当增强在很大程度上取决于纤维/聚合物界面处的适当连接. 因此,本研究的主要目的是评估喜马拉雅荨麻纤维的硅烷化处理对生物环氧复合材料性能的影响. 使用两种具有不同官能团的硅烷: 浓度为1%和2%的3-氯丙基甲基二甲氧基硅烷和N-(2-氨基乙基)-3-氨基丙基三甲氧基硅烷. 使用傅立叶变换红外光谱(FTIR)和扫描电子显微镜(SEM)证实了纤维硅烷化过程的有效性. 研究发现,与用具有氯基团的硅烷处理相比,用具有胺基的硅烷处理在纤维和环氧树脂基体之间提供了更有效的粘合. 此外,还测定了复合材料的热稳定性、冲击强度、弯曲强度、冲击强度和密度以及吸水率. 需要强调的是,硅烷处理对测试的层状复合材料的冲击强度、抗冲击性和弯曲模量有积极影响.
Introduction
Due to the growing consumption of functional and construction polymer materials obtained from nonrenewable sources, it becomes justified to design new, ecological composite materials. Hence, many scientific reports concern the analysis of the application potential of natural fibers and natural fillers of various origins as reinforcing materials in polymer composites (Andrzejewski et al. Citation2024; Aniśko and Barczewski Citation2023; Sanjay et al. Citation2018; Zaaba and Ismail Citation2019). Most often, glass or carbon fibers are used in combination with thermo- or chemically hardening resins. Therefore, it is important to show the wide possibilities and properties of plant fibers as an alternative to industrially produced glass or carbon mats and fabrics (Khan et al. Citation2022). Natural fibers can be made from plant stems, leaves or seeds and they contain mainly cellulose, hemicellulose, lignin, pectin and waxy substances (Bhattacharyya, Subasinghe, and Kim Citation2015; Karimah et al. Citation2021). Moreover, natural fibers are compostable, biodegradable, renewable, low density and cheap (Elfaleh et al. Citation2023). Therefore, designing construction materials using them brings not only utility benefits but also supports the protection of the natural environment (Kamarudin et al. Citation2022).
Epoxy resin, due to its favorable mechanical and thermal properties and ease of processing, is a good matrix for fiber-reinforced polymer composites (Barczewski et al. Citation2023; Jeyapragash, Srinivasan, and Sathiyamurthy Citation2020). A large group of engineering materials are layered composites, also known as laminates, used in the automotive, aviation and marine industries (Okafor et al. Citation2022). Birch, palm, and eucalyptus fibers were used to produce composites with good mechanical properties by resin transfer molding technique (Sarikaya, Çallioğlu, and Demirel Citation2019). Torres-Arellano et all. applied Ixtle and Henequen fiber (Asparagus family) and jute fiber (Corchorus olitorius) to produce biolaminates from biobased epoxy resin (Torres-Arellano, Renteria-Rodríguez, and Franco-Urquiza Citation2020). Chew et al studied flax-epoxy laminates and proved that helicoidal laminates were found to be more resistant to fiber damage as compared to cross-ply and quasi-isotropic laminates (Chew et al. Citation2021). Bambach described compression tests of geometrically identical structural channel sections fabricated from fiber-epoxy composites of flax, jute, hemp, glass and carbon (Bambach Citation2020). Prepreg sheets based on curaua fiber have been used to produce epoxy laminates with increased tensile and bending strength (Libera Junior et al. Citation2022).
Himalayan nettle fiber may be extracted by water, dew, controlled microbial retting, enzymatic treatment, and mechanical decortication methods. Cellulose (>86 wt.%) is the main constituent of this fiber (Mudoi, Sinha, and Parthasarthy Citation2023). Composites based on epoxy (Suarsana et al. Citation2021), poly (lactic) acid (PLA) (Fischer, Werwein, and Graupner Citation2012; Kumar and Das Citation2017), polyester (Mahendrakumar et al. Citation2015), and polypropylene (Paukszta et al. Citation2013) with nettle fiber have been successfully prepared and characterized. However, lignin, oils, and wax cover the fiber surface, making it highly hydrophilic and incompatible with hydrophobic polymer matrices (Doan, Brodowsky, and Mäder Citation2012). Chemical treatment techniques are based on the reaction of the functional groups of the agents used with natural fibers and enable the removal of non-cellulosic components from the fibers (Madhu, Sanjay, Jawaid, et al. Citation2020; Sanjay et al. Citation2019). Therefore, alkalization, mercerization, acrylation, acetylation, peroxide treatment, silanization and benzoylation are often used to prepare fiber before processing (Kenned, Sankaranarayanasamy, and Kumar Citation2021). It should be emphasized that treatment of lignocellulosic fibers allows the production of polymer composites with increased strength properties (Rangappa et al. Citation2022). Silane coupling agents are mainly used to improve the fiber/matrix interfacial adhesion and modify the fiber surface (Gieparda, Rojewski, and Różańska Citation2021). In order to improve the mechanical properties of the epoxy matrix, it is reinforced with fibers, which are often modified with a coupling agent. The novelty of this work is the comparison of the influence of two types of silanes with different functional groups, such as chloro and amine groups, used to modify nettle fabric on the properties of the bio epoxy composite.
The main aim of the research was to assess the influence of the silanization treatment of the fibers on the properties of epoxy composites reinforced with nettle fiber. Two types of silanes were used to modify the fibers: 3-chloropropylmethyldimethoxysilane and N-(2-aminoethyl)-3-aminopropyl trimethoxysilane at concentrations of 1 and 2 wt.%. The fibers feature were estimated with the use of Fourier Transform Infrared Spectroscopy (FTIR), scanning electron microscopy (SEM) and thermogravimetric analysis (TGA). Moreover, the thermal stability, impact strength, bending strength, impact resistance, density and water absorption of the composites were determined.
Experimental
Materials and fibre surface treatments
In this study the subsequent materials were used: 50% biosourced epoxy resin Gédéo Crystal Resin Bio PRO (Pebeo) with hardener, 3-chloropropylmethyldimethoxysilane (described as silane-Cl) and N-(2-aminoethyl)-3-aminopropyltrimethoxysilane (described as silane-NH2) both produced Sigma Aldrich (). The fabric used was made of Himalayan nettle, plain weave, grammage 370 g/m2, hand-woven (Pokhara, Nepal). Due to the average length of the Himalayan nettle fiber is 1.5 m, the use of industrial spinning methods is limited. The main chemical constituents of nettle fibers were determined in accordance with the methodology for determining the chemical composition of biomass: extractable substances using the Soxhlet method in ethanol; mineral substances according to DIN 51,731, lignin according to the Tappi method; cellulose by Seifert method; holocellulose using sodium chlorite. The results are given in % of dry weight: 83,7% cellulose, 4,04% lignin, 95,69% holocellulose, 1,26% extractive substances, 6,21% mineral substances.
Figure 1. Chemical structure of the silanes used a) 3-chloropropylmethyldimethoxysilane (silane-Cl) b) N-(2-aminoethyl)-3-aminopropyltrimethoxysilane (silane-NH2).

The silane-Cl and silane-NH2 1 and 2 wt.% respectively were dissolved in a mixture of water/ethanol (20:80 w/w). The pH of the solution was adjusted to the value equal to 4 with acetic acid and stirred continuously for 2 h. Next, the fibers were immersed in the solution for 2 h at room temperature. Then fibers were filtered and rinsed in demineralized water were dried in air for 24 h and 80°C for 12 h. During that time the silane agent infiltrated into nettle fibers and reacted with the OH group on the cell wall (Abdelmouleh et al. Citation2005).
The composites were made by hand lamination by placing 6 layers of fabric on a teflon mold. Then, the composite sheets were post-cured at 50°C/24 and 100°C/1 h. The test samples were cut using water cutting technology. Depending on the fiber modification method used, the composites were marked: 1%NH2 laminate; 2%NH2 laminate; 1%Cl laminate; 2%Cl laminate. The composite made of unmodified nettle fiber is marked as: laminate.
Method of characterization
Fourier Transform Infrared Spectroscopy (FTIR)
To examine the surface of the fibers, the Attenuated Total Reflectance Fourier Transform Infrared Spectroscopy (ATR-FTIR) was applied by spectrometer Bruker Vertex 70 (Germany) with a total of 64 scans at a resolution of 1 cm−1.
Scanning Electron Microscopy (SEM)
The fiber and composites morphology was investigated with the use of a scanning electron microscope TM 3000 Hitachi (Tokyo, Japan). All the specimens were sputtered with a layer of gold with palladium
Thermogravimetry (TGA)
The thermal stability was assessed by the thermogravimetric method (TGA) with temperatures ranging from 30 to 900°C at the heating rate of 10°C/min under nitrogen atmospheres using a TG 209 F1 Netzsch (Germany) apparatus. Approximately 10 mg samples were placed in ceramic pans. The T5% and T10% decomposition temperature was determined as 5% and 10% weight loss temperature respectively. The residual mass (ΔW%) was defined at about 900°C. The maximum temperature of thermal degradation and rate was also determined based on derivative thermogravimetric curves (DTG).
Density determination and evaluation of porosity
Density of the materials was determined using the Pycnomatic gas pycnometer by Thermo Fisher Scientific Inc. (Waltham, MA, USA) in helium atmosphere (0,2 MPa) at 20°C and by using measuring cell with a volume of 40 cm3.
Water absorption
Samples measuring approximately (length × width) 30 mm × 10 mm were cut from the plate. Each sample was accurately weighed and sized before testing for water absorption. The samples were then immersed in distilled water for 24 h, 48 h and 72 h and maintained at 20°C. After the appropriate time, the samples were removed, dried on blotting paper and then weighed on an analytical balance RADWAG AS 220.R2 (Poland) to the accuracy of 0.0001 g. This operation was repeated after the specified time. Three repetitions of each material were performed.
Charpy impact strength
The impact strength of unnotched composite samples was tested using the Charpy method (ISO 179) at room temperature. Furthermore, the peak load was defined as the maximum force (Fmax) measured during the experimental test. A Zwick/Roell HIT 25P (Germany) impact tester with a 5 J hammer was used.
Flexural test
Three-point bending test of samples (with specimen dimensions of 150 mm x 20 mm x 8.0 mm) cut from the sheets of composites were examined using the Zwick Roell Z010 (Germany) testing machine in accordance with DIN EN ISO 14,125 with a testing speed of 1 mm/min and with a load cell of 10 kN. The length of the span was calculated as 16 times the sample thickness.
Impact test
Impact tests were conducted by the TQC Impact tester 1890 Industrial Physics (Netherlands) according to ISO 6272–1:2002. In order to assess the type of damage of the composites to impact energies 10 J were used.
Results and discussion
Fourier transform infrared spectroscopy
The FTIR spectra obtained for unmodified and modified nettle fibers are presented in . Moreover, the effectiveness of the silanization process was assessed after various times of immersion of the fibers in the solution, i.e. after 30 min, 1 h and 2 h. Raw nettle fibers are characterized by a high cellulose content and lower hemicellulose and lignin content compared to other natural fibers (Mudoi, Sinha, and Parthasarthy Citation2023). When treating fibers with silanes, the methoxy groups of the coupling agent can hydrolyze in water and result in the formation of silanol, which reacts with the – OH group of the cellulose fibers and forms a stable covalent Si-O-Si bond with the cell wall (Kalia, Kaith, and Kaur Citation2009). The FTIR spectra of fibers exhibit characteristic absorption bands from groups of lignocellulosic materials (Masłowski et al. Citation2021). Broad band at ῡ = 3620–3000 cm−1 ascribed to the vibration of – OH phenol and alcohol groups was visible in all the spectra. At ῡ = 3100–2800 cm−1 peaks corresponding to asymmetric and symmetric methyl and methylene stretching vibrations of C-H bonds in cellulose and hemicellulose are observed (Viju and Thilagavathi Citation2022). At ῡ = 1730–1690 cm−1 characteristic vibrations of C=O from ester and aldehyde groups were noticed. The bands in the range of 1750–1500 cm−1 may be attributed to amines, alkenes and aryl groups. The vibration of C – O, C – C, C – O – C groups related to axial deformation, asymmetric and symmetric stretching esters and ethers from hemicellulose and lignin are confirmed by peaks at 1200–1060 cm−1 (De Rosa et al. Citation2010). The peak at 1020 cm−1 is ascribed to the asymmetric stretching of phosphates.
Figure 2. FTIR spectra of untreated and treated nettle fibers with a) 1% of N-(2-aminoethyl)-3-aminopropyltrimethoxysilane, b) 2% of N-(2-aminoethyl)-3-aminopropyl trimethoxysilane.

Figure 3. FTIR spectra of untreated and treated nettle fibers with a) 1% 3-chloropropylmethyldimethoxysilane b) 2% 3-chloropropylmethyldimethoxysilane.

Figure 4. SEM images of untreated and treated nettle fibers a) pure nettle fiber b) 1%NH2 nettle c) 2%NH2 nettle d) 1%Cl nettle e) 2%Cl nettle.

Figure 5. SEM images of investigated composites a) laminate b) 1%NH2 laminate c) 2%NH2 laminate d) 1%Cl laminate e) 2%Cl laminate.

After silane-NH2 treatment a new peak emerged at 3700 cm−1 due to the N-H stretching vibration of the amine groups bonded to the nettle fibers (Dharmalingam, Meenakshisundaram, and Kugarajah Citation2020). According to the literature (Majoul, Aouida, and Bessaïs Citation2015; Sepe et al. Citation2018), the characteristic bands from silane should be observable at ca. 1410 cm−1 matching the SiCH2 peak, at 1130 cm−1 and 1044 cm−1 at asymmetric stretching modes assigned to the Si-O-Si bond. Furthermore, the peaks from the NH2 deformation modes of the amine groups are visible at 1562 cm−1 and 1484 cm−1. A broad band at 698 cm−1 may indicate the presence of the C-Cl bond, while the broad absorption at 1167 cm−1 of the Si-alkyl groups (Adam, Osman, and Hello Citation2009). Furthermore, it can be assumed that for all tested fibers, that a prolong residence time of the fabric in the silylation solution leads to the formation of an increased number of connections between the coupling agent and the cell wall. This is corroborated by more the heightened peaks in the wavelength range attributed to the presence of silanol groups, specifically various Si-O – H groups (at 3612, 3525 and 3473 cm−1) such as ≡Si(OH), =Si(OH)2, –Si(OH)3 and (SiO2)=Si(OH)CH2CH2CH2Cl along with the Si – O – Si vibrations near ῡ = 1101 cm−1 (Adam, Osman, and Hello Citation2009).
Amine group is more reactive than halogenated. It can be assumed that in the case of the NH2 group, the number of -OH bonds formed will be greater than in the case of the Cl group (Nandi and Das Citation2023).
Scanning Electron Microscopy (SEM)
The structures of the treated fiber were evaluated by scanning electron microscopy. The SEM micrographs of nettle silane treatment fibers with and without are shown in . Silanes are widely used as efficient coupling agents to improve adhesion between fiber reinforcement and polymer matrix (Xie et al. Citation2010). During the silanization treatment the natural fiber surface is coated with silanes and this thin film may be visible in SEM pictures and marked. As a result of silane treatment, a layer of deposited silane was observed on the fiber surface (Melo et al. Citation2022). The treatment did not negatively affect the fiber structure, which proves that the silanization process was carried out correctly. Chemical treatment of natural materials aims to remove non-cellulosic components and impurities from the fiber surface. Moreover, rough surfaces of modified fibers are often observed, which improves the interfacial bond between the polymer matrix and the natural reinforcement (Madhu, Sanjay, Senthamaraikannan, et al. Citation2020; Shravanabelagola Nagaraja Setty et al. Citation2022). In order to assess the connections at the fiber/polymer matrix interface, shows SEM images of the fractures of composite samples after the impact test.
The images of the fractures composites show that the silane treatment process with amine groups ensured effective adhesion between the fiber and the epoxy matrix. It can be observed that for composites 1%NH2 laminate and 2%NH2 laminate cracking of the fiber is visible, not of the matrix. The presence of -OH groups on the surface of silane-treated fibers improves the adhesion between the epoxy matrix and the natural fiber (Kaliappan et al. Citation2023). Moreover, the amino groups present in NH2-silane may react with the epoxy monomer. A characteristic pull-out effect is visible for the composite based on unmodified nettle fibers. In it can be observed the holes that were created after pulling the fibers out of the matrix. The functional groups contained in the silane structure promote covalent bonds at the fiber matrix interface, which translates into favorable mechanical properties of layered materials.
Thermogravimetry
Thermogravimetric analysis of fibers and composites in a nitrogen atmosphere was carried out, and the characteristic curves are shown in In all cases, a single-stage decomposition process of the tested materials was observed.
Detailed information on the fiber and composites degradation characteristics, such as: 5% and 10% weight loss temperatures (T5% and T10%), maximum intensity of thermal degradation temperatures (DTG), and residual mass, are summarized in . These data are necessary to select parameters during the processing and production of bio composites with natural fibers. The characteristics weight loss in the range 60–100°C is attributed to the heat of the vaporizing water in the natural materials. In chance, weight loss in range 325–350°C is the break of glucosides linkages of cellulose (Manikandan Nair, Thomas, and Groeninckx Citation2001). The silane treatment may cause the formation of new groups on the fiber surface, which can restrict the segmental mobility and improve the thermal stability of the fiber (Gañan et al. Citation2005). For silane-treated fibers, a larger amount of residue was observed, which indicates the presence of an inorganic phase. In the case of 1%NH2-silane and 1%Cl-silane fibers, a slight increase in thermal stability was observed compared to unmodified nettle fiber. In the group of tested composites, no significant changes were observed, the average temperature T5% of the beginning of degradation was 320°C, and the residue was approximately 12%.
Table 1. TG and DTG data of modified nettle fiber.
Table 2. TG and DTG data of investigate composites.
Density determination and evaluation of porosity
The results of the obtained actual density of the materials were used to calculate the theoretical density and porosity of composites according to EquationEquations (1)(1)
(1) and (Equation2
(2)
(2) ) as the difference between the theoretical and actual density including the content of nettle fiber fabric.
where: ρth – theoretical density of the composite, [g/cm3]; ρm – density of the matrix, g/cm3;
ρf – density of the nettle fabric, g/cm3; φ- a volume fraction of the fabric.
The porosity of the material was determined by EquationEquation (2)(2)
(2) :
where: p-porosity [%]; ρac – an experimental density of composite [g/cm3].
summarizes the obtained density and porosity results of the tested materials. The density of all composites was similar and amounted to 1.23 g/cm3 on average. This confirms that the production method did not have a significant impact on the structure of the composites. However, a slight increase in porosity was observed for composites made of modified fibers, which may be due to the entanglement of the bundles during the fiber treatment process.
Table 3. Density and porosity of the tested composites.
Water absorption
Moisture absorption of natural fiber polymer composites is one of the main problems that may eliminate their use as outdoor structural elements. Plastic is usually hydrophobic. Moisture can penetrate into the composite by natural fibers (Wang, Sain, and Cooper Citation2006). The water absorption results after 24, 48 and 72 h for the tested materials are summarized in . It can be seen that the treatment of silane-Cl fibers had a positive effect on reducing water absorption by the composite. Water absorption after 72 hours for the 1%Cl laminate and 2%Cl laminate was 3.75% and 4.51%, respectively, compared to the reference laminate for which it was 5.12%.
Table 4. Water absorption of the tested materials.
Charpy impact strength
Impact strength corresponds to the brittleness of materials, described by the work required to dynamically fracture the sample and depending on the size of the cross-section samples (Jia et al. Citation2022). Layered composites are exposed to lateral impact loads during operation. Therefore, the use of an effective method of modifying reinforcing fibers, i.e. silanization, can improve the impact resistance of construction materials (Lascano et al. Citation2021). The determined values of impact strength and the maximum force needed to destroy the sample are listed in . The appearance of the samples after the test is shown in . It should be emphasized that the silane treatment had a positive effect on the impact strength of the tested layered composites. The highest value of impact strength as well as the force needed to destroy the sample was recorded for the 1%NH2 laminate and 1%Cl laminate. For all composites reinforced with silanized nettle fiber, the F max values were higher than the reference sample. This is indirectly confirmed by the higher adhesion between the natural fiber and the epoxy matrix (Gassan Citation2002). The results of the work are consistent with the reports of Suryawan et al., who showed that the treatment of nettle fibers with 6% silane coupling agent improved the impact strength of epoxy-reinforced composites (Suryawan et al. Citation2023). Parhipan et al. also demonstrated a beneficial effect of silane treatment of natural fibers on the impact strength of kenaf fiber-reinforced epoxy composite (Parthipan et al. Citation2020).
Figure 8. Photographs of the damaged area of composites after the impact test. 1- laminate, 2- 1%NH2 laminate, 3- 2%NH2 laminate, 4- 1%Cl laminate, 5- 2%Cl laminate.

Table 5. Impact strength and bending strength of the tested composites.
Flexural strength
The flexural behavior of composites depends on the ductility of the matrix and fiber modulus (Parmiggiani, Prato, and Pizzorni Citation2021). The flexural strength () of the tested composites with modified nettle fiber was on average 65 MPa and was lower than the reference sample, whose flexural strength was 71 MPa. The interfacial adhesion has a significant impact on the mechanical properties of composites consisting of hydrophilic fiber and hydrophobic polymer (Chandra Sekhar et al. Citation2023). Silane treatment can protect the fiber surface from moisture absorption by forming chemical bonds with the fiber structure. However, the process of treating the fibers in a solution with silane could indirectly affect their structure by softening the fibers, which in the bending test showed greater plasticity than unmodified nettle fibers. The lower of fiber strength maybe due to the severe dissolution of hemicellulose as interfibrillar matrix after modifications (Zhu et al. Citation2015). Furthermore, the presence of long silane chains in composites can act as a plasticizing agent. However, the 2%NH2 laminate and 2%Cl laminate samples showed higher values of the flexural modulus than the reference sample, which proves that the silanes at a concentration of 2% by weight used for fiber modification effectively improved the flexural properties of composites. Chao et al also observed a beneficial effect of silane treatment of natural fibers on the flexural modulus of thermosetting composites (Cho, Lee, and Han Citation2009).
The images of the samples during the study are presented in . The photos show that bio composites reinforced with modified nettle fiber deform more plastically compared to the reference sample.
Impact test
The impact test provides information about the response of the composite structure to impact (Matykiewicz and Barczewski Citation2020). It allows rank materials according to the force required to break flat, rigid plastic samples. displays impact side and back side of the laminates after impact test. The use of silane treatment of fibers resulted in different types of impact and shape of damaged areas in investigated composites. The main damage area was visible around the impact site for all samples tested. However, for composites reinforced with modified fiber, the damage area and crack type on the back side are more regular than for the reference samples. This proves that these composite plates absorb impact energy more regularly.
Conclusions
The research results confirmed that the use of Himalayan nettle fiber as a reinforcement in epoxy composites allows the production of light materials with favorable thermal and mechanical properties. As a result of the silane treatment method described in this study, the use of nettle fabric in the production of epoxy biocomposites will be more efficient. The FTIR method confirmed the silanization of nettle fibers using: 3-chloropropylmethyldimethoxysilane and N-(2-aminoethyl)-3-aminopropyl trimethoxysilane. Modified with 1% silane-NH2 and 1%silane-Cl fibers showed higher thermal stability compared to unmodified nettle fibers. SEM images of the fractures composites show that the silane-NH2 treatment ensured more effective adhesion between the fiber and the epoxy matrix than silane-Cl treatment. This is due to the greater reactivity of NH2 groups with the epoxy matrix than Cl groups. It should be highlighted that the silane treatment had a positive effect on the impact strength, impact resistance and flexural modulus of the tested layered composites. The highest value of impact strength as well as the force needed to destroy the sample was recorded for the 1%NH2 laminate and 1%Cl laminate.
Highlights
Himalayan nettle fiber as a reinforcement in epoxy composites allows the production of light materials with favorable thermal and mechanical properties.
Silane treatment had a positive effect on the impact strength of the nettle fibre/epoxy layered composites.
NH2-silane treatment ensured more effective adhesion between the fiber and the epoxy matrix than Cl-silane treatment.
Disclosure statement
No potential conflict of interest was reported by the author(s).
Additional information
Funding
References
- Abdelmouleh, M., S. Boufi, M. N. Belgacem, A. Dufresne, and A. Gandini. 2005. “Modification of Cellulose Fibers with Functionalized Silanes: Effect of the Fiber Treatment on the Mechanical Performances of Cellulose–Thermoset Composites.” Journal of Applied Polymer Science 98 (3): 974–19. https://doi.org/10.1002/app.22133.
- Adam, F., H. Osman, and K. M. Hello. 2009. “The Immobilization of 3-(Chloropropyl)triethoxysilane Onto Silica by a Simple One-Pot Synthesis.” Journal of Colloid and Interface Science 331 (1): 143–147. https://doi.org/10.1016/j.jcis.2008.11.048.
- Andrzejewski, J., M. Barczewski, D. Czarnecka-Komorowska, T. Rydzkowski, K. Gawdzińska, and V. K. Thakur. 2024. “Manufacturing and Characterization of Sustainable and Recyclable Wood-Polypropylene Biocomposites: Multiprocessing-Properties-Structure Relationships.” Industrial Crops and Products 207: 117710. https://doi.org/10.1016/j.indcrop.2023.117710.
- Aniśko, J., and M. Barczewski. 2023. “Uniaxial Rotational Molding of Bio-Based Low-Density Polyethylene Filled with Black Tea Waste.” Materials 16 (10): 3641. https://doi.org/10.3390/ma16103641.
- Bambach, M. R. 2020. “Direct Comparison of the Structural Compression Characteristics of Natural and Synthetic Fiber-Epoxy Composites: Flax, Jute, Hemp, Glass and Carbon Fibers.” Fibers 8 (10): 62. https://doi.org/10.3390/fib8100062.
- Barczewski, M., K. Sałasińska, W. Raś, A. Hejna, S. Michałowski, P. Kosmela, J. Aniśko, A. Boczkowska, and M. Szostak. 2023. “The Effect of Hybridization of Fire Retarded Epoxy/flax-Cotton Fiber Laminates by Expanded Vermiculite: Structure-Property Relationship Study.” Advanced Industrial and Engineering Polymer Research 6 (2): 181–194. https://doi.org/10.1016/j.aiepr.2023.01.005.
- Bhattacharyya, D., A. Subasinghe, and N. K. Kim. 2015. “Natural Fibers.” In Multifunctionality of Polymer Composites, 102–143. Elsevier.
- Chandra Sekhar, V., A. Dasore, B. Yalamasetti, K. Sudha Madhuri, G. Narendar 2023. “Flexural Behavior of Natural Fiber Epoxy Composites.” Materials Today: Proceedings.
- Chew, E., J. L. Liu, T. E. Tay, L. Q. N. Tran, and V. B. C. Tan. 2021. “Improving the Mechanical Properties of Natural Fibre Reinforced Laminates Composites Through Biomimicry.” Composite Structures 258: 113208. https://doi.org/10.1016/j.compstruct.2020.113208.
- Cho, D., H. S. Lee, and S. O. Han. 2009. “Effect of Fiber Surface Modification on the Interfacial and Mechanical Properties of Kenaf Fiber-Reinforced Thermoplastic and Thermosetting Polymer Composites.” Composite Interfaces 16 (7–9): 711–729. https://doi.org/10.1163/092764409X12477427307537.
- De Rosa, I. M., J. M. Kenny, D. Puglia, C. Santulli, and F. Sarasini. 2010. “Morphological, Thermal and Mechanical Characterization of Okra (Abelmoschus Esculentus) Fibres As Potential Reinforcement in Polymer Composites.” Composites Science and Technology 70 (1): 116–122. https://doi.org/10.1016/j.compscitech.2009.09.013.
- Dharmalingam, S., O. Meenakshisundaram, and V. Kugarajah. 2020. “Effect of Degree of Silanization of Luffa on the Properties of Luffa-Epoxy Composites.” Colloids and Surfaces A: Physicochemical and Engineering Aspects 603: 125273. https://doi.org/10.1016/j.colsurfa.2020.125273.
- Doan, T.-T.-L., H. Brodowsky, and E. Mäder. 2012. “Jute Fibre/Epoxy Composites: Surface Properties and Interfacial Adhesion.” Composites Science and Technology 72 (10): 1160–1166. https://doi.org/10.1016/j.compscitech.2012.03.025.
- Elfaleh, I., F. Abbassi, M. Habibi, F. Ahmad, M. Guedri, M. Nasri, and C. Garnier. 2023. “A Comprehensive Review of Natural Fibers and Their Composites: An Eco-Friendly Alternative to Conventional Materials.” Results in Engineering 19:101271. https://doi.org/10.1016/j.rineng.2023.101271.
- Fischer, H., E. Werwein, and N. Graupner. 2012. “Nettle fibre (Urtica dioica L.) reinforced poly(lactic acid): A first approach.” Journal of Composite Materials 46 (24): 3077–3087. https://doi.org/10.1177/0021998311435676.
- Gañan, P., S. Garbizu, R. Llano‐Ponte, and I. Mondragon. 2005. “Surface Modification of Sisal Fibers: Effects on the Mechanical and Thermal Properties of Their Epoxy Composites.” Polymer Composites 26 (2): 121–127. https://doi.org/10.1002/pc.20083.
- Gassan, J. 2002. “A Study of Fibre and Interface Parameters Affecting the Fatigue Behaviour of Natural Fibre Composites. ” Composites Part A: Applied Science and Manufacturing. 33 (3): 369–374. https://doi.org/10.1016/S1359-835X(01)00116-6.
- Gieparda, W., S. Rojewski, and W. Różańska. 2021. “Effectiveness of Silanization and Plasma Treatment in the Improvement of Selected Flax Fibers’ Properties.” Materials 14 (13): 3564. https://doi.org/10.3390/ma14133564.
- Jeyapragash, R., V. Srinivasan, and S. Sathiyamurthy 2020. “Mechanical Properties of Natural Fiber/Particulate Reinforced Epoxy Composites – a Review of the Literature.” Materials Today: Proceedings, 22, 1223–1227.
- Jia, W., A. Pi, Z. Zhao, S. Wang, C. Wei, Z. Jie, and F. Huang. 2022. “Study on Intrinsic Influence Law of Specimen Size and Loading Speed on Charpy Impact Test.” Materials 15 (11): 3855. https://doi.org/10.3390/ma15113855.
- Kalia, S., B. S. Kaith, and I. Kaur. 2009. “Pretreatments of Natural Fibers and Their Application As Reinforcing Material in Polymer Composites – A Review.” Polymer Engineering & Science 49 (7): 1253–1272. https://doi.org/10.1002/pen.21328.
- Kaliappan, S., B. Arunadevi, N. Sateesh, and B. C. Nookaraju. 2023. “Effect of Amino Silane Grafted Cellulose and Kenaf Fibers in Mechanical, Impact Toughness and Drilling Characteristics of Epoxy Resin Composite.” Silicon 15 (7): 3149–3158. https://doi.org/10.1007/s12633-022-02245-x.
- Kamarudin, S. H., M. S. Mohd Basri, M. Rayung, F. Abu, S. Ahmad, M. N. Norizan, S. Osman, N. Sarifuddin, M. S. Z. M. Desa, U. H. Abdullah, et al. 2022. “A Review on Natural Fiber Reinforced Polymer Composites (NFRPC) for Sustainable Industrial Applications.” Polymers 14 (17): 3698. https://doi.org/10.3390/polym14173698.
- Karimah, A., M. R. Ridho, S. S. Munawar, D. S. Adi, Ismadi, R. Damayanti, B. Subiyanto, W. Fatriasari, and A. Fudholi. 2021. “A Review on Natural Fibers for Development of Eco-Friendly Bio-Composite: Characteristics, and Utilizations.” Journal of Materials Research and Technology 13: 2442–2458. https://doi.org/10.1016/j.jmrt.2021.06.014.
- Kenned, J. J., K. Sankaranarayanasamy, and C. S. Kumar. 2021. “Chemical, Biological, and Nanoclay Treatments for Natural Plant Fiber-Reinforced Polymer Composites: A Review.” Polymers and Polymer Composites 29 (7): 1011–1038. https://doi.org/10.1177/0967391120942419.
- Khan, F. M., A. H. Shah, S. Wang, S. Mehmood, J. Wang, W. Liu, and X. Xu. 2022. “A Comprehensive Review on Epoxy Biocomposites Based on Natural Fibers and Bio-Fillers: Challenges, Recent Developments and Applications.” Advanced Fiber Materials 4 (4): 683–704. https://doi.org/10.1007/s42765-022-00143-w.
- Kumar, N., and D. Das. 2017. “Fibrous Biocomposites from Nettle (Girardinia Diversifolia) and Poly(lactic Acid) Fibers for Automotive Dashboard Panel Application.” Composites Part B: Engineering 130: 54–63. https://doi.org/10.1016/j.compositesb.2017.07.059.
- Lascano, D., R. Balart, D. Garcia-Sanoguera, A. Agüero, T. Boronat, and N. Montanes. 2021. “Manufacturing and Characterization of Hybrid Composites with Basalt and Flax Fabrics and a Partially Bio-Based Epoxy Resin.” Fibers and Polymers 22 (3): 751–763. https://doi.org/10.1007/s12221-021-0209-5.
- Libera Junior, V. D., L. A. Teixeira, S. C. Amico, and S. Maria da Luz. 2022. “Processing, Thermal and Mechanical Properties of Composite Laminates with Natural Fibers Prepregs.” Polymers and Polymer Composites 30:096739112210875. https://doi.org/10.1177/09673911221087591.
- Madhu, P., M. R. Sanjay, M. Jawaid, S. Siengchin, A. Khan, and C. I. Pruncu. 2020. “A New Study on Effect of Various Chemical Treatments on Agave Americana Fiber for Composite Reinforcement: Physico-Chemical, Thermal, Mechanical and Morphological Properties.” Polymer Testing 85: 106437. https://doi.org/10.1016/j.polymertesting.2020.106437.
- Madhu, P., M. R. Sanjay, P. Senthamaraikannan, S. Pradeep, S. Siengchin, M. Jawaid, and M. Kathiresan. 2020. “Effect of Various Chemical Treatments of Prosopis juliflora Fibers As Composite Reinforcement: Physicochemical, Thermal, Mechanical, and Morphological Properties.” Journal of Natural Fibers 17 (6): 833–844. https://doi.org/10.1080/15440478.2018.1534191.
- Mahendrakumar, N., P. R. Thyla, P. V. Mohanram, A. Sabareeswaran, R. B. Manas, and S. Srivatsan. 2015. “Mechanical and Dynamic Properties of Nettle-Polyester Composite.” Materials Express 5 (6): 505–517. https://doi.org/10.1166/mex.2015.1263.
- Majoul, N., S. Aouida, and B. Bessaïs. 2015. “Progress of Porous Silicon APTES-Functionalization by FTIR Investigations.” Applied Surface Science 331: 388–391. https://doi.org/10.1016/j.apsusc.2015.01.107.
- Manikandan Nair, K., S. Thomas, and G. Groeninckx. 2001. “Thermal and Dynamic Mechanical Analysis of Polystyrene Composites Reinforced with Short Sisal Fibres.” Composites Science and Technology 61 (16): 2519–2529. https://doi.org/10.1016/S0266-3538(01)00170-1.
- Masłowski, M., A. Aleksieiev, J. Miedzianowska, and K. Strzelec. 2021. “Common Nettle (Urtica dioica L.) as an Active Filler of Natural Rubber Biocomposites.” Materials 14 (7): 1616. https://doi.org/10.3390/ma14071616.
- Matykiewicz, D., and M. Barczewski. 2020. “On the Impact of Flax Fibers As an Internal Layer on the Properties of Basalt-Epoxy Composites Modified with Silanized Basalt Powder.” Composites Communications 20: 100360. https://doi.org/10.1016/j.coco.2020.100360.
- Melo, E. C. R. D., M. D. O. Camillo, P. R. C. Marcelino, R. Barbosa dos Santos da Silva, T. Colares Firmino, B. Ferreira de Oliveira, D. Profeti, A. Camposo Pereira, S. Neves Monteiro, and M. Picanço Oliveira. 2022. “Influence of Silanization Treatment of Sponge Gourd (Luffa Cylindrica) Fibers on the Reinforcement of Polyester Composites: A Brief Report.” Polymers 14 (16): 3311. https://doi.org/10.3390/polym14163311.
- Mudoi, M. P., S. Sinha, and V. Parthasarthy. 2023. “Himalayan Nettle Fibre-Reinforced Polymer Composite: A Physical, Mechanical, and Thermal Analysis.” Biomass Conversion and Biorefinery. https://doi.org/10.1007/s13399-023-04819-0.
- Nandi, P., and D. Das. 2023. “Mechanical, Thermo-Mechanical and Biodegradation Behaviour of Surface-Silanized Nettle Fabric-Reinforced Poly(lactic Acid) Composites.” Materials Chemistry and Physics 297: 127381. https://doi.org/10.1016/j.matchemphys.2023.127381.
- Okafor, C. E., L. C. Kebodi, J. Kandasamy, M. May, and I. E. Ekengwu. 2022. “Properties and Performance Index of Natural Fiber Reinforced Cross-Ply Composites Made from Dioscorea Alata Stem Fibers.” Composites Part C Open Access 7: 100213. https://doi.org/10.1016/j.jcomc.2021.100213.
- Parmiggiani, A., M. Prato, and M. Pizzorni. 2021. “Effect of the Fiber Orientation on the Tensile and Flexural Behavior of Continuous Carbon Fiber Composites Made via Fused Filament Fabrication.” The International Journal of Advanced Manufacturing Technology 114 (7–8): 2085–2101. https://doi.org/10.1007/s00170-021-06997-5.
- Parthipan, N., M. Ilangkumaran, T. Maridurai, and S. C. Prasanna. 2020. “Effect of Silane Treated Silicon (IV) Oxide Nanoparticle Addition on Mechanical, Impact Damage and Drilling Characteristics of Kenaf Fibre-Reinforced Epoxy Composite.” Silicon 12 (2): 459–467. https://doi.org/10.1007/s12633-019-00138-0.
- Paukszta, D., J. Mańkowski, J. Kołodziej, and M. P. Szostak. 2013. “Polypropylene (PP) Composites Reinforced with Stinging Nettle (Urtica Dioica L.) Fiber.” Journal of Natural Fibers 10 (2): 147–158. https://doi.org/10.1080/15440478.2013.789287.
- Rangappa, S. M., S. Siengchin, J. Parameswaranpillai, M. Jawaid, and T. Ozbakkaloglu. 2022. “Lignocellulosic Fiber Reinforced Composites: Progress, Performance, Properties, Applications, and Future Perspectives.” Polymer Composites 43 (2): 645–691. https://doi.org/10.1002/pc.26413.
- Sanjay, M. R., P. Madhu, M. Jawaid, P. Senthamaraikannan, S. Senthil, and S. Pradeep. 2018. “Characterization and Properties of Natural Fiber Polymer Composites: A Comprehensive Review.” Journal of Cleaner Production 172: 566–581. https://doi.org/10.1016/j.jclepro.2017.10.101.
- Sanjay, M. R., S. Siengchin, J. Parameswaranpillai, M. Jawaid, C. I. Pruncu, and A. Khan. 2019. “A Comprehensive Review of Techniques for Natural Fibers As Reinforcement in Composites: Preparation, Processing and Characterization.” Carbohydrate Polymers 207: 108–121. https://doi.org/10.1016/j.carbpol.2018.11.083.
- Sarikaya, E., H. Çallioğlu, and H. Demirel. 2019. “Production of Epoxy Composites Reinforced by Different Natural Fibers and Their Mechanical Properties.” Composites Part B: Engineering 167: 461–466. https://doi.org/10.1016/j.compositesb.2019.03.020.
- Sepe, R., F. Bollino, L. Boccarusso, and F. Caputo. 2018. “Influence of Chemical Treatments on Mechanical Properties of Hemp Fiber Reinforced Composites.” Composites Part B: Engineering 133: 210–217. https://doi.org/10.1016/j.compositesb.2017.09.030.
- Shravanabelagola Nagaraja Setty, V. K., G. Goud, S. Peramanahalli Chikkegowda, S. Mavinkere Rangappa, and S. Siengchin. 2022. “Characterization of Chemically Treated Limonia Acidissima (Wood Apple) Shell Powder: Physicochemical, Thermal, and Morphological Properties.” Journal of Natural Fibers 19 (11): 4093–4104. https://doi.org/10.1080/15440478.2020.1853925.
- Suarsana, I., I. Suryawan, N. Suardana, S. Winaya, R. Soenoko, B. Suyasa, W. Sunu, and M. Rasta. 2021. “Flexural Strength of Hybrid Composite Resin Epoxy Reinforced Stinging Nettle Fiber with Silane Chemical Treatment.” AIMS Materials Science 8 (2): 185–199. https://doi.org/10.3934/matersci.2021013.
- Suryawan, I. G. P. A., N. P. G. Suardana, I. Suarsana, W. Widhiada, and L. S. Putra. 2023. “Analysis of Stinging Nettle (Laportea Bulbifera (Siebold & Zucc) Wedd) Fiber in NaOH and Silane on the Impact Strength of Composite Epoxy for Electric Battery Box.” 040020.
- Torres-Arellano, M., V. Renteria-Rodríguez, and E. Franco-Urquiza. 2020. “Mechanical Properties of Natural-Fiber-Reinforced Biobased Epoxy Resins Manufactured by Resin Infusion Process.” Polymers 12 (12): 2841. https://doi.org/10.3390/polym12122841.
- Viju, S., and G. Thilagavathi. 2022. “Characterization of Surface Modified Nettle Fibers for Composite Reinforcement.” Journal of Natural Fibers 19 (5): 1819–1827. https://doi.org/10.1080/15440478.2020.1788491.
- Wang, W., M. Sain, and P. Cooper. 2006. “Study of Moisture Absorption in Natural Fiber Plastic Composites.” Composites Science and Technology 66 (3–4): 379–386. https://doi.org/10.1016/j.compscitech.2005.07.027.
- Xie, Y., C. A. S. Hill, Z. Xiao, H. Militz, and C. Mai. 2010. “Silane Coupling Agents Used for Natural Fiber/Polymer Composites: A Review.” Composites Part A, Applied Science and Manufacturing 41 (7): 806–819. https://doi.org/10.1016/j.compositesa.2010.03.005.
- Zaaba, N. F., and H. Ismail. 2019. “Thermoplastic/Natural Filler Composites: A Short Review.” Journal of Physical Science 30 (Supp.1): 81–99. https://doi.org/10.21315/jps2019.30.s1.5.
- Zhu, J., H. Zhu, K. Immonen, J. Brighton, and H. Abhyankar. 2015. “Improving Mechanical Properties of Novel Flax/Tannin Composites Through Different Chemical Treatments.” Industrial Crops and Products 67: 346–354. https://doi.org/10.1016/j.indcrop.2015.01.052.