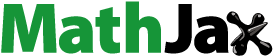
ABSTRACT
Mesenchymal stem cells (MSCs) with multipotential differentiation ability are main cell source for cartilage tissue engineering, but its application is restricted by the difficulty in regulating committed chondrogenic differentiation. Study revealed that certain substrate topographic structure can enhance the chondrogenic differentiation of MSCs. This study prepared silk fibroin (SF) films with gradient microscopic surface patterns based on P280-P7000 type specification sandpapers, and investigated the adhesion, proliferation and chondrogenic differentiation of human adipose-derived stem cells (hADSCs) on the SF films. The results showed that the SF films surface had convex dots with diameter of 3–50 μm, and hADSCs could adhere and proliferate well on the film. Transcriptomics analysis indicated that hADSCs tend to differentiate differently on the SF films, among which hADSCs on P1000 SF films had higher expression of chondrogenic markers Sox-9 and Col-II, with activated yes associated protein (YAP) and restricted F-actin. In vitro co-culture of human OA knee cartilage with hADSCs seeded SF films found satisfactory cartilage protection effect in P1000 group. Overall, this study preliminarily revealed the microscopic surface pattern of SF films may induce chondrogenic differentiation of hADSCs through extracellular matrix mediated Hippo/Yap pathway, and provided a new strategy for cartilage repair.
摘要
具有多向分化潜能的间充质干细胞是软骨组织工程的主要细胞来源,但其应用受难以调控定向成软骨细胞分化限制。研究表明,一定的基底表面拓扑结构可以促进间充质干细胞向软骨细胞分化。本研究基于P280-P7000规格的砂纸制备了具有梯度表面微形貌的丝素蛋白(SF)膜,并研究了人脂肪干细胞(hADSCs)在SF膜上的粘附、增殖和成软骨细胞分化行为。 结果表明,SF膜表面具有直径为3-50 μm的凸点,hADSCs能很好地在SF膜上粘附和增殖。转录组测序及分析表明,hADSCs在各组SF膜上具有不同的分化倾向,其中hADSCs在P1000组SF膜上具有更高的软骨形成标志蛋白Sox-9和Col II的表达,且yes相关蛋白(YAP)表达升高,F-肌动蛋白(F-actin)表达下降。人OA膝关节软骨外植体与接种了hADSCs的SF膜体外共培养证实,P1000组SF膜软骨保护效果最好。本研究初步揭示了SF膜的表面微形貌可能通过细胞外基质介导的Hippo/YAP途径诱导hADSCs成软骨细胞分化,可用于组织工程修复软骨损伤。
Introduction
Articular cartilage defect is a common form joint injury in clinical and is the leading cause of osteoarthritis (OA) and disability. Cartilage tissue engineering (CTE) with optimized combination of seed cells, bioscaffolds, and growth factors has been considered as one of the most promising strategies in regenerating articular cartilage defect (Armiento et al. Citation2018; B. J. Huang, Hu, and Athanasiou Citation2016). Significant research efforts are aimed at producing engineered cartilage as a cell-based approach for articular cartilage repair. Mesenchymal stem cells (MSCs) abundant in joint tissues (i.e. subchondral bone, synovial fluid, synovium, and adipose tissue) have been widely investigated as a valuable cell source in cartilage regeneration due to its multipotential differentiation ability, satisfactory proliferation and low immunogenicity property. And researches focused on the cell source had emphasized on the regulation of chondrogenic differentiation within the tissue engineered bioscaffolds (Xu et al. Citation2022).
In order to achieve favorable differentiation, the main manner of CTE in directing the chondrogenic differentiation of MSCs is adding growth factors, such as transforming growth factor-β3 (TGF-β3) and fibroblasts growth factor (FGF) (Cleary et al. Citation2015). However, growth factors are of high cost, and the dosage of growth factors needed to induce sufficient chondrogenic differentiation may beyond the normal level of relative growth factors in suit. For instance, the usage of TGF-β3 in CTE can cause the fibrosis of hyaline cartilage and even progress to osteoarthritis (OA) (van der Kraan PM and van der Kraan Citation2017). Besides, to achieve long-term controlled release of growth factors in CTE bioscaffolds remains a challenge (Kim and Mikos Citation2021). By far, growth factor free CTE bioscaffolds which made of chondrogenic inducing biomaterials (i.e. sulfated polysaccharide, gelatin, polylactic acid, alginate, hyaluronic acid, et al.) have become new alternative (Dinoro et al. Citation2019; Ghandforoushan et al. Citation2022; Liu et al. Citation2022; Rastogi and Kandasubramanian Citation2019; Trucco et al. Citation2021), whose chondrogenic inducing property can be further enhanced by functional-group-modification (-CH3, -OH) (Cao et al. Citation2017), and constructing patterned surface topology (Carthew et al. Citation2021). Nevertheless, this kind of CTE bioscaffolds is restrict by biocompatibility, suitable degradation, sufficient mechanical property and physicochemical plasticity.
Silk fibroin (SF) extracted from Bombyx mori silkworm cocoon is Food and Drug Administration (FDA) proved natural protein, which displays controllable in vivo bio-degradation, excellent mechanical properties, feasible modification and formability (Sahoo et al. Citation2023), and is widely used in CTE in variety forms of sponges, hydrogels, particles and coatings (Z. Zhou et al. Citation2022). Studies based on micro-surface morphology patterned polydimethylsiloxane (PDMS) molds revealed that the chondrogenic differentiation of MSCs can be enhanced by certain substrate topographic structure (He et al. Citation2022; Yao, Wang, and Ding Citation2021). In the present study, we prepared SF films with gradient microscopic surface patterns based on P280-P7000 type specification sandpapers and investigated the effects of topologic design on the adhesion, proliferation and chondrogenic differentiation of human adipose-derived stem cells (hADSCs). The SF films had convex dots on surface with diameter of 3–50 μm, which is close to the cell size. hADSCs were found to adhere and proliferate well on the surface of SF films, but represented distinct cellular morphology. Transcriptomics analysis indicated that hADSCs had different differentiation tendency on the SF films, among which hADSCs on P1000 SF films showed higher expression of chondrogenic markers Sox-9 and Col-II, with activated yes associated protein (YAP) and restricted F-actin. In vitro co-culture of human OA knee cartilage with hADSCs seeded SF films showed that P1000 group had better cartilage protection effect (). In conclusion, the results of this study preliminarily revealed the mechanism of microscopic surface pattern induced hADSCs chondrogenic differentiation, and proved that surface patterned SF films were promising CTE scaffold in supporting MSCs amplification and chondrogenic differentiation.
Figure 1. Study design and experimental scheme for the silk fibroin (SF) films.

Materials and methods
Preparation of SF films
Bombyx mori silkworm cocoons were kindly provided by the Institute of Biotechnology of Southwest University. Silk solutions were prepared according to our previously published procedures (Cheng et al. Citation2022). Briefly, silkworm cocoon was cut into pieces and boiled in 0.02 M Na2CO3 solution for 30 min, followed by a rinsing process with copious amounts of deionized water to extract sericin proteins. The degummed silk was dried in a 60°C oven and dissolved in ternary reagent (a mixture of CaCl2, EtOH, and H2O, with a molar ratio of 1:2:8) at 75°C for 1 h. The resulting solution was dialyzed in deionized water using a dialysis tube (molecular weight cutoff 3500) for 3 d, during which time the deionized water was changed every 3 h. Finally, the SF solution was centrifuged for 20 min at 4000 rpm and 4°C to remove impurities, and enriched to a concentration of 5 wt %.
Matador sandpaper (Germany) with type specification of P280, P1000, P2000, P5000, and P7000 (with graded gravel size) were used to fabricate the PDMS molds. In a typical workflow, sandpaper was cut into 3 cm × 3 cm and attached to a 60-mm petri dish with its rough surface upward. Sylgard-184A and Sylgard-184B (Dow Corning, USA) were mixed evenly in a mass ratio of 10:1, and then poured into the above culture dish slowly. The excess gas was removed by a vacuum pump, and the PDMS molds were obtained by reacting in an incubator at 60°C for 6 h. To prepare SF films with different microscopic surface texture, 2 mL of the SF solution (5 wt %) was added onto the PDMS mold and incubated in an incubator at 60°C for 90 min. Finally, the SF films were cross-linked by anhydrous ethanol (Tang et al. Citation2021).
Characterization of SF films
The microscopic surface morphology of the SF films was observed under a ZEISS Crossbeam 340 scanning electron microscope (SEM) after dehydration with gradient ethanol and acetone, and a Filmetrics-Optical profilometer (Profilm3D, USA) was utilized to reveal the 3D texture. For surface hydrophilicity, a contact angle goniometer (Ossila, UK) was used to acquire static contact angle picture and analyzed with ImageJ software. The degradation of the SF films was detected by measuring weight loss in PBS. After immersing for 3, 5, 7, 14, and 21 d in sterile PBS at 37°C, the SF films were washed with distilled water, dried in an oven, and weighed. The weight loss (WL) was calculated according to the following equation:
where W0 was the initial weight and Wn was the dry weight of the SF films at different time intervals.
Adhesion and proliferation of hADSCs on SF films
hADSCs were purchased from Cyagen and cultured in Dulbecco’s modified Eagle’s medium (DMEM, Invitrogen) containing 10% fetal bovine serum (FBS, Gibco) and 1% penicillin/streptomycin (Beyotime). hADSCs at passage 3 were used in the following experiments. To measure the influence of the microscopic topology of the SF films on the proliferation of hADSCs, SF films from each group were cut into suitable size, sterilized under ultraviolet light, and immersed in culture medium for 30 min before being used in 96-well plates. Then, 5 × 103 cells/well hADSCs were seeded onto 96-well plates, and incubated for 1, 2, and 3 d at a 37°C incubator. The Cell Counting Kit-8 (CCK-8, Biosharp) was used to evaluate the cytotoxicity.
To observe the cellular adhesion and changes of hADSCs on the surface of the SF films, a custom-made punch was used to punch the SF films into a round shape of 12 mm in diameter firstly, and then sterilized under ultraviolet light before pre-culturing in DMEM at 37°C for 30 min. Finally, 100 uL of culture medium with 1 × 105 hADSCs was added to the pre-culture SF film in a 24-well plate and cultured for 2 h to allow adhesion before adding culture medium up to 2 mL. After 48 h culturing, the samples were collected for SEM observation, and transcriptome sequencing (Knorigene Technologies, China) was conducted to analysis the gene expression differences related to the differentiation of hADSCs on different SF films.
Immunofluorescence staining
hADSCs were seeded on ultraviolet light sterilized SF films with the same operation of cellular adhesion observation. After 48 h culturing, the SF films were fixed in 4% paraformaldehyde, blocked with the QuickBlock blocking buffer for 2 h in confocal dishes. For YAP distribution analysis, hADSCs seeded SF films were subsequently incubated with the YAP primary antibody (ab205270, Abcam) overnight at 4°C, followed by incubation with the secondary antibody (Rb-488, Beyotime) for 1 h at room temperature, and nuclei were stained with DAPI (C1002, Beyotime) for 15 min. For cytoskeleton change analysis, the films were incubated with the labeled F-actin antibody (R415, Invitrogen), and Vinculin antibody (26520‐1‐AP, Proteintech) with the secondary antibody (Rb-488, Beyotime), respectively, before DAPI staining. Finally, samples were observed by a ZEISS laser scanning confocal microscope (LSM780, Germany).
Western blot analysis
Total protein was extracted from the hADSCs using RIPA lysis buffer (Beyotime) supplemented with protease-phosphatase inhibitors (Roche) on ice for 20 min, followed by centrifugation at 12,000 g for 30 min at 4°C. Protein concentration was determined using BCA protein assay kit (Beyotime). Next, 50 ng of protein was separated by SDS-PAGE (Beyotime) and subsequently transferred to PVDF membrane (Millipore). The membranes were blocked with QuickBlock blocking buffer overnight at 4°C and then incubated overnight at 4°C with the primary antibodies of Osteonectin (ab290636, abcam), Osteopontin (ab63856, abcam), ACAN (NB600–504, Novus) and SOX-9 (NBP1–85551, Novus), followed by incubation with respective secondary antibodies for 1 h at room temperature. After washing three times with TBST, the membranes were visualized using SuperSignal west femto kit (ThermoFisher).
Co-culturing of human OA knee cartilage with hADSCs seeded SF films
The knee articular cartilage was obtained from OA patients (Kellgren–Lawrence grading IV) and rinsed with sterilized PBS. hADSCs were seeded onto the SF films as described above and the cell side of SF film was attached to the surface of the isolated OA cartilage. After culturing in chondrogenic induction medium (GUXMD-90041, Cyagen) for 7 d, SF films were removed and OA cartilage was paraffin sectioned for hematoxylin-eosin (HE) staining, safranin O staining and immunohistochemistry staining of Col-II.
Statistical analysis
Data were expressed as mean ± SD by using Prism 9.0 software (GraphPad Prism). Two different groups were compared by the independent sample t-test and multiple group comparisons were performed by one-way ANOVA with Tukey’s post hoc test. In all cases, results were considered statistically significant when p < .05.
Results and discussion
Morphology and hydrophilia of the SF films
PDMS molds were prepared based on different type specification sandpapers to ensure that all the obtained SF films had the same microscopic surface texture within the same group. shows the custom-made PDMS molds, and representative pictures of a SF film detaching from its mold after ethanol cross-linking treatment. The 2D and 3D surface microstructure of the SF films were analyzed by SEM and optical profilometry, respectively. As shown in , the SF films represented convex dot-featured topography which were similar to their sandpaper molds, that the diameter of the convex dots decreased gradually form 50 μm of P280 SF films to 3 μm of P7000 SF films. It was reported that most cells could response to micro-environment topologic changes within 10 nm–100 μm scale (Nabizadeh et al. Citation2022; Yang et al. Citation2020). For 3D topography, the P280 SF films had significantly higher convex dots with wider gaps than other groups (). The average height and space between dots decreased with the increase of dots number from P280 to P7000. The microscopic surface may affect the hydrophilia of the SF films, because rough surface can lead to the formation of air pockets that water droplets fail to contact adequately with the film surface (Cho et al. Citation2020). This was confirmed by our water contact angle results (), P280 SF films had water contact angle of 94.97 ± 1.87°, and increased to 100.93 ± 0.97°, 103.8 ± 0.30°, 116.67 ± 1.93°, and 123.46 ± 0.46° for P1000−P7000 SF films, respectively. In contrast, the degradation rate of the SF films saw an opposite trend compared with hydrophilia, as the weight loss of SF films increased with the density increment of dots (). This might be a result of surface area enlargement, that hydrolyzation could went on more sufficiently (Konganapuram Narasimma Bharathi et al. Citation2022; Uddin et al. Citation2021).
Figure 2. Characterization of the SF films.

Cellular adhesion and cytotoxicity of the SF films
As a subtype of MSCs, hADSCs are relatively more accessible than bone marrow MSCs, with repeatable access to the subcutaneous adipose tissue and easy enzyme-based isolation procedures, which have been reported to be used as seed cells for cartilage repair (Liao et al. Citation2022; Naderi et al. Citation2017). The early adhesion is a prerequisite for hADSCs proliferation, migration and subsequent differentiation behavior on the patterned surface of SF films (Ma et al. Citation2019). After 48 h culturing, hADSCs were found to attach well on the SF films with pseudopodia in the cell periphery, indicating migration capacity of the cells (). Apart from the outstanding biocompatibility, the exhibition of arginine-glycine-aspartic acid (RGD) sequences in SF was reported to enhance cell adhesion (Zou et al. Citation2022), and hence SF was widely used in coating for metal orthopedic implants to promote osseointegration by facilitating cell adhesion (Wenhao et al. Citation2020; W. Zhou et al. Citation2023). As a versatile material, SF films can also be prepared by electrospinning and weft knitting methods, and it would be interesting to compare which fabrication technique was more suitable for cell adhesion (Khademolqorani et al. Citation2021). The close-up pictures showed that the cells exhibited more flattened and cycloidal morphology on the 280-, 1000-, and 2000-SF films, whereas spindle-shaped and small in size on 5000- and 7000-SF films, suggesting hADSCs tend to undergo diverse directions of development, as the remodeling of cytoskeleton is closely related to the differentiation of MSCs (D Huang et al. Citation2023; Qian et al. Citation2017). The proliferation ability of hADSCs cultured on the patterned surfaces of SF films was detected using the CCK-8 method at 24 h, 48 h, and 72 h. As shown in , hADSCs proliferation was enhanced with the increase of dot density, and significant difference (p < .05) was found when comparing 280 group with 5000 or 7000 group, throughout the three detection time points. In addition to hADSCs, Karahaliloglu et al. found that dermal fibroblast cells also showed a higher adherence and proliferation rate on nanostructured SF films than smooth films (Karahaliloğlu et al. Citation2015), indicating that the microscopic surface pattern SF film may offer a promising way for cell amplification in vitro. Taken the cellular adhesion graphs and proliferation data together, we might speculate that hADSCs tend to undergo differentiation process on 280, 1000, and 2000 SF films, while amplification on 5000 and 7000 films.
Figure 3. Adhesion and proliferation of hADSCs on the SF films.

Transcriptomics analysis of hADSCs differentiation on the SF films
Based on the morphology and proliferation differences of hADSCs observed on different SF films, transcriptome sequencing was conducted to further analysis the underlying transcriptional mechanism. Representative genes related to adipogenic, chondrogenic, and osteogenic differentiation of hADSCs were analyzed first. As shown in the heatmap of , hADSCs had stronger osteogenic gene (mainly MMP2, OPN3, and RUNX2) expression on 280-SF films, relatively better chondrogenic gene (mainly Col2A1, SOX9) expression in 1000 group, and better adipogenic gene (ANXA1, CCL5) in 2000 group. Besides, no clear differentiation inclination was observed in 5000 or 7000 group, that cell proliferation related genes were analyzed in to verify the hypothesis that 280–2000-SF films would promote the differentiation of hADSCs, and 5000–7000-SF films could support hADSCs proliferation. As expected, MSLN, PCNA, and CDKN2A expression were lower in 280–2000 groups compared with 5000–7000 groups, which interpreted the outcome that hADSCs proliferated much better on 5000 and 7000 SF films.
Figure 4. Transcriptomics analysis of hADSCs differentiation on the SF films.

The substrate topographic structure induced MSCs differentiation was mainly mediated by integrin associated cell to extracellular matrix (ECM) adhesion events and downstream cytoskeleton remodeling (Larsen et al. Citation2006; Oliver-De La Cruz et al. Citation2019). One of the most classic pathways is integrin induced actin cytoskeleton remodeling and downstream Hippo/YAP regulated cell differentiation in MSCs (Feng et al. Citation2020; Nardone et al. Citation2017). Where substrate topology as a mechanical cue was recognized by specific integrins on the cell membrane, which inhibited F-actin tension and activated Hippo cascade to direct the differentiation of MSCs by regulating YAP/TAZ function (Dupont et al. Citation2011). As shown in the analysis results of , much more up-regulated integrin expression was observed in 280 group and 1000 group than 2000–7000 groups, and the downstream Hippo/YAP pathway was further analyzed. Along with the up regulation of integrins, LATS1 and subsequent YAP1 was up-regulated in 280 group and 1000 group (). YAP-1 was reported to form when YAP was dephosphorylated by LATS1 and transferred into the nucleus to perform transcriptional functions (Varelas Citation2014), indicating that P280 and P1000 SF films may exist better ability in directing hADSCs differentiation through YAP/TAZ. On the contrary, hADSCs on P2000–7000 SF films may receive positive signals of proliferation, and hence amplified.
Physical guidance by topographical patterns in regulating cellular behavior could avoid the problems of chemical cues, such as the high cost but short half-life of growth factors, and the potential adverse impact of overdose (Yoon et al. Citation2016). Therefore, a variety of materials including hydrogels, PDMS, Ti, silica and oxide ceramics have been utilized to fabricate specific micro/nanopatterns to mimic the mechanical behavior of native tissues for tissue engineering purpose (Tran and Kumar Citation2021). The frequently used techniques for patterning on the materials mainly included photolithography, soft lithography, two-photon lithography, etc. with features of grooves, pits, pillars and other customized patterns (Zhang et al. Citation2019). The results of this study indicated that the casting-drying method used to prepare SF films offered a cost-effective way to achieve gradient microscopic surface, although the definition of convex dots was less accurate than lithography.
Immunofluorescence staining and western blot analysis
According to the transcriptomics analysis results, detailed variations in actin cytoskeleton remodeling and YAP distribution were detected by IF staining and WB. The IF staining photographs showed that the F-actin of MSCs cultured on 280 and 1000 group SF films was weakened compared with 2000–7000 groups (), in contrast to that of vinculin expression. Besides, hADSCs on the 280 and 1000 group SF films had relative higher fluorescence intensity of intranuclear YAP-1 (), indicating the negative correlation between cytoskeleton tension and dephosphorylated YAP aggregation. Furthermore, the expression of chondrogenic and osteogenic markers were detected by WB. hADSCs on the 280 group SF films expressed more osteogenic proteins of osteonectin and osteopontin, and expressed more chondrogenic proteins of SOX-9 and ACAN on the 1000 group SF films, compared to 2000–7000 groups, which showed low differentiation symptom (). As reported, the transcriptional activity of Yap/Taz may affect cell behavior in various ways, depending on the developmental stage and the cell/tissue type. For instance, F-actin accumulation caused by loss-of-function of capping proteins in Drosophila third instar wing imaginal discs could led to cell proliferation and tissue overgrowth (Fernández et al. Citation2011). For another, rearrangement of actin cytoskeleton in response to the variation of ECM stiffness, regulated the Yap/Taz activity, directing cell differentiation of human hADSCs to specific cell lineages (Dupont et al. Citation2011).
Figure 5. If staining and WB detection on the cytoskeleton remodeling and YAP distribution.

Histological analysis of explant co-culture
Since hADSCs on the SF films presented directed differentiation property, the hADSCs seeded SF films were used for cartilage repair assay, by co-culturing with cartilage explants obtained from OA patients undergoing surgery. Similarly, Baboolal et al. had used this method to study the synovial fluid hyaluronan mediated MSCs attachment to cartilage (Baboolal et al. Citation2016). As shown in the HE staining results, cartilage explant cultured with hADSCs loaded 1000-SF film had a smoother surface, compared with other groups (). For the cartilage matrix of proteoglycan, 1000 group also showed stronger safranine O staining intensity (). Consistently, the distribution of Col-II in 1000 group was also superior than the other groups (), indicating that hADSCs loaded 1000 SF film had best cartilage repair capability as a CTE scaffold.
Figure 6. Histologic analysis of cartilage protection effect after explant co-culture.

Conclusions
In this study, SF films with gradient convex dot diameter of 3–50 μm were fabricated by using a convenient template of sandpapers, and proved that surface patterned SF films were promising CTE scaffold in supporting MSCs amplification and chondrogenic differentiation. hADSCs were found to differentiate differently on the SF films through extracellular matrix mediated Hippo/Yap pathway. In vitro study preliminarily indicated that hADSCs seeded 1000 SF films are promising for cartilage repair. However, the present study had some limitation. Firstly, the mechanical property can be changed along with the topological alternation of SF film surface, that the mechanism in directing hADSCs differentiation might be multifactorial cascade, apart from the Hippo/Yap pathway. Secondly, the cartilage repair ability of the hADSCs loaded SF film was only tested in vitro, within a short experiment period, that long time in vivo performance of degradation and integration should be explored.
Highlights
The biocompatible silk fibroin (SF) films with gradient microscopic surface patterns were prepared via casting SF solution on PDMS molds derived from type specification sandpapers.
The growth factor-free, convex dot featured SF film regulates chondrogenic differentiation in hADSCs through Hippo/YAP pathway.
The growth factor-free SF films may serve as a novel functional bioscaffold for articular cartilage regeneration.
Institutional review board statement
This study was approved by the Ethics Committee of the First Affiliated Hospital of Army Medical University, PLA (No.KY2021040).
Acknowledgment
We thank the technical support in bioinformatics analysis offered by Knorigene Technologies.
Disclosure statement
No potential conflict of interest was reported by the author(s).
Data availability statement
The data presented in this study are available on request from the corresponding author.
Additional information
Funding
References
- Armiento, A. R., M. J. Stoddart, M. Alini, and D. Eglin. 2018. “Biomaterials for Articular Cartilage Tissue Engineering: Learning from Biology.” Acta Biomaterialia 65:1–13. https://doi.org/10.1016/j.actbio.2017.11.021.
- Baboolal, T. G., S. C. Mastbergen, E. Jones, S. J. Calder, F. P. Lafeber, and D. McGonagle. 2016. “Synovial Fluid Hyaluronan Mediates MSC Attachment to Cartilage, a Potential Novel Mechanism Contributing to Cartilage Repair in Osteoarthritis Using Knee Joint Distraction.” Annals of the Rheumatic Diseases 75 (5): 908–915. https://doi.org/10.1136/annrheumdis-2014-206847.
- Cao, B., Y. Peng, X. Liu, and J. Ding. 2017. “Effects of Functional Groups of Materials on Nonspecific Adhesion and Chondrogenic Induction of Mesenchymal Stem Cells on Free and Micropatterned Surfaces.” ACS Applied Materials & Interfaces 9 (28): 23574–23585. https://doi.org/10.1021/acsami.7b08339.
- Carthew, J., H. H. Abdelmaksoud, M. Hodgson-Garms, S. Aslanoglou, S. Ghavamian, R. Elnathan, J. P. Spatz, et al. 2021. “Precision Surface Microtopography Regulates Cell Fate via Changes to Actomyosin Contractility and Nuclear Architecture.” Advanced Science (Weinheim, Baden-Wurttemberg, Germany) 8 (6): 2003186. https://doi.org/10.1002/advs.202003186.
- Cheng, G., X. Wang, M. Wu, S. Wu, L. Cheng, X. Zhang, F. Dai, et al. 2022. “Insignificant Difference in Biocompatibility of Regenerated Silk Fibroin Prepared with Ternary Reagent Compared with Regenerated Silk Fibroin Prepared with Lithium Bromide.” Polymers 14 (18): 3903. https://doi.org/10.3390/polym14183903.
- Cho, E., M. Kim, J. S. Park, and S. J. Lee. 2020. “Plasma-Polymer-Fluorocarbon Thin Film Coated Nanostructured-Polyethylene Terephthalate Surface with Highly Durable Superhydrophobic and Antireflective Properties.” Polymers 12 (5): 1026. https://doi.org/10.3390/polym12051026.
- Cleary, M. A., G. J. van Osch, P. A. Brama, C. A. Hellingman, and R. Narcisi. 2015. “FGF, TGFβ and Wnt Crosstalk: Embryonic to in vitro Cartilage Development from Mesenchymal Stem Cells.” Journal of Tissue Engineering and Regenerative Medicine 9 (4): 332–342. https://doi.org/10.1002/term.1744.
- Dinoro, J., M. Maher, S. Talebian, M. Jafarkhani, M. Mehrali, G. Orive, J. Foroughi, et al. 2019. “Sulfated Polysaccharide-Based Scaffolds for Orthopaedic Tissue Engineering.” Biomaterials 214:119214. https://doi.org/10.1016/j.biomaterials.2019.05.025.
- Dupont, S., L. Morsut, M. Aragona, E. Enzo, S. Giulitti, M. Cordenonsi, F. Zanconato, et al. 2011. “Role of YAP/TAZ in Mechanotransduction.” Nature 474 (7350): 179–183. https://doi.org/10.1038/nature10137.
- Feng, Q., H. Gao, H. Wen, H. Huang, Q. Li, M. Liang, Y. Liu, et al. 2020. “Engineering the Cellular Mechanical Microenvironment to Regulate Stem Cell Chondrogenesis: Insights from a Microgel Model.” Acta Biomaterialia 113:393–406. https://doi.org/10.1016/j.actbio.2020.06.046.
- Fernández, B. G., P. Gaspar, C. Brás-Pereira, B. Jezowska, S. R. Rebelo, and F. Janody. 2011. “Actin-Capping Protein and the Hippo Pathway Regulate F-Actin and Tissue Growth in Drosophila.” Development 138 (11): 2337–2346. https://doi.org/10.1242/dev.063545.
- Ghandforoushan, P., J. Hanaee, Z. Aghazadeh, M. Samiei, A. M. Navali, A. Khatibi, S. Davaran, et al. 2022. “Novel Nanocomposite Scaffold Based on Gelatin/PLGA-PEG-PLGA Hydrogels Embedded with TGF-β1 for Chondrogenic Differentiation of Human Dental Pulp Stem Cells in vitro.” International Journal of Biological Macromolecules 201:270–287. https://doi.org/10.1016/j.ijbiomac.2021.12.097.
- He, Y., Y. Yu, Y. Yang, Y. Gu, T. Mao, Y. Shen, Q. Liu, et al. 2022. “Design and Aligner-Assisted Fast Fabrication of a Microfluidic Platform for Quasi-3D Cell Studies on an Elastic Polymer.” Bioactive Materials 15:288–304. https://doi.org/10.1016/j.bioactmat.2021.12.010.
- Huang, B. J., J. C. Hu, and K. A. Athanasiou. 2016. “Cell-Based Tissue Engineering Strategies Used in the Clinical Repair of Articular Cartilage.” Biomaterials 98:1–22. https://doi.org/10.1016/j.biomaterials.2016.04.018.
- Huang, D., Y. Li, Z. Ma, H. Lin, X. Zhu, Y. Xiao, X. Zhang, et al. 2023. “Collagen Hydrogel Viscoelasticity Regulates MSC Chondrogenesis in a ROCK-Dependent Manner.” Science advances 9 (6): eade9497. https://doi.org/10.1126/sciadv.ade9497.
- Karahaliloğlu, Z., B. Ercan, E. B. Denkbaş, and T. J. Webster. 2015. “Nanofeatured Silk Fibroin Membranes for Dermal Wound Healing Applications.” Journal of Biomedical Materials Research Part A 103 (1): 135–144. https://doi.org/10.1002/jbm.a.35161.
- Khademolqorani, S., H. Tavanai, I. S. Chronakis, A. Boisen, and F. Ajalloueian. 2021. “The Determinant Role of Fabrication Technique in Final Characteristics of Scaffolds for Tissue Engineering Applications: A Focus on Silk Fibroin-Based Scaffolds.” Materials Science & Engineering C, Materials for Biological Applications 122:111867. https://doi.org/10.1016/j.msec.2021.111867.
- Kim, Y. S., and A. G. Mikos. 2021. “Emerging Strategies in Reprogramming and Enhancing the Fate of Mesenchymal Stem Cells for Bone and Cartilage Tissue Engineering.” Journal of Controlled Release: Official Journal of the Controlled Release Society 330:565–574. https://doi.org/10.1016/j.jconrel.2020.12.055.
- Konganapuram Narasimma Bharathi, S. S., V. Adiga, S. Khasnabis, B. Nath, N. A. Khan, and P. C. Ramamurthy. 2022. “Study of Nano Cellulose-Based Membrane Tailorable Biodegradability for Use in the Packaging Application of Electronic Devices.” Chemosphere 309 (Pt 2): 136683. https://doi.org/10.1016/j.chemosphere.2022.136683.
- Larsen, M., V. V. Artym, J. A. Green, and K. M. Yamada. 2006. “The Matrix Reorganized: Extracellular Matrix Remodeling and Integrin Signaling.” Current Opinion in Cell Biology 18 (5): 463–471. https://doi.org/10.1016/j.ceb.2006.08.009.
- Liao, H., Q. Tu, Y. Kang, G. Mao, Z. Li, S. Hu, P. Sheng, et al. 2022. “CircNFIX Regulates Chondrogenesis and Cartilage Homeostasis by Targeting the miR758-3p/KDM6A Axis.” Cell Proliferation 55 (11): e13302. https://doi.org/10.1111/cpr.13302.
- Liu, F., X. Wang, Y. Li, M. Ren, P. He, L. Wang, J. Xu, et al. 2022. “Dendrimer-Modified Gelatin Methacrylate Hydrogels Carrying Adipose-Derived Stromal/Stem Cells Promote Cartilage Regeneration.” Stem Cell Research & Therapy 13 (1): 26. https://doi.org/10.1186/s13287-022-02705-6.
- Ma, C., M. L. Kuzma, X. Bai, and J. Yang. 2019. “Biomaterial-Based Metabolic Regulation in Regenerative Engineering.” Advanced science (Weinheim, Baden-Wurttemberg, Germany) 6 (19): 1900819. https://doi.org/10.1002/advs.201900819.
- Nabizadeh, Z., M. Nasrollahzadeh, H. Daemi, M. Baghaban Eslaminejad, A. A. Shabani, M. Dadashpour, M. Mirmohammadkhani, et al. 2022. “Micro- and Nanotechnology in Biomedical Engineering for Cartilage Tissue Regeneration in Osteoarthritis.” Beilstein Journal of Nanotechnology 13:363–389. https://doi.org/10.3762/bjnano.13.31.
- Naderi, N., E. J. Combellack, M. Griffin, T. Sedaghati, M. Javed, M. W. Findlay, C. G. Wallace, et al. 2017. “The Regenerative Role of Adipose-Derived Stem Cells (ADSC) in Plastic and Reconstructive Surgery.” International Wound Journal 14 (1): 112–124. https://doi.org/10.1111/iwj.12569.
- Nardone, G., J. Oliver-De La Cruz, J. Vrbsky, C. Martini, J. Pribyl, P. Skládal, M. Pešl, et al. 2017. “YAP Regulates Cell Mechanics by Controlling Focal Adhesion Assembly.” Nature communications 8 (1): 15321. https://doi.org/10.1038/ncomms15321.
- Oliver-De La Cruz, J., G. Nardone, J. Vrbsky, A. Pompeiano, A. R. Perestrelo, F. Capradossi, K. Melajová, P. Filipensky, and G. Forte. 2019. “Substrate Mechanics Controls Adipogenesis Through YAP Phosphorylation by Dictating Cell Spreading.” Biomaterials 205:64–80. https://doi.org/10.1016/j.biomaterials.2019.03.009.
- Qian, W., L. Gong, X. Cui, Z. Zhang, A. Bajpai, C. Liu, A. B. Castillo, et al. 2017. “Nanotopographic Regulation of Human Mesenchymal Stem Cell Osteogenesis.” ACS Applied Materials & Interfaces 9 (48): 41794–41806. https://doi.org/10.1021/acsami.7b16314.
- Rastogi, P., and B. Kandasubramanian. 2019. “Review of Alginate-Based Hydrogel Bioprinting for Application in Tissue Engineering.” Biofabrication 11 (4): 042001. https://doi.org/10.1088/1758-5090/ab331e.
- Sahoo, J. K., O. Hasturk, T. Falcucci, and D. L. Kaplan. 2023. “Silk Chemistry and Biomedical Material Designs.” Nature Reviews Chemistry 7 (5): 302–318. https://doi.org/10.1038/s41570-023-00486-x.
- Tang, Z., X. Wang, J. Yang, X. Song, Y. Huang, C. Chen, H. Yang, et al. 2021. “Microconvex Dot-Featured Silk Fibroin Films for Promoting Human Umbilical Vein Endothelial Cell Angiogenesis via Enhancing the Expression of bFGF and VEGF.” ACS Biomaterials Science & Engineering 7 (6): 2420–2429. https://doi.org/10.1021/acsbiomaterials.0c01647.
- Tran, V. D., and S. Kumar. 2021. “Transduction of Cell and Matrix Geometric Cues by the Actin Cytoskeleton.” Current Opinion in Cell Biology 68:64–71. https://doi.org/10.1016/j.ceb.2020.08.016.
- Trucco, D., L. Vannozzi, E. Teblum, M. Telkhozhayeva, G. D. Nessim, S. Affatato, H. Al‐Haddad, et al. 2021. “Graphene Oxide-Doped Gellan Gum–PEGDA Bilayered Hydrogel Mimicking the Mechanical and Lubrication Properties of Articular Cartilage.” Advanced Healthcare Materials 10 (12): e2100873. https://doi.org/10.1002/adhm.202100873.
- Uddin, M. G., B. J. Allardyce, N. Rashida, and R. Rajkhowa. 2021. “Mechanical, Structural and Biodegradation Characteristics of Fibrillated Silk Fibres and Papers.” International Journal of Biological Macromolecules 179:20–32. https://doi.org/10.1016/j.ijbiomac.2021.02.211.
- van der Kraan PM, and P. M. van der Kraan. 2017. “The Changing Role of TGFβ in Healthy, Ageing and Osteoarthritic Joints.” Nature Reviews Rheumatology 13 (3): 155–163. https://doi.org/10.1038/nrrheum.2016.219.
- Varelas, X. 2014. “The Hippo Pathway Effectors TAZ and YAP in Development, Homeostasis and Disease.” Development (Cambridge, England) 141 (8): 1614–1626. https://doi.org/10.1242/dev.102376.
- Wenhao, Z., T. Zhang, J. Yan, Q. Li, P. Xiong, Y. Li, Y. Cheng, et al. 2020. “In vitro and in vivo Evaluation of Structurally-Controlled Silk Fibroin Coatings for Orthopedic Infection and in-Situ Osteogenesis.” Acta Biomaterialia 116:223–245. https://doi.org/10.1016/j.actbio.2020.08.040.
- Xu, W., W. Wang, D. Liu, and D. Liao. 2022. “Roles of Cartilage-Resident Stem/Progenitor Cells in Cartilage Physiology, Development, Repair and Osteoarthritis.” Cells 11 (15): 2305. https://doi.org/10.3390/cells11152305.
- Yang, L., K. M. Jurczak, L. Ge, and P. van Rijn. 2020. “High-Throughput Screening and Hierarchical Topography-Mediated Neural Differentiation of Mesenchymal Stem Cells.” Advanced Healthcare Materials 9 (11): e2000117. https://doi.org/10.1002/adhm.202000117.
- Yao, X., X. Wang, and J. Ding. 2021. “Exploration of Possible Cell Chirality Using Material Techniques of Surface Patterning.” Acta Biomaterialia 126:92–108. https://doi.org/10.1016/j.actbio.2021.02.032.
- Yoon, J. K., H. N. Kim, S. H. Bhang, J. Y. Shin, J. Han, W. G. La, G.-J. Jeong, et al. 2016. “Enhanced Bone Repair by Guided Osteoblast Recruitment Using Topographically Defined Implant.” Tissue engineering Part A 22 (7–8): 654–664. https://doi.org/10.1089/ten.tea.2015.0417.
- Zhang, K., X. Xiao, X. Wang, Y. Fan, and X. Li. 2019. “Topographical Patterning: Characteristics of Current Processing Techniques, Controllable Effects on Material Properties and Co-Cultured Cell Fate, Updated Applications in Tissue Engineering, and Improvement Strategies.” Journal of Materials Chemistry B 7 (45): 7090–7109. https://doi.org/10.1039/C9TB01682A.
- Zhou, W., T. Bai, L. Wang, Y. Cheng, D. Xia, S. Yu, Y. Zheng, et al. 2023. “Biomimetic AgNps@antimicrobial Peptide/Silk Fibroin Coating for Infection-Trigger Antibacterial Capability and Enhanced Osseointegration.” Bioactive materials 20:64–80. https://doi.org/10.1016/j.bioactmat.2022.05.015.
- Zhou, Z., J. Cui, S. Wu, Z. Geng, and J. Su. 2022. “Silk Fibroin-Based Biomaterials for Cartilage/Osteochondral Repair.” Theranostics 12 (11): 5103–5124. https://doi.org/10.7150/thno.74548.
- Zou, S., X. Yao, H. Shao, R. L. Reis, S. C. Kundu, and Y. Zhang. 2022. “Nonmulberry Silk Fibroin-Based Biomaterials: Impact on Cell Behavior Regulation and Tissue Regeneration.” Acta Biomaterialia 153:68–84. https://doi.org/10.1016/j.actbio.2022.09.021.