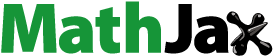
ABSTRACT
The most crucial variables that influence the characteristics and quality of cotton fibers during the cotton growth and ginning processes are temperature and moisture regains. Here the cotton fiber samples were generated with pre-treatment using a synergistic strategy of moisture regains and low temperatures, the morphology and structure of the fibers with different pre-treatments were analyzed by using scanning electron microscopy (SEM), Fourier transform infrared spectroscopy (FTIR), X-ray Photoelectron Spectrometer (XPS), X-ray diffraction (XRD), and Carbon-13 Nuclear Magnetic Resonance Spectrometry (13C NMR). Further, the mechanical properties of the cotton fibers were evaluated using LLY-06E tensile. The results shown that, with 3 days of freezing in -18℃, the linear density variation of the cotton fiber samples reached maximum, and the surface of the fibers showed noticeable fractures, local holes, and folded damage. Moreover, the low temperature and high moisture regain caused the internal water molecules of the cotton fibers interact in complex ways, altering the intermolecular forces but without changing the chemical composition. This research expands the temperature field of cotton fibers, particularly in the ginning process, by investigating the damage to the mechanical characteristics of cotton fibers caused by the combined effect of low temperature and moisture regain.
摘要
在棉花生长和轧棉过程中,影响棉纤维特性和质量的最关键变量是温度和回潮率。在这里,使用回潮和低温的协同策略对棉纤维样品进行了预处理,并使用扫描电子显微镜(SEM)、傅立叶变换红外光谱(FTIR)、X 射线光电子能谱仪(XPS)、X 射线衍射(XRD)和碳 13 核磁共振谱(13CNMR)分析了经过不同预处理的纤维的形态和结构。此外,还使用 LLY-06E 拉伸法评估了棉纤维的机械性能。结果表明,在-18℃下冷冻 3 天后,棉纤维样品的线性密度变化达到最大值,纤维表面出现明显的断裂、局部孔洞和折叠损伤。此外,低温和高回潮导致棉纤维内部水分子发生复杂的相互作用,改变了分子间的作用力,但没有改变化学成分。这项研究通过研究低温和回潮共同作用对棉纤维机械特性造成的破坏,拓展了棉纤维的温度领域。
Introduction
Cotton is a well-liked natural textile material, due to its outstanding physical and chemical properties, cotton fibers are widely used. Cotton has been part of daily life for many millennia, but our understanding of its nature remains incomplete. First, the processability of cotton fiber is closely related to the variety, followed by the process of growth and production by the environmental temperature and humidity, for example, cotton fiber has a high correlation with temperature in the growth and maturity stage (Gipson and Joham Citation1968; Rousselle, Thibodeaux, and French Citation2005). In addition, in order to ensure the quality of cotton fiber, cotton ginning enterprises must ensure the ginning environment during the processing within an appropriate range (Cao and Xu Citation2022; Cao et al. Citation2021; Patil, Madhukar Padole, and Fulchand Agrawal Citation2007). The reality, however, is that the fiber quality of grown fibrils and ginning were as reflections of their moisture regains (MR) and temperature (Anthony Citation1994; Tesema and Drieling Citation2020). There was extensively studied rationale for cotton fiber conditioned to a standard MR and temperature prior to testing, and for ginning to reduce fiber damage. However, the performance of cotton fibers in harsh environments has emerged as a research hotspot, especially in the cotton picking and ginning process. Through actual cotton harvesting and ginning production research, the cotton fibers ginning companies process fibers under extreme conditions (TEMP ≥ −10°C, MR ≥ 12%) leads to quality decrease (Cao et al. Citation2021). Consequently, it is crucial to study the mechanisms behind cotton fibers mechanical property deterioration caused by harsh environments.
In general, the researchers believe that changes in temperature or MR can affect the structure of cotton fibers (Atalie et al. Citation2022; Cevheri Citation2022), which were the single strategies have been carried out to characterize the fiber properties and explore the effects in harsh environmental (Leitgeb and Wakeham Citation1954; Shizuo and EMORI Citation1993), for instance, subfreezing temperatures, extremely low temperatures, absorbed water (Barker et al. Citation2001; Hart et al. Citation1955; Nakamura, Hatakeyama, and Hatakeyama Citation1981), etc. Moreover, the alterations in the lattice structure, crystallinity, grain size, and O-H groups of cellulose I were investigated, along with the thermal, mechanical, and antibacterial properties of cellulose fibers, by using differential scanning calorimetry (DSC), XRD, and NMR (Atalla Citation1978; Martin and Haigler Citation2004; Singh et al. Citation1969; Zelinka et al. Citation2012), respectively, these parameters impacting fiber qualities were identified and examined. Furthermore, the microstructure and crystal changes of the supercooled water were observed, and in the 6th stage, the water droplets broke and produced ice-crystals, which caused internal damage to the microfibril (Kalita et al. Citation2023; Powell-Palm, Rubinsky, and Sun Citation2020). Studies have shown that harsh environments impact on the mechanical properties of cotton fibers, including the fracture morphology that was mirrored in the molecular structure of the fiber (Cintrón and Ingber Citation2013). In particular, water freezing was the process of ice crystallization from supercooled water (Z. S. Zhang and Liu Citation2018), the study by (Nakamura, Hatakeyama, and Hatakeyama Citation1981) demonstrated that three different types of water-free water, freezing-bound water, and nonfreezing bound water-exist in the adsorbed water on cellulose samples and attach to the hydroxyl groups of their binding state. However, structure damaged on cotton fiber via coupling effect of moisture regains and low temperature have rare been studied, here the research on the characterization of fiber mechanical properties through the pre-treatment with moisture conditioning – low temperature – rewarming can be closer to actual production under harsh conditions, which also increases the correlation between the environment and actual production.
In our previous work (Cao and Xu Citation2022; Cao et al. Citation2021), the problem of fiber qualities in actual production was shown by the first data gathered through field research. As a result, the effects of moisture regains and low-temperature on cotton fibers were examined in this work. In particular, the test results of cotton fiber with moisture regain of 75% and 8 days are as expected, that significant differences in surface morphology, structure, and mechanical properties. In addition, cotton fiber test samples apparent structure, mechanical characteristics, and macromolecular structure were identified and examined by using FTIR (Abidi, Cabrales, and Haigler Citation2014), XRD (Atalla and Nagel Citation1974; Yue Citation2011), XPS (Johansson and Campbell Citation2004; Johansson et al. Citation2004), 13C NMR (Svanson Citation1978; Zhao et al. Citation2007), and LLY-06E. The damage mechanism was also clarified, which the findings indicated that the native fibrils on both the fibers surface and inside were destroyed, along with the fibers crystalline structure, mechanism, and stability of internal aggregate state structure. It was hoped that the study would avoid harvesting and processing of cotton fibers under harsh conditions of temperature and moisture regain.
Materials and methods
Materials
Cotton fibers with a fiber density of 1.54 g/cm3, average linear density 2.16 dtex, average fiber length of 28.6 mm, and maturity degree is 4.6, sourced from cotton processing factory in Beiquan Town, Shihezi were used for this study.
Experimental details
All experimental procedures were conducted using standard testing methods. The process for pre-treating moisture conditioning-low temperature-rewarming was outlined as follows: Firstly, 30 g of cotton fiber was weighed using a BT25S electronic balance. Next, EquationEquation 1(1)
(1) , which calculates the MR, was used to determine the appropriate amount of deionized water to the cotton fiber in a self-sealing sample bag. After blending the cotton fiber samples, the moisture-adjusted samples were generated with in-situ water molecules and cotton fiber-free moisture absorption proportions of 8.5%, 25%, 50%, 75%, and 100%. These different moistures regain proportions were advantages for examining morphological traits and mechanical qualities. The hygroscopicity in this method follows Peirce’s binomial theory of hygroscopicity (Peirce Citation1929), and EquationEquation 1
(1)
(1) is used to control the moisture regain.
where W and W0 were the wet and dry weights of cotton fibers (g), respectively.
Secondly, a variable-temperature freeze set to −18°C was used to freeze the moisture-regain sample that had been adjusted in the previous phase. The freezing times were 0, 1, 3, 5, and 8 days. The post-freezing morphology of the fibers and any potential mechanical damage were described. After being removed from the freezer in the second step, the samples were dried at a temperature of 80°C for a duration of 2 to 3 h.
Subsequently, the samples were cooled to a temperature of 20°C before being placed in a standard laboratory environment (20 ± 2°C, 65 ± 5%) for testing and characterization. The tests included the use of a single-fiber tensile strength tester LLY-06E, as well as FTIR, XRD, XPS, and13C NMR analyzes.
Measurement and characterization
All cotton fiber samples were placed in a standard constant temperature and humidity laboratory for 24 hours. The instruments were used to test and characterize cotton fibers included Fourier transform infrared spectroscopy (FTIR) (Nicolet5700, USA) to obtained the infrared absorption spectra of the fibers by irradiating the samples with different wavelengths of infrared light. The X-ray Photoelectron Spectrometer (XPS) (Escalab 250Xi, China) was selected to study the chemical elements and their chemical states contained in cotton fibers based on the XPS. Further, the X-ray diffraction (XRD) spectra of cotton fibers were obtained using a Bruker type X-ray diffractometer (D8 ADVANCE,). The structure of the aggregated state of cotton fiber polymers was studied using an NMR spectrometer (AVANCE400, USA) with a resonance frequency of 400 MHz, 13C resolution of 3.7 Hz, and 13C sensitivity of 49:1 a scanning electron microscope (SEM) (DXS-10ACKT, Japan) to observed the surface morphology at magnifications of 1000× and 3000 × . To understand the mechanical properties of the fibers after pre-treatment, an electronic single fiber strength tester (LLY-06E, China) was used to measure fracture strength and elongation at break.
Results and discussion
Linear density of the cotton fiber
To evaluate the changes with morphological and characteristics of cotton fibers, we measured the average diameter of various cotton fiber samples using Image Pro software. Additionally, we determined the linear density of the cotton fibers, the linear density did not significantly vary with an increase in the freezing day of low-temperature treatment. However, it exhibited a pattern of initial growth followed by a decline. The linear density reached its peak at 3 days, as shown in . Furthermore, as the moisture regains increased, the linear density of the cotton gradually increased, and this could be attributed to the cotton fiber porous structure, which enhances moisture absorption performance. The observation aligns with Peirce’s two-phase theory of moisture absorption (Peirce Citation1929), direct water absorption can disrupt the interaction between fiber macromolecules, thereby affecting the physical properties of the cotton fiber. Indirect water absorption, on the other hand, leads to an increase in the number of water molecules, causing swelling in non-crystalline areas and disintegration in weak crystalline areas. Consequently, this affects the morphological structure and mechanical properties of the fiber. The fineness of cotton fibers increases as they absorb moisture, with greater radial expansion compared to axial expansion. When cotton fibers are dried to remove absorbed water molecules, shrinkage occurs, but the change in orientation is retained.
Structure of the cotton fiber
The Hindeleh-Johnson multipeak resolution method (Hindeleh, Johnson, and Montague Citation1980) was used to achieve peak resolution of the corrected and normalized WAXS spectra. The least-squares difference between the calculated and observed intensities for each spectrum was minimized to obtain reliable parameters for measuring the crystallinity index and crystallite size (Sarna and Włochowicz Citation2003). presents the FTIR comparison graphs of various freezing days at 75% moisture regain, along with the cellulose fiber infrared spectra, to facilitate the analysis of changes in the macromolecular structure. By examining the location of the characteristic absorption peaks in the infrared spectra obtained from the experiments, which determine the type of chemical bonds or functional groups present in the samples. Moreover, there have been slight alterations in the typical absorption peaks of the C-O-H bending vibration at a wave number of 1038 cm−1 and the stretching vibration of the O-H bond at a wave number of 3328 cm−1. Additionally, the characteristic cotton fiber peaks at 1038 cm−1 and 3328 cm−1 exhibited a pattern of increasing, then decreasing, and finally increasing with the freezing day. This pattern can be attributed to the low-temperature freezing can damage the original structure of cotton fibrils, and the increase of moisture regain could enhanced the hydrogen bonds between cotton fiber macromolecules while leaving the structure of the macromolecules essentially unaltered (Singh et al. Citation1969).
Figure 2. Comparison of FTIR spectra of cotton fiber at 75% moisture regain with freezing day as a variable: (a) moisture regain of 75% with freezing day as a variable. (b) cotton fiber FTIR fractional peak plot cotton fiber fractional peak area ratio, the inset shows the hydrogen bonding characteristic peak area ratio.

Notably, FTIR research on cellulose fibers, the focus was on the 3650 ~ 3000 cm−1 band, which represents for the -OH stretching. The infrared absorption bands in the 3650 ~ 3000 cm−1 -OH stretching region were shown in . Using Origin 9.0 image processing software, the characteristic peaks were fitted to four fitted peaks by splitting the peaks with Gaussian functions at the following positions, i.e. 3440 cm−1 (O(2)H…O(6)), 3349 cm−1 (O(3)H…O(5)), 3277 cm−1 (O(6)H…O(3)), and 3201 cm−1 (O-H stretching), respectively. The total area of the fitted peaks S0 and the areas of the four fitted peaks Si (i = 1, 2, 3, 4) (Liu et al. Citation2018) can be used to determine the area ratio of each fitted peak, as shown in the following EquationEquation 2(2)
(2) .
As shown in inset of , the FTIR analysis of cotton fibers revealed characteristic peak area ratios of hydrogen bonding, with the freezing day as a variable. According to Igarashi’s research (Igarashi et al. Citation2020), in an unsaturated state, water vapor was the most stable phase and bound water can only exist in a thinner state. It was may be the hydrophobic effect of air on water molecules near the water–air interface strengthens their hydrogen bonds. As the freezing day of low-temperature treatment increased, the fitted peak area ratios at positions 3440 cm−1 (O(2)H…O(6)) and 3349 cm−1 (O(3)H…O(5)) shown a pattern of growth, followed by a decrease, and then an increase. The positions at 3277 cm−1 (O(3)H…O(5)) and 3201 cm−1 (O(3)H…O(5)) also exhibited an increasing and then declining pattern. These results suggest that the hydrogen bonds within cotton fiber molecules varied in response to changes in moisture regain. However, the primary intermolecular connections in cotton fibers were hydrogen bonds, when water penetrates, these hydrogen bonds break, leading to molecular a rapid rearrangement. Although the breakdown of hydrogen bonds during water adsorption had impacted on the structure of cotton fibers, the hydrogen bonds were subsequently repaired, stabilizing the molecular structure once more (Svanson Citation1978). Therefore, the findings of this experiment imply that the pre-treatment has an impact on the quantity of hydrogen bonds between macromolecules.
X-ray photoelectron spectroscopy (XPS) was used to determine the elements present in cotton fibers. depict the distinctive C1s and O1s peaks of the control sample and the sample subjected to 75% freezing for 8 days, at binding energies of 284.81 eV and 532.54 eV, respectively. The were shown the characteristic peaks of the XPS diagram of cotton fibers were not altered by the pre-treatment, which indicates the pre-treatment had no effect on the chemical characteristics of the cotton fibers. Additionally, carbon (C) and oxygen (O) were found to be the major elements in both cotton fiber samples.
Figure 3. Results of XPS tests performed on cotton fibers on various moisture regain and freezing day: (a) O1s scan. (b) C1s scan. (c) XPS spectra of cotton fibers. (d) Cotton fibers fractionated using XPS under typical circumstances. (e) Cotton fibers were fractionated using XPS under 75% freezing for 8 days. (f) Fitted peak area ratio for 8 days at 75% freezing and normal conditions.

To further understand the structure of C1s in cotton fibers, Gaussian function was used to analyze the splitting process. The splitting plots for the control sample and 8 days of freezing were shown in , respectively. Carbon atoms in cotton fibers were primarily divided into four binding forms: C1 (C-C/C-H), C2 (C-OH), C3 (O-C-O/C=O), and C4 (O=C-O), and the binding energies for these forms were 284.8, 285.3 ~ 286.8, 286.3 ~ 287.8, and 288.4 ~ 289.9 eV, respectively. The C-C form accounts for approximately 60% of the carbon content and was the main form of carbon present, followed by C-OH and C=O. The content of O=C-O was the lowest, at approximately 5%. C1 exists in the form of carbon atoms connected only to carbon or hydrogen atoms, resulting in a low electron binding energy, and C2 exists in the form of a carbon atom attached to a non-carbonyl-like oxygen atom, which had a higher electron binding energy than C1 due to the polar nature of the C-O bond. Further, C3 exists in the form of a carbon atom attached to two non-carbonyl-like oxygen atoms, resulting in high electron binding energy due to the high oxidation degree of the connection. Finally, C4 exists in the form of a carbonyl group attached to a carbon atom and a non-carbonyl oxygen atom (Johansson and Campbell Citation2004). The area ratio of the fitted peaks of the XPS carbon fraction of cotton fiber can be calculated using EquationEquation 2(2)
(2) , and the results are shown in . The findings also revealed the chemical bond types of the macromolecules on the surface of cotton fiber cellulose crystals, namely C1, C2, C3, and C4, remained the same after pre-treatment, but the ratio of each chemical bond changed significantly. Compared to the control sample, the cotton fiber sample frozen for 8 days showed an increase of 0.29% in C1 and 7.55% in C2, while the C3 and C4 decreased by 21.04% and 25.83%, respectively. This indicates that more carbon chains and hydroxyl groups appeared on the surface of the cotton fiber.
Crystallinity of the cotton fiber
The aggregated state structure of cellulose fibers consists of both crystalline and amorphous parts, rather than being completely crystalline or amorphous. Both the crystalline and amorphous parts of the aggregated state structure play important roles in determining their properties (Yu Citation2018). The crystalline forms of cellulose fibers include cellulose I, cellulose II, and cellulose III, among others (Agoda-Tandjawa et al. Citation2010).
From , it can be concluded that the X-ray diffraction curves obtained after pre-treatment shown a consistent trend, with no significant change in the position of the diffraction angle where the characteristic peaks were located. The X-ray diffraction curves of cotton fibers exhibit strong diffraction peaks near 14.91°, 16.56°, 22.80°, and 34.32°, corresponding to the ī10, 101, 002, and 400 crystalline planes, respectively. The 002 crystal has shown the strongest diffraction, while the 400 crystal has shown the weakest diffraction. These results indicate that the characteristic peaks of the cotton fiber pre-treatment were close to the theoretically calculated values for cellulose I, suggesting the crystalline form of the cotton fiber still belongs to typical cellulose I. Furthermore, the presence of water molecules in the amorphous region of cellulose, combined with the three hydroxyl groups of each glucose unit, did not undergo any significant changes due to the low-temperature freezing.
Figure 4. XRD results of cotton fibers under various moisture regain and freezing day: (a) XRD diffraction pattern. (b) the trend of crystallinity with freezing day.

Since cotton was natural cellulose fibers, the empirical XRD crystallinity index can be calculated using the peak intensity ratio method (Thygesen et al. Citation2005), to characterize the change and study in crystallinity, the method proposed by Segal (Segal et al. Citation1959), allows for quick determination of fiber crystallinity and is given by EquationEquation 3(3)
(3) .
where I002 represents the intensity of the 002 crystalline surface diffraction peak and Iam represents the diffraction intensity of the amorphous component at 2θ = 18°.
Combined with EquationEquation 3(3)
(3) , the results can be obtained from , and the range of variation in the crystallinity of the pre-treated fibers was not significant. The crystallinity of the cotton fiber tends to decrease as the number of freezing days increases, reaching the lowest value of 64.39% on the 8 days of freezing, and the crystalline were 2.07% less than the control sample. Additionally, this supports the impact of the pre-treatment on the composition and fracture strength of the cotton fiber (Liang et al. Citation2007). As the number of freezing days increases, the microstructure of the cotton fibers is compromised, due to the increased in ice-crystals. Moreover, when the freezing days reach 8, the ice-crystal particles press against the hollow structure, bringing the arrangement of its macromolecules closer.
In order to understand the cellulose, 13C NMR was used to analyze the shift changes of the wave peaks in the cellulose13C NMR (Svanson Citation1978; R. E. Taylor et al. Citation2008). The13C NMR results were shown in , which were consistent with the13C NMR curves of the cotton fibers from the control sample and the sample treated with 75% freezing for 8 days, and no new distinctive peaks emerged. The pre-treatment cotton fibers produced the following cellulose resonances: The C-1 peak varied from 102 ppm to 108 ppm, while the C-4 peak contained two resonance peaks at 89 ppm and 84 ppm, respectively. These resonance peaks indicated the presence of cellulose Iα in the crystalline structure of the cotton fibers. Additionally, the resonance peaks of C-2, 3, and 5 overlapped and appeared as double peaks near 75 ppm and 72 ppm, respectively, preventing the use for the analysis of the crystalline structure. These resonance peaks of C-2, 3, and 5 were attributed to the cyclic carbon not linked to the glycosidic bond. Finally, the primary alcohol C-6 were shown two peaks, one larger at 65 ppm and the other at 63 ppm. The links in the cellulose chain were provided by C-1 and C-4, and the smaller peaks for C-4 and C-6 suggest a “less organized environment” in the cellulose chain (L. Zhang et al. Citation2007).
Figure 5. 13C NMR results of cotton fiber under various moisture regain and freezing day:(a) 13C NMR results. (b) results of fitting the C-1 signal peak of the cotton fiber13C NMR under typical circumstances. (c) results of fitting the C-1 signal peak of the cotton fibers13C NMR, which was frozen for 8 days in 75% condition. (d) results of fitting the C-4 signal peak of the cotton fiber13C NMR under typical circumstances. (e) results of the fitting of the13C NMR C-4 signal peak.

In addition, the13C NMR C-1 peaks of cotton fibers subjected to pretreatment were fitted using Lorentzian linear to represent the various crystalline types (see ) (H. S. Taylor, Haiges, and Kershaw Citation2013). It could be observed the crystalline forms were predominantly cellulose Iβ, with smaller amounts of cellulose Iα, cellulose II, and secondary paracrystalline (Atalla and VanderHart Citation1999). The experimental findings, presented in , demonstrate the composition of the crystalline structure by pre-treatment was modified. Compared to the control sample, the content of cellulose II increased by 31.31% and the content of paracrystalline increased by 0.11%. However, the percentage of cellulose Iβ and cellulose Iα in the treated cotton fiber samples decreased. This may be due to the cellulose II fibers have a more stable structure than cellulose I fibers (Yue Citation2011).
Table 1. Comparison of the percentage of crystal types and the XRD and NMR crystallinity index.
Furthermore, the C-4 peak (shown in ) was fitted using Lorentzian (H. S. Taylor, Haiges, and Kershaw Citation2013). It consists of two peaks: a sharp resonance peak and a broader side peak, indicating the crystalline and non-crystalline phases of the fiber. The measurement of fiber crystallinity using the13C NMR method was more accurate than XRD method. In comparison to X-ray diffraction, 13C NMR was more sensitive to the order in a small range. demonstrates that cotton fibers processed with a moisture regain of 75% for 8 days had slightly higher crystallinity than the control sample, which was consistent with the trend of fiber crystallinity as determined by XRD.
Morphology of the cotton fiber
Herein, cotton fiber samples were manufactured and characterized by a scanning electron microscope (SEM). It was observed that various moisture recovery methods, such as low-temperature freezing and rewarming, caused microscopic damage to the surface of the cotton fibers. illustrates the changes in experimental samples, with the x-axis and y-axis represented different factors. The control sample, which had normal moisture regain, showed a relatively smooth surface without obvious damage. Among the morphology samples, the most representative one was the 75% pre-treatment sample, which exhibited fractures (e.g., 5 days, 8.5%), localized holes (e.g., 3 days, 8 days, 50%), fold-like damaged (e.g., 1 day, 75%), and enhanced cracking (e.g., 8 days, 75%; 3 days, 25%). These damages tend to weaken the fiber rings and increase the likelihood of fracture during processing. Further, the arrows in the indicate the presence of increased striations on the fiber surface, as the moisture regain decreased, the banded-waist rounded morphology of the cotton fibers became wet and flattened, reducing the number of natural turns. Additionally, the results showed that fiber surface damage increased with decreasing moisture regain, and the severity of fiber surface damage increased with the length of cryogenic freezing treatment (Z. S. Zhang and Liu Citation2018). Fibers with greater cracks, perforations, and folds had worse mechanical qualities and may crack during subsequent ginning processes (Sarna and Włochowicz Citation2003).
Mechanical properties of the cotton fiber
The fracture strength and elongation at the break of cotton fibers were known to be affected by rapidly changing moisture regains (Lawson, Ramey, and Krowicki Citation1976). Therefore, it was important to examine the mechanical behavior of cotton fibers to accurately assess the level of damage to their mechanical characteristics in severe conditions. presents comparison graphs of the fracture strength and elongation at break curves from tensile experimental data by using the LLY-06E machine. were shown that the fracture strength and elongation at break tended to decrease, reaching minimum values of 388.71 MPa and 7.7%, respectively, after 3 and 8 days of treatment. This indicates that short-term pre-treatment can reduce the mechanical properties of the fibers. Compared to the control sample at 8 days, the fracture strength decrease was 4.32%, but the largest decrease occurred after 3 days, reaching 4.62%. The reduction in fracture strength and elongation at break may be attributed to the increase in the volume of ice-crystals (Kalita et al. Citation2023), which leads to the broken of fibrils and the occurrence of a “weak-link phenomenon” within the cotton fibers (Ye and Weidong Citation2006). This broken alters the friction properties of the fibers and decreases the original degree of orientation when compressed by the ice-crystals. The initial modulus of the cotton fibers was also examined (). It was shown a trend of initially decreasing, then increasing, and finally decreasing with the freezing day. The most significant decrease, reaching 948.32 MPa, was observed after 1-day freezing. After 8 days freezing, the initial modulus reached as low as 4180.17 MPa, which was 29.76% lower than control sample. These findings confirm cryogenic freezing affects the physical and mechanical characteristics of cotton fibers, as determined by SEM examination.
Fracture damage mechanism
According to the hygroscopic theory of fiber analysis, the water molecules in the air adsorb on the unbound molecular bonds in the cellulose macromolecule through the role of β-1,4-glucosidic bonds. This absorption leads to cross-linking, improving the degree of tightness of molecular aggregation and allowing the fibers to withstand external factors more evenly. The phenomenon was likely due to the hygroscopic properties of cotton fiber (Singh et al. Citation1969). illustrates the fracture damage mechanism of cotton fiber in a moisture conditioning and low-temperature environment. When the moisture regain reaches 75%, pre-treatment causes ice-crystals damage to the fibrils. This damage was likely a result of increased ice-crystals particles that extrusion the fibrils, and damage the fibrils. Moreover, the presence of a “freezing bound” component, which was a weakly-bound water population found in clusters or associated with voids, decreases the size of cavities in the center of cotton fibers. This component may result in a more stable, orderly, and increased orientation of the macromolecular chains of the fibers following the rewarming treatment. Similar to the findings of Meredith (Meredith Citation1957), at saturation regain, one molecule of water was bonded to each accessible polar group. These polar groups would otherwise be connected to similar polar groups in nearby chain molecules through hydrogen bonds. The trends in mechanical properties explained by the formation of amorphous areas of ice-crystals during freezing, which alters the intermolecular distances inside cotton fibers. Additionally, the crystal growth of ice-crystals in supercooled water (Z. S. Zhang and Liu Citation2018) leads to a more stable and regular arrangement of fiber macromolecular chains, increasing the crystallinity of the fibers.
Conclusion
Different qualities of cotton fibers were obtained from the treatment of moisture conditioning- low-temperature- rewarming, we studied the changes in the physical properties and structure of cotton fibers. The linear density of cotton fibers also exhibits an upward and downward pattern, reaching its peak. Furthermore, cotton fiber crystalline structure barely changed, while the macromolecular structure of cellulose fibers mostly remained unchanged, and the chemical composition of cotton fibers was unaffected by the moisture conditioning and low temperature freezing processes. However, in the low-temperature freezing environment, cracks, localized holes, and fold-like damage on the fiber surface become more obvious. The banded-waist rounded morphology of cotton fibers also changes as they become saturated, flatten out, and lose some of their turning curvature. Additionally, the mechanical characteristics of cotton fibers in this work deteriorated when subjected to a brief period of cryogenic freezing treatment, therefore, the fracture damage mechanism given the reason. We envision that, by studying cotton fibers with different moisture regain and low temperature environments, this article focuses on the damage of extreme environments to the original quality of cotton fibers, taking us considerable step closer to sustainable cotton fiber harvest and ginning process technology.
Highlights
A new pretreatment strategy, i.e., moisture regains and low-temperature, was designed for study the property changes of cotton fibers. The different types of cotton fiber samples were obtained by the treatment of moisture conditioning-low temperature-rewarming. Comparing the control sample and the pretreatment fiber that had more obvious cracks, local holes and folded damage with increase of low-temperature freezing days.
Synergistic effects of high moisture regain and low-temperature on the fibrils structure and crystallinity, and bonding energy of cotton fibers. The apparent structure, mechanical properties and macromolecular structure of the cotton fiber test samples were characterized and analyzed using IR, XRD, XPS and 13C NMR, etc. It was further verified that the internal molecules may have been changed or reorganized during the treatment of moisture conditioning- low-temperature- rewarming, and the stability of the internal aggregated state structure of the fibers decreases, exhibiting a decrease in the mechanical properties of the fibers.
Ice-crystal damage to the fibrils causes mechanical property reduction. The results of mechanical indicated that, the mechanical properties of cotton fibers decreased rapidly when subjected to low-temperature freezing treatment, with a minimum value of 388.71 MPa and 7.7%, respectively, when the treatment days were 3 and 8.
Author’s contributions
Jiqiang Cao: Methodology, Software, Testing, Data curation, Writing-Original draft preparation. Xiang Liu: Software, Supervision, Validation, Writing- Reviewing. Ruolian Mo: Visualization, Investigation Testing. Hongling Liu: Thesis structure construction, Data handling, sample analysis. Hong Xu: Conceptualization, Writing- Reviewing and Editing, Funding acquisition.
Consent to publish
I confirm that any participants (or their guardians if unable to give informed consent, or next of kin, if deceased) who may be identifiable through the manuscript (such as a case report), have been given an opportunity to review the final manuscript and have provided written consent to publish.
Ethical approval
I confirm that all the research meets ethical guidelines and adheres to the legal requirements of the study country.
Acknowledgments
We gratefully acknowledgements the financial support of the National Natural Science Foundation of China, the authors also thank College of Textile and Fashion, and the Ginning Company providing cotton sample, field investigation, and fiber testing.
Disclosure statement
No potential conflict of interest was reported by the author(s).
Additional information
Funding
References
- Abidi, N., L. Cabrales, and C. H. Haigler. 2014. “Changes in the Cell Wall and Cellulose Content of Developing Cotton Fibers Investigated by FTIR Spectroscopy.” Carbohydrate Polymers 100:9–15. https://doi.org/10.1016/j.carbpol.2013.01.074.
- Agoda-Tandjawa, G., S. Durand, S. Berot, C. Blassel, C. Gaillard, C. Garnier, and J. L. Doublier. 2010. “Rheological Characterization of Microfibrillated Cellulose Suspensions After Freezing.” Carbohydrate Polymers 80 (3): 677–686. https://doi.org/10.1016/j.carbpol.2009.11.045.
- Anthony, W. S. 1994. Cotton Ginners Handbook. Washington, DC: Department of Agriculture Agricultural Research Service.
- Atalie, D., G. Ashagre, E. Ferede, F. Getnet, and A. Nibret. 2022. “Investigation of Ethiopian Cotton Fiber Stickiness. Part 1 – Northern Part.” Journal of Natural Fibers 19 (15): 12026–12038. https://doi.org/10.1080/15440478.2022.2049420.
- Atalla, R. H. 1978. “Conformation Effects in the Hydrolysis of Cellulose.” Advances in Chemistry 9:1–23. https://doi.org/10.1021/ba-1979-0181.ch003.
- Atalla, R. H., and S. C. Nagel. 1974. “Cellulose: Its Regeneration in the Native Lattice.” Science 185 (4150): 522–523.
- Atalla, R. H., and D. L. VanderHart. 1999. “The Role of Solid State C-13 NMR Spectroscopy in Studies of the Nature of Native Celluloses.” Solid State Nuclear Magnetic Resonance 15 (1): 1–19. https://doi.org/10.1016/s0926-2040(99)00042-9.
- Barker, G. L., J. Weldon Laird, M. G. Pelletier, and G. A. Holt. 2001. “Relative Velocity, Density, and Temperature Effects on Cotton Moisture Transfer Rates.” Journal of Cotton Science 5:243–251.
- Cao, J., L. Cheng, C. Zhang, S. Yuan, and H. Xu. 2021. “Current Status and Countermeasures of Machine-Picked Cotton Primary Processing Research.” Cotton Textile Technology 49 (2): 75–79.
- Cao, J., and H. Xu. 2022. “Development Status and Quality Improvement Countermeasure of Xinjiang Cotton.” Cotton Textile Technology 50 (6): 71–74.
- Cevheri, C. I. 2022. “The Effect of Rain on Fiber and Yarn Quality of Cotton (G. Hirsutum L.) Varieties in Two Different Growing Seasons.” Journal of Natural Fibers 19 (9): 3558–3570. https://doi.org/10.1080/15440478.2021.2009402.
- Cintrón, M. S., and B. F. Ingber. 2013. “Preliminary Examination of the Effects of Relative Humidity on the Fracture Morphology of Cotton Flat Bundles.” Textile Research Journal 83 (10): 1044–1054. https://doi.org/10.1177/0040517512470194.
- Gipson, J. R., and H. E. Joham. 1968. “Influence of Night Temperature on Growth and Development of Cotton (Gossypium Birsutum L.). II Fiber Properties 1.” Agronomy journal 60 (3): 296–298. https://doi.org/10.2134/agronj1968.00021962006000030015x.
- Hart, W. J., T. L. W. Bailey, W. R. Keyser, and J. Compton. 1955. “The Effect of an Excessive Gin-Drying Temperature of Cotton on Yarn Quality and Mill Processing.” Textile Research Journal 25 (5): 415–421. https://doi.org/10.1177/004051755502500508.
- Hindeleh, A. M., D. J. Johnson, and P. E. Montague. 1980. “Computational Methods for Profile Resolution and Crystallite Size Evaluation in Fibrous Polymers.” In Fiber Diffraction Methods, edited by A. D. French and K. H. Gardner, 149–182. US: ACS Symposium: ACS Publications.
- Igarashi, T., M. Hoshi, K. Nakamura, T. Kaharu, and K.-I. Murata. 2020. “Direct Observation of Bound Water on Cotton Surfaces by Atomic Force Microscopy and Atomic Force Microscopy-Infrared Spectroscopy.” The Journal of Physical Chemistry C 124 (7): 4196–4201. https://doi.org/10.1021/acs.jpcc.0c00423.
- Johansson, L. S., and J. M. Campbell. 2004. “Reproducible XPS on biopolymers: cellulose studies.” Surface and Interface Analysis 36 (8): 1018–1022. https://doi.org/10.1002/sia.1827.
- Johansson, L. S., J. Campbell, K. Koljonen, M. Kleen, and J. Buchert. 2004. “On surface distributions in natural cellulosic fibres.” Surface and Interface Analysis 36 (8): 706–710. https://doi.org/10.1002/sia.1741.
- Kalita, A., M. Mrozek-McCourt, T. F. Kaldawi, P. R. Willmott, N. Duane Loh, S. Marte, R. G. Sierra, et al. 2023. “Microstructure and Crystal Order During Freezing of Supercooled Water Drops.” Nature 620 (7974): 557–561. https://doi.org/10.1038/s41586-023-06283-2.
- Lawson, R., H. H. Ramey, and R. S. Krowicki. 1976. “Cotton Fiber Tenacity and Elongation in Rapidly Changing Relative Humidity.” Textile Research Journal 46 (10): 715–719. https://doi.org/10.1177/004051757604601004.
- Leitgeb, D. J., and H. Wakeham. 1954. “Cotton Quality and Fiber Properties: Part III: Effects of Drying at High Temperatures Prior to Ginning.” Textile Research Journal 24 (12): 1047–1057. https://doi.org/10.1177/004051755402401203.
- Liang, S., L. Zhang, Y. Li, and J. Xu. 2007. “Fabrication and Properties of Cellulose Hydrated Membrane with Unique Structure.” Macromolecular Chemistry and Physics 208 (6): 594–602.
- Liu, H., T. Gu, W. Yu, Y. Xing, and J. Zhou. 2018. “Observation of Luminescent Gold Nanoclusters Using One-Step Syntheses from Wool Keratin and Silk Fibroin Effect.” European Polymer Journal 99:1–8. https://doi.org/10.1016/j.eurpolymj.2017.11.036.
- Martin, L. K., and C. H. Haigler. 2004. “Cool Temperature Hinders Flux from Glucose to Sucrose During Cellulose Synthesis in Secondary Wall Stage Cotton Fibers.” Cellulose 11 (3): 339–349. https://doi.org/10.1023/b:cell.0000046420.10403.15.
- Meredith, R. 1957. “14—rigidity, moisture and fibre structure.” Journal of the Textile Institute Transactions 48 (6): T163–T74.
- Nakamura, K., T. Hatakeyama, and H. Hatakeyama. 1981. “Studies on Bound Water of Cellulose by Differential Scanning Calorimetry.” Textile Research Journal 51 (9): 607–613. https://doi.org/10.1177/004051758105100909.
- Patil, P. G., P. Madhukar Padole, and J. Fulchand Agrawal. 2007. “Effect of Roller Speed and Moisture Content of Cotton on Ginning Rate, Lint Quality and Electric Energy Consumption in Double Roller Gins.” Textile Research Journal 77 (9): 635–644. https://doi.org/10.1177/0040517507078039.
- Peirce, F. T. 1929. “16—A Two-Phase Theory of the Absorption of Water Vapour by Cotton Cellulose.” Journal of the Textile Institute Transactions 20 (6): T133–T50.
- Powell-Palm, M. J., B. Rubinsky, and W. Sun. 2020. “Freezing Water at Constant Volume and Under Confinement.” Communications Physics 3 (1): 39. https://doi.org/10.1038/s42005-020-0303-9.
- Rousselle, M.-A., D. P. Thibodeaux, and A. D. French. 2005. “Cotton Fiber Properties and Moisture: Water of Imbibition.” Textile Research Journal 75 (2): 177–180. https://doi.org/10.1177/004051750507500217.
- Sarna, E., and A. Włochowicz. 2003. “Changes in the Superstructure and Surface Morphology of Cotton During Spinning.” Textile Research Journal 73 (3): 216–220. https://doi.org/10.1177/004051750307300305.
- Segal, L. G. J. M. A., J. J. Creely, A. E. Martin Jr, and C. M. Conrad. 1959. “An Empirical Method for Estimating the Degree of Crystallinity of Native Cellulose Using the X-Ray Diffractometer.” Textile Research Journal 29 (10): 786–794.
- Shizuo, K., and K. EMORI. 1993. “Modification of Cotton Fiber by Low Temperature Plasma.” Journal of Photopolymer Science and Technology 6 (3): 353–360.
- Singh, B., C. L. Jain, A. Pande, and V. B. Chipalkatti. 1969. “Moisture Sorption of Cross-Linked Cotton Cellulose in Relation to Swelling.” Textile Research Journal 39 (12): 1117–1125. https://doi.org/10.1177/004051756903901207.
- Svanson, S. E. 1978. “Changes in NMR Characteristics of Cotton and Wool During Water Adsorption.” Journal of Applied Polymer Science 22 (2): 571–576.
- Taylor, R. E., A. D. French, G. R. Gamble, D. S. Himmelsbach, R. D. Stipanovic, D. P. Thibodeaux, P. J. Wakelyn, and C. Dybowski. 2008. “H-1 and C-13 Solid-State NMR of Gossypium Barbadense (Pima) Cotton.” Journal of Molecular Structure 878 (1–3): 177–184. https://doi.org/10.1016/j.molstruc.2007.08.006.
- Taylor, H. S., R. Haiges, and A. Kershaw. 2013. “Increasing Sensitivity in Determining Chemical Shifts in One Dimensional Lorentzian NMR Spectra.” The Journal of Physical Chemistry A 117 (16): 3319–3331. https://doi.org/10.1021/jp310725k.
- Tesema, G. B., and A. Drieling. 2020. “Effect of Ginning on Tenacity, Elongation and Other Fibre Quality Properties.” The Journal of the Textile Institute 111 (3): 326–333. https://doi.org/10.1080/00405000.2019.1638214.
- Thygesen, A., J. Oddershede, H. Lilholt, A. B. Thomsen, and K. Stahl. 2005. “On the Determination of Crystallinity and Cellulose Content in Plant Fibres.” Cellulose 12 (6): 563–576. https://doi.org/10.1007/s10570-005-9001-8.
- Ye, L., and Y.U. Weidong. 2006. “Characterization of Weak-Link Rate of High-Performance Fibres Based on the Distributions of Single-Fibre Tensile Properties.” Journal of Donghua University (Natural Science Edition) 1:100–104.
- Yu, W. 2018. Textile Materials Science. US: China Textile Press.
- Yue, Y. 2011. A Comparative Study of Cellulose I and II and Fibers and Nanocrystals. Louisiana, USA: Louisiana State University.
- Zelinka, S. L., M. J. Lambrecht, S. V. Glass, A. C. Wiedenhoeft, and D. J. Yelle. 2012. “Examination of Water Phase Transitions in Loblolly Pine and Cell Wall Components by Differential Scanning Calorimetry.” Thermochimica acta 533:39–45. https://doi.org/10.1016/j.tca.2012.01.015.
- Zhang, Z. S., and X. Y. Liu. 2018. “Control of Ice Nucleation: Freezing and Antifreeze Strategies.” Chemical Society Reviews 47 (18): 7116–7139. https://doi.org/10.1039/c8cs00626a.
- Zhang, L., J. Ye, S. Wang, and P. Chem Ind. 2007. Studies Upon the Structure and Properties Oflong-Staple Cotton Fiber Under Microwave Radiation. Paper presented at the International Conference on Advanced Fibers and Polymer Materials, Shanghai, Peoples R China, 2007 October 15-17.
- Zhao, H. B., J. H. Kwak, Z. C. Zhang, H. M. Brown, B. W. Arey, and J. E. Holladay. 2007. “Studying Cellulose Fiber Structure by SEM, XRD, NMR and Acid Hydrolysis.” Carbohydrate polymers 68 (2): 235–241. https://doi.org/10.1016/j.carbpol.2006.12.013.