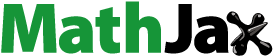
ABSTRACT
Viruses remain a global threat to animals, plants, and humans. The type 1 human immunodeficiency virus (HIV-1) is a member of the retrovirus family and carries an RNA genome, which is reverse transcribed into viral DNA and further integrated into the host-cell DNA for viral replication and proliferation. The RNA structures from the HIV-1 genome provide valuable insights into the mechanisms underlying the viral replication cycle. Moreover, these structures serve as models for designing novel therapeutic approaches. Here, we review structural data on RNA from the HIV-1 genome as well as computational studies based on these structural data. The review is organized according to the type of structured RNA element which contributes to different steps in the viral replication cycle. This is followed by an overview of the HIV-1 transactivation response element (TAR) RNA as a model system for understanding dynamics and interactions in the viral RNA systems. The review concludes with a description of computational studies, highlighting the impact of biomolecular simulations in elucidating the mechanistic details of various steps in the HIV-1’s replication cycle.
Overview: HIV-1 replication cycle
Viruses are infectious agents which hijack the host-cell’s biological apparatus for the synthesis of the viral RNA and proteins necessary for viral replication [Citation1–10]. Since viruses are widespread and rapidly develop resistance to vaccines and other drug therapies [Citation11], continued efforts are necessary to combat them [Citation12,Citation13]. The Baltimore classification system [Citation1,Citation14] categorizes viruses into seven classes based on their viral genomes. Among them, retroviruses (Retroviridae) contain genomes with a positive sense single-stranded RNA with DNA intermediates (ssRNA-RT) [Citation15]. After the identification of the first retroviral disease in chickens [Citation16], it was discovered that retroviruses could produce a DNA copy of the viral RNA genome through the reverse transcription process [Citation17,Citation18] and integrate it into the host-cell chromosomal DNA [Citation16,Citation19]. Later, the acquired immunodeficiency syndrome (AIDS) disease was linked to the human immunodeficiency virus type 1 (HIV-1) [Citation20,Citation21], one of the deadliest viruses that is still non-curative [Citation22].
The HIV-1 virion has a spherical shape with a diameter of 120 nm (top right; ). The virion contains two copies of a single-stranded long positive-sense RNA genome, each of which consists of
10,000 nucleotides [Citation23]. These two copies of the HIV-1 RNA genome, along with the viral replication enzymes, namely the reverse transcriptase (RT), integrase (IN), and nucleocapsid (NC) protein, are encapsulated in the conically-shaped mature capsid (CA) core (top right; ) [Citation24]. The HIV-1 replication cycle consists of a series of biological events which can be broadly divided into early and late steps () [Citation25]. The key early steps include binding to the cell membrane, cell entry (fusion), reverse transcription, nucleus import, and the integration of the viral genome into the host-cell genome () [Citation26]. Likewise, the late steps include the transcription step for producing the viral gRNA and the viral messenger RNAs (mRNAs), nuclear export of the newly transcribed RNAs, translation, dimerization and packaging of the gRNA strands, assembly of the new virus particle on the cell membrane, and finally the release of the virus particle for further maturation () [Citation23,Citation25].
Figure 1. A schematic overview of the HIV-1 replication cycle. The entry of HIV-1 begins with the binding of the HIV-1 virion to the cell membrane through the interactions of the envelope-glycoproteins (Env) with the cell membrane receptors (step 1). This is followed by the fusion of the virion with the cell membrane and entry of the viral particle into the cell (step 2). While the gRNA strands are reverse transcribed into vDNA, the viral core gets transported to the cell nucleus (steps 3–4). vDNA generated through the reverse transcription process is further integrated into the host-cell chromosome (step 5). Upon completion of the integration step, proviral DNA gets transcribed into vRNA molecules which are exported into the cytoplasm (steps 6–7). The vRNA molecules are then either translated into viral proteins or assembled for further packaging with newly synthesized protein molecules (steps 8–9). The assembled viral particle buds on the cell membrane using newly synthesized viral proteins and gRNA and is further released from the cell membrane upon completion of the assembly step (steps 10–11). The release is followed by the maturation process to produce an infectious viral particle (step 12).

The binding process is mediated by the interactions between the HIV-1 envelope-glycoprotein (Env) spikes and the cell-membrane receptors (step 1; ). These interactions induce structural rearrangements in the HIV-1 virion resulting in the fusion of viral and host-cell membranes (step 2; ) [Citation25]. Upon completion of the fusion step, the conically-shaped HIV-1 core is released into the cell cytoplasm (step 2; ). While the HIV-1 core is transported to the cell nucleus, the reverse transcription process is performed by the RT enzyme which converts viral RNA (vRNA) into linear double-stranded viral DNA (vDNA) (step 3; ). During reverse transcription, a pre-packaged tRNA molecule () anneals to the primer binding site (PBS) RNA located in the
-untranslated region (UTR) of the HIV-1 genome [Citation27]. After that, the annealed double-stranded RNA (dsRNA) binds the nucleic acid-binding cleft of RT to proceed with the next step of the reverse transcription process. After being transported to the cell nucleus, the HIV-1 genetic material is imported into the cell nucleus (step 4; ). Inside the cell nucleus, the reverse transcribed vDNA is integrated into the host-cell DNA genome by the HIV-1 IN enzyme (step 5; ) [Citation28]. To complete the integration step, the HIV-1 capsid dissociates via the uncoating process to release the reverse transcribed vDNA. However, the precise timing of the uncoating process occurring in the cell cytoplasm or in the nucleus, as well as the mechanism of uncoating, are still under debate [Citation29].
The HIV-1 vDNA integration signifies the transition from the early stage to the late stage in the HIV-1 replication cycle. Transcription of the integrated vDNA, commonly referred to as a provirus, results in the synthesis of viral gRNA and mRNA molecules (step 6; ). The transcription process is modulated by the binding of the transactivator of transcription (Tat) protein to the conserved TAR RNA hairpin at the -end of the newly synthesized RNA transcripts. After transcription is completed, the newly synthesized mRNA and gRNA molecules are exported into the cell cytoplasm (step 7; ). This process is mediated by the binding of the regulator of expression of virion (Rev) protein to the conserved Rev response element (RRE) RNA present in mRNAs. Next, HIV-1 mRNA molecules are translated using the host-cell ribosomes into a group of viral enzymes (step 8; ). Specifically, structural proteins matrix (MA), CA, and NC are synthesized as part of the precursor polyprotein group-specific antigen (Gag) chain. Additionally, several enzymes (e.g. Tat, Rev) are synthesized and imported back into the cell nucleus to further promote the transcription and the nuclear export steps (step 8; ). The Gag molecules mediate all processes during the assembly step of the new virus particle formed on the cell membrane with the dimerized gRNA strands (step 9; ) [Citation30]. The gRNA dimerization process is initiated by the formation of the kissing-loop complex between the dimerization initiation site (DIS) motifs located in SL1 RNA of two gRNA strands. During the assembly process, the virus particle begins to bud on the cell membrane forming a spherically-shaped particle with packaged gRNA (step 10; ). The immature (non-infectious) HIV-1 virion is then released from the cell membrane (step 11; ). This step is followed by the cleavage of the Gag polyprotein into MA, CA, and NC proteins which leads to structural rearrangements inside the HIV-1 virion. As a result, a mature (infectious) HIV-1 virion is produced via the maturation process (step 12; ).
The entire HIV-1 RNA genome has been characterized at a single-nucleotide resolution using selective -hydroxyl acylation analysed by primer extension (SHAPE) technique [Citation12,Citation31]. The entire genome has also been characterized using dimethyl sulphate mutational profiling with sequencing (DMS-MaPseq) [Citation32,Citation33]. In the HIV-1 genome, a variety of structured RNA elements have established effects on the viral replication process, including reverse transcription, protein synthesis, nuclear export, and dimerization processes [Citation2–4,Citation8,Citation9,Citation34–37]. The molecular organization of RNA is best understood by determining its structure using experimental tools such as nuclear magnetic resonance (NMR) spectroscopy [Citation38,Citation39], X-ray crystallography [Citation40], and cryo-electron microscopy (cryo-EM) [Citation41]. Here, we review the roles and functions of the HIV-1 RNA elements which participate in the viral replication cycle, including PBS RNA, TAR RNA, RRE RNA, and Psi RNA. While no structural studies are known on the HIV-1 RNA elements participating in the translation process, we highlight the progress made via biochemical studies. We also review the usefulness of HIV-1 TAR RNA as a model system for characterizing dynamics and interactions in RNA. We conclude by summarizing computational studies on RNA molecules from HIV-1 and providing a brief perspective.
Structural and biochemical studies on HIV-1 PBS RNA
Brief overview of PBS RNA and its role in reverse transcription
The reverse transcription process is one of the key early steps in the HIV-1 replication cycle. In this step, two copies of the single-stranded positive-sense gRNA are reverse transcribed into DNA (step 3; ). Reverse transcription is initiated upon annealing of a human primer to the complementary conserved PBS RNA located in the
-UTR of the HIV-1 genome [Citation27]. This process is proposed to consist of multiple intermediate steps which are briefly described below and a detailed overview of reverse transcription is provided elsewhere [Citation25,Citation42]. The human
is selectively prepackaged in the HIV-1 virion [Citation43]. Prior to annealing to the PBS RNA, the primer
is recognized by the copackaged human lysyl-tRNA synthetase (hLysRS) protein [Citation44,Citation45]. The hLysRS protein further targets the U-rich loop (URL) motif of the tRNA-like element (TLE) in the PBS-segment RNA [Citation43].
Binding of the hLysRS protein to the URL motif promotes recognition of the primer by the A-rich loop (ARL) motif located in the TLE [Citation46]. The PBS RNA consists of 18 nucleotides which bind the complementary
-end of the primer
to form an 18-base pair intermolecular RNA helix. The annealed double-stranded RNA (dsRNA) is a key element of the reverse transcription initiation complex (RTIC), which also includes the URL and the ARL motifs in TLE, and the primer activation site (PAS). The secondary structures of the HIV-1 PBS RNA and the
primer are shown in . The annealed 18-nucleotides long dsRNA then binds the nucleic acid-binding cleft of RT to engage in the synthesis of the minus-strand DNA from the
-end of the
primer ().
Figure 2. The initiation complex of the HIV-1 reverse transcription. Shown are (A) the secondary structures of the PBS-segment RNA and the primer with various structural motifs uniquely colored and labeled; (B) the side-view snapshot of the PBS-segment RNA with key structural motifs uniquely colored and labeled following the color scheme defined in panel A (PDB 7LVA) [Citation47]; and (C) the side-view snapshot of the ARL anticodon of the
(pink) annealed to the ARL (blue) of the HIV-1 TLE (light blue; PDB 2K7E) [Citation46]. The modifications in the ARL anticodon motif are highlighted in red. The snapshots in panels B and C utilize the first frame of the corresponding NMR structure. (D) The side-view snapshot of the HIV-1 reverse transcription initiation complex (PDB 6HAK) [Citation48] is shown with two RT domains, the p66 and p51 domains, highlighted in light blue and pink, respectively. The dsRNA structure is colored following the color scheme in panel A. (E) Two conformations of the
end of the anti-PBS segment of the
primer annealed to PBS at the RTIC are shown along with the vDNA strand from the elongation complex at the P site (PDBs 6B19, 6HAK, 5TXL) [Citation48–50]. The primer conformations correspond to superimposed functional states of the RTIC on the elongation complex with vDNA: state 1 (dark orange;
+1) and state 2 (light orange;
) and the elongation complex (white; P).
![Figure 2. The initiation complex of the HIV-1 reverse transcription. Shown are (A) the secondary structures of the PBS-segment RNA and the tRNA3Lys primer with various structural motifs uniquely colored and labeled; (B) the side-view snapshot of the PBS-segment RNA with key structural motifs uniquely colored and labeled following the color scheme defined in panel A (PDB 7LVA) [Citation47]; and (C) the side-view snapshot of the ARL anticodon of the tRNA3Lys (pink) annealed to the ARL (blue) of the HIV-1 TLE (light blue; PDB 2K7E) [Citation46]. The modifications in the ARL anticodon motif are highlighted in red. The snapshots in panels B and C utilize the first frame of the corresponding NMR structure. (D) The side-view snapshot of the HIV-1 reverse transcription initiation complex (PDB 6HAK) [Citation48] is shown with two RT domains, the p66 and p51 domains, highlighted in light blue and pink, respectively. The dsRNA structure is colored following the color scheme in panel A. (E) Two conformations of the 3′ end of the anti-PBS segment of the tRNA3Lys primer annealed to PBS at the RTIC are shown along with the vDNA strand from the elongation complex at the P site (PDBs 6B19, 6HAK, 5TXL) [Citation48–50]. The primer conformations correspond to superimposed functional states of the RTIC on the elongation complex with vDNA: state 1 (dark orange; P′+1) and state 2 (light orange; P′) and the elongation complex (white; P).](/cms/asset/f4f7df7e-1e27-4a4b-9a04-63adc4130f72/krnb_a_2289709_f0002_oc.jpg)
In this section, we highlight the impact of structural studies of the HIV-1 RNA participating in the reverse transcription process. Between 2008 and 2023, a number of RNA structures which participate in the reverse transcription process have been resolved, providing valuable insights on this process (). However, the majority of these structures are focused on the RTIC and the interactions between the viral PBS RNA and the RT enzyme [Citation48,Citation49,Citation53–55]. Therefore, we still lack a detailed understanding of the mechanism of recognition of the full PBS-segment RNA by the hLysRS protein and the primer during the first step of reverse transcription. Furthermore, the role of post-transcriptional modifications during reverse transcription is not fully understood. Specifically, we still lack complete structural details on the effect of various post-transcriptional modifications on the stability of the PBS/
RNA complex. Moreover, the role of post-transcriptional modifications in regulation of the reverse transcription process is also poorly understood [Citation56,Citation57].
Table 1. Structural data on the HIV-1 RNA elements participating in reverse transcription. For each structure, shown is the HIV-1 RNA identifier, the corresponding pdb, the binding partner/ligand, the experimental method used to resolve the structure, and the publication year. The following abbreviations are used: ARL, A-rich loop; cryo-EM, cryo-electron microscopy; NCp7, nucleocapsid protein 7; NMR, nuclear magnetic resonance; PBS, primer binding site; RT, reverse transcriptase; TAR, transactivation response element. Furthermore, RNA/DNA is used to indicate the RNA/DNA hybrid.
Studies on the initiation complex between PBS RNA and tRNA3Lys primer
Reverse transcription of HIV-1 gRNA is initiated when the primer anneals to the PBS-segment RNA consisting of several flexible stem-loops [Citation31,Citation58,Citation59]. However, complete structural features of the PBS-segment RNA were not resolved until 2021 when a solution structure of the complete HIV-1 PBS-segment RNA, prior to
binding, was reported [Citation47] (). Based on the NMR structure, it was proposed that the three-way junction initially formed at the adenosine-rich segment in the PAS stem (inset; ) and then folded into two stem-loops, the tRNA annealing arm and the TLE containing flexible URL and ARL motifs (). Infectivity assays and mutational analysis further showed that the disruption of the three-way junction configuration led to reduced viral infectivity, thereby highlighting the importance of the PBS-segment RNA in reverse transcription [Citation47].
Next, a number of interactions are formed between the primer and the HIV-1 gRNA, resulting in a highly structured initiation complex. Specifically, based on data from experiments involving NMR and single-molecule fluorescence spectroscopy, it was proposed that the PBS-segment RNA in complex with the primer tRNA could adopt two distinct conformations in vitro [Citation60]. One of these conformations was proposed to contain an interaction between the PAS RNA and the tRNA primer [Citation60]. This interaction could destabilize the gRNA by inducing a steric strain on the gRNA intramolecular helix and reduce the RT pausing [Citation60]. However, despite being highly structured, so far only a single structure has been resolved of a segment of the
primer annealed to the ARL motif of the PBS-segment RNA () [Citation46].
Specifically, this NMR structure revealed the formation of two coaxially stacked A-form RNA helices separated by two mismatched base pairs, U162-39 and G163-A38 (labelled in ). Two anticodon modifications, 2-thiouridine (s2U34) and pseudouridine (
), were determined to stabilize the interactions between the ARL anticodon and the ARL motifs (highlighted in red; ). In a different study, it was determined that the absence of the N1-methyl group at the A58 nucleotide in the primer
led to a decreased integration of the HIV-1 genome [Citation61,Citation62]. Additionally, the 5,
-O-dimethyluridine at the U54 nucleotide was hypothesized to act as a second RT-stop site but further studies are necessary to probe the mechanistic details of the termination of reverse transcription [Citation62].
NMR was further used for characterizing binding of hLysRS to the TLE motif in the HIV-1 initiation complex [Citation43]. The TLE motif mimics the L-shaped tRNA conformation, thus extending the global fold of the PBS/TLE RNA region (). It was shown that the anticodon-binding domain (ACB) of the hLysRS bound to the primer and the wild-type TLE with similar affinity [Citation43]. This observation implied that the interactions between the hLysRS protein and the TLE motif were required to trigger the release of
from the hLysRS protein [Citation43]. Importantly, the tRNA mimicry of the initiation complex was conserved, not only in the more commonly studied prototypical HIV-1 strain NL4–3, but also across another significant subset of the HIV-1 strains, the MAL strain [Citation63]. Furthermore, the phosphorylation of the S207 amino acid in the hLysRS protein was proposed to promote the preferential binding of the hLysRS protein to the PBS-segment RNA in an open conformation, distinct from that of a non-phosphorylated protein [Citation64].
Studies on PBS RNA and reverse transcriptase
The resulting 18-nucleotides long dsRNA interacts with the HIV-1 RT to engage in the minus-strand DNA synthesis [Citation65]. Multiple structures of the HIV-1 RT have been resolved since the first crystal structures of the RT were reported in 1990s [Citation66–69]. These structural data revealed a heterodimeric shape of the HIV-1 RT consisting of two subunits (p66 and p51 subunits). The p66 subunit contained two functional active sites, an N-terminal DNA polymerase which was subdivided into fingers, thumb, and palm, and a C-terminal ribonuclease H (RNase H). These RT structures helped in understanding the functions of the RT enzyme, assisting drug design, and explaining drug resistance of the RT enzyme [Citation25]. While a number of crystal structures of the individual RT domains or RT with vDNA are available [Citation50,Citation66–69] and reviewed elsewhere [Citation25], we chiefly focus on the interactions between RT and the HIV-1 RNA.
The RTIC structure in the RT-enzyme bound form of the /PBS complex was not resolved until 2014 [Citation48]. At first, the crystal structures of the RNA/DNA hybrid bound to the RT enzyme were reported [Citation55], revealing the conformational flexibility of the hybrid complex in comparison to the complex with two DNA strands in the active site. Then, two crystal structures of the HIV-1 RTIC with the RT enzyme bound to the
/PBS RNA complex at 4.5 Å and 3.95 Å resolutions were resolved using cryo-EM [Citation48,Citation49]. The first structure had the primer
-end positioned away (by
7 Å) from the priming site (P-site) due to the presence of an extra nucleotide at the
-end (denoted
+1 in ) [Citation49]. In the other structure, the primer
-end appeared closer to the P-site, but it still remained mispositioned with respect to the P site by
5 Å (denoted
in ) [Citation48]. The mispositioning of the
-end in both crystal structures was proposed to contribute to a significantly slower rate of the nucleotide incorporation by the initiation complex in comparison to the elongation complex of the RT [Citation48]. In a different study [Citation53], the vRNA strand was extended by three deoxynucleotides which also led to a slow rate of reverse transcription due to mispositioning of the
/PBS complex in the RT cleft of the RTIC.
Studies on PBS RNA and therapeutic agents
Additionally, two cryo-EM structures of the HIV-1 RTIC with two non-nucleoside reverse transcriptase inhibitors (NNRTIs) were resolved at 3.1 Å and 2.9 Å resolutions, respectively [Citation54]. Based on these structures, it was proposed that the NNRTIs stabilized the RTIC in the inactive conformation is incapable of properly positioning the structured vRNA template [Citation54]. Importantly, novel NNRTIs are designed to feature conformational flexibility and positional adaptability, thereby imposing a high genetic barrier to mutation of RT [Citation54]. Thus, these RTIC structures could serve as good models for characterizing the evasion mechanisms of NNRTIs against resistance mutations in RT. However, while a set of FDA-approved drugs which target RT are currently available as part of the ART [Citation70], there continues to be a need for novel inhibitors targeting either RT or the vRNA during reverse transcription. Several different types of promising inhibitor strategies are undergoing testing, including the anti-RT RNA aptamers [Citation71], the ruthenium-based compounds [Citation72,Citation73], and the CRISPR-Cas9 gene-editing technology [Citation74,Citation75]. These strategies yielded high selectivity and high activity towards HIV-1 RNA during reverse transcription but further work is necessary to characterize the inhibition mechanisms.
Studies on PBS RNA and NC protein
Another important factor of the reverse transcription process is the mature NC protein which facilitates the two obligatory strand transfers during reverse transcription [Citation76]. After reaching the -end of the genome, the intermediate minus-strand strong-stop DNA (−sssDNA) is synthesized from the annealed dsRNA [Citation77]. To complete the synthesis, the minus-strand DNA is transferred to the
-end of the same or the other co-packaged RNA genome [Citation77,Citation78]. This process is commonly referred to as the first strand transfer or the minus strand transfer. This transfer occurs from the repeat sequence R (consists of TAR and polyA hairpins) at the
-end of the minus-strand DNA to an equivalent R region at the
-end of the co-packaged RNA strand [Citation78,Citation79]. The NC protein was shown to promote the annealing of the TAR RNA element from the R region with the complementary TAR sequence from the R region in synthesized vDNA during the first (minus) strand transfer [Citation78,Citation80]. Based on the NMR data, it was proposed that annealing by the NC protein was performed through the destabilization of several base pairs in the upper part of the TAR hairpin, adjacent to the stem-loops [Citation80]. These findings were further confirmed by performing single-molecule optical tweezers experiments which revealed that the NC cleavage from the Gag polypeptide improved the NC selectivity for the TAR RNA destabilization [Citation81].
Once -sssDNA is transferred and annealed to the -end of the viral RNA, minus-strand DNA synthesis continues, while the RNA template strand is degraded by RNase H [Citation82]. However, the RNA genome contains a short polypurine tract (PPT) which is resistant to RNase H degradation [Citation82]. The PPT serves as a primer for the plus-strand DNA synthesis which is stopped after a portion of the primer tRNA is reverse-transcribed while generating a plus-strand strong-stop DNA (+sssDNA) [Citation82]. The primer tRNA is removed by the RNase H and the +sssDNA is annealed to the
-end of the minus-strand DNA resulting in the second strand transfer [Citation77,Citation82]. Then, the plus- and minus-strand syntheses are completed while the two strands of DNA serve as templates for each other [Citation82]. The NC protein promotes annealing of the 18-nucleotide long complementary DNA PBS sequence at the
ends of the positive and negative DNA strands [Citation52]. A structure of the complex of HIV-1 vDNA with the nucleocapsid protein 7 (NCp7), which promotes annealing of the 18-nucleotide long complementary DNA PBS sequence during strand transfer, was resolved using NMR [Citation52]. The structure revealed that melting of the PBS stem-loop could be promoted by NCp7 which weakened the C5-G11 base pair next to the stem-loop and opened the PBS stem-loop [Citation52].
Structural and biochemical studies on HIV-1 TAR RNA
Brief overview of TAR RNA and its role in transcription
After the HIV-1 RNA genome is reverse transcribed into double-stranded vDNA, it gets integrated into the host-cell chromosome (step 5; ). The integration of vDNA is performed in a multi-step procedure by the viral integrase (IN) enzyme which forms the intasome complex with vDNA described elsewhere [Citation28]. The integrated vDNA (provirus) serves as the template for the transcription of viral RNA. Importantly, the presence of the HIV-1 provirus is detected even after the HIV-1 infection is suppressed with ART for an extended time-period [Citation83–85]. The DNA provirus is transcribed by the cellular machinery into the vRNA transcripts, which encode a variety of viral proteins or further participate in the RNA genome packaging.
One of the key steps in HIV-1 transcription is the binding of Tat protein to TAR RNA. TAR RNA adopts an A-form helical stem loop with a three-nucleotide bulge motif that divides the structure into two helices (Helix 1 and Helix 2) and capped by an apical loop (). The arginine rich motif (ARM) of the Tat protein recognizes TAR RNA near the bulge motif and further recruits the host-cell super elongation complex (SEC) [Citation23]. SEC includes two complexes, the positive transcript elongation factor b (P-TEFb) complex which contains CDK9 and cyclin T1 and the polymerase-associated factor 1 (PAF-1) complex [Citation23]. Therefore, the disruption of the TAR-Tat interaction represents a promising target for antiretroviral therapeutics. To achieve this goal, a variety of ligands have been designed to perturb the TAR-Tat interaction and inhibit the transcription process (; ).
Figure 3. TAR RNA and its complexes with various ligands. Shown is (A) the secondary structure of the HIV-1 TAR RNA highlighting various structural motifs (loop, helix 2, bulge, helix 1) that are labeled and colored uniquely; (B) the snapshots of TAR RNA (stick representation) bound to various peptides (left; labeled counterclockwise light orange) and to small-molecules (right; labeled counterclockwise light blue). The ligands are shown in space-filling representations [Citation86–95] with the apo conformation of TAR RNA (PDB 1ANR) shown at the center of the panel [Citation96]; (C) the sequences of the 2–
3 loops from four lab-evolved TBPs with key arginine residues highlighted in boxes; (D) the cartoon representation of superimposed TBPs bound to TAR RNA with the
2–
3 loops and the key arginine residues and RNA nucleotides (stick representations) highlighted in unique colors following the color scheme in panel C [Citation97,Citation98]; (E) the snapshot of the TBP6.7 binding site with key arginine residues depicted with sticks (PDB 6HX1) [Citation97]; and (F) the snapshot of the G1G truncated joint region between TAR RNA and PolyA RNA along with the truncated secondary structure (PDB 7DD4) [Citation51]. The
-terminal G2 and G3 nucleotides and the
-terminal UG nucleotides are highlighted in unique colors.
![Figure 3. TAR RNA and its complexes with various ligands. Shown is (A) the secondary structure of the HIV-1 TAR RNA highlighting various structural motifs (loop, helix 2, bulge, helix 1) that are labeled and colored uniquely; (B) the snapshots of TAR RNA (stick representation) bound to various peptides (left; labeled counterclockwise light orange) and to small-molecules (right; labeled counterclockwise light blue). The ligands are shown in space-filling representations [Citation86–95] with the apo conformation of TAR RNA (PDB 1ANR) shown at the center of the panel [Citation96]; (C) the sequences of the β2–β3 loops from four lab-evolved TBPs with key arginine residues highlighted in boxes; (D) the cartoon representation of superimposed TBPs bound to TAR RNA with the β2–β3 loops and the key arginine residues and RNA nucleotides (stick representations) highlighted in unique colors following the color scheme in panel C [Citation97,Citation98]; (E) the snapshot of the TBP6.7 binding site with key arginine residues depicted with sticks (PDB 6HX1) [Citation97]; and (F) the snapshot of the G1G truncated joint region between TAR RNA and PolyA RNA along with the truncated secondary structure (PDB 7DD4) [Citation51]. The 5′-terminal G2 and G3 nucleotides and the 3′-terminal UG nucleotides are highlighted in unique colors.](/cms/asset/6ba6f735-c6bf-435f-9d78-f324bf270dae/krnb_a_2289709_f0003_oc.jpg)
Table 2. Structural data on the HIV-1 RNA elements participating in transcription. Data similar to are shown for the HIV-1 RNA elements participating in transcription, and the following additional abbreviations are used: G-quadruplex, guanine-rich quadruplex; SEC, super elongation complex; Tat, transactivator of transcription; TBP, TAR-binding protein.
The ability of TAR RNA in binding various inhibitors and proteins is rooted in the conformational flexibility inherent to various secondary structure motifs (e.g. bulge; ) of TAR RNA, as characterized by the experimental [Citation103] and computational methods [Citation104]. While a number of inhibitors have been designed to target TAR RNA with moderate-to-high binding affinity, none of these compounds have reached the clinical trials stage [Citation86,Citation97]. Therefore, continued effort is required for identifying new lead structures which can bind TAR RNA with high selectivity. It is also necessary to further characterize the dynamics governing the recognition mechanisms between TAR RNA and the various elements of the SEC since our knowledge is limited to a few crystal structures [Citation99,Citation102,Citation105]. In this section, we highlight the impact of structural studies of the HIV-1 TAR RNA molecules on our understanding of the transcription process ().
Studies on TAR RNA and therapeutic agents
A number of experimental structures exist for TAR RNA bound to small-molecules with inhibitory properties () [Citation87–91,Citation93,Citation96]. Most small-molecules are known to bind TAR RNA in the major groove of the Helix 2, with the exception of neomycin B which binds to TAR RNA within the Helix 1 (1QD3; ). Binding of small-molecules, except the acetylpromazine molecule (1LVJ; ), disrupted the stacking interactions between the U23 bulge nucleotide and the A22 nucleotide from the Helix 1, thereby inducing the outward flipping of at least one of the bulge nucleotides (e.g. 1UUD; ). These local conformational changes resulted in the formation of a coaxially-stacked configuration of TAR RNA without a binding site for the Tat protein. Interestingly, while the binding of the acetylpromazine ligand perturbed the coaxially-stacked conformation, it assisted in the formation of a continuous stack of 10 bases (including benzene ring of the ligand) [Citation89].
Importantly, in the unbound TAR RNA structure, the U23 nucleotide stacked on the A22 nucleotide, causing helical conformation in RNA and providing a binding site for the Tat protein (1ANR; ). Thus, these structures revealed TAR RNA as a flexible structure sampling various interhelical states around the bulge motif which can be stabilized with small-molecules. We conducted a set of molecular dynamics (MD) simulations of TAR RNA in the apo and ligand-bound (small-molecules or cyclic peptides) configurations [Citation106]. Our simulations further confirmed TAR RNA to be a flexible molecule and revealed that the conformational heterogeneity in TAR RNA facilitates recognition of a diverse set of ligands [Citation106].
Other types of inhibitors for which the crystal structures are not available were developed to perturb the interaction between Tat and TAR RNA, including the metallo supramolecular helicates with iron(II), nickel(II), and ruthenium(II) metals [Citation107,Citation108]. Another group utilized the existing NMR structure of TAR RNA (PDB 1LVJ) to perform in silico screening of compounds from the Enamine database [Citation109]. In silico docking identified 173 compounds which were experimentally tested to identify a novel compound which could potentially serve as a new lead structure for future anti-HIV-1 therapeutics [Citation109].
Another therapeutic strategy was proposed as a method to eradicate the latent HIV-1 provirus copies deposited in the host cells’ genome [Citation110]. Specifically, it was revealed that the methyltransferase complex METTL3/METTL14/WTAP enhanced HIV-1 transcription and production of virus particles by introducing m6A modifications to viral mRNA molecules [Citation110]. This protein complex could be activated by a set of small-molecules which enhanced m6A methylation of the HIV-1 mRNA [Citation110]. Therefore, these activators of the latent HIV-1 provirus in the host-cell genome were proposed as targets for novel antiviral drugs [Citation110].
Studies on TAR RNA and cyclic peptides
TAR RNA has also been targeted with cyclic peptides which mimic the ARM of the Tat protein [Citation86,Citation92,Citation94,Citation95] (; ). The peptides bind to TAR RNA through reorganization of the apical loop and bulge nucleotides which almost enclose various peptide ligands (). Interestingly, the peptides induced a conformation in TAR RNA resembling the structure of TAR RNA bound to the Tat protein and rearranged the apical loop for potential binding of the host-cell factors [Citation86]. However, the peptides were not completely buried into the binding site of RNA and had the D-Pro-L-Pro end of the peptide protrude away from the RNA structure, thereby exposing itself to the solvent [Citation94]. While designing these peptides, positively-charged amino acid sidechains were proposed to play the most crucial role in recognition of RNA by the peptide. MD simulations further confirmed these findings and revealed that the rearrangements of ions around the RNA/peptide complexes play an important role in accommodating the peptide in the binding pocket [Citation111].
Studies on TAR RNA and lab-evolved proteins
Another class of TAR inhibitors consisted of a set of lab-evolved TAR-binding proteins (TBPs) which mimic the ARM of the Tat protein () [Citation97,Citation98,Citation112]. These TBPs formed a set of conserved arginine-to-guanine interactions within a lab-evolved 2-
3 loop and inhibited the Tat-TAR interaction () [Citation97,Citation98,Citation112]. For example, the R47, R49, and R52 amino acids of the TBP6.7 protein formed hydrogen-bonding interactions with the G26, G28, and G36 nucleotides of TAR RNA (). Based on the biochemical data [Citation97,Citation98], it was further proposed that variations in the compositions as well as the positions in the
2-
3 loop altered the affinity of TBPs for TAR RNA. TBPs present an alternative route for inhibiting TAR RNA function but warrant further optimization. We probed these interactions using the free energy methods and reported on the energetic contributions of individual arginine residues in the
2-
3 loop for binding to TAR RNA [Citation113].
Studies on the joint region between TAR RNA and PolyA RNA
TAR RNA was also determined to play an important role in reverse transcription. Another key segment of the UTR of the HIV-1 genome is the joint region between the TAR RNA and the PolyA stem () [Citation51]. The NMR structure revealed the formation of the coaxially stacked stem between TAR RNA and PolyA RNA with the U106 nucleotide in a flipped out conformation () [Citation51]. It was further revealed that the number of guanine residues at the
-end influenced the conformation of the joint region, thereby affecting reverse transcription and transcription rates [Citation51,Citation114]. Specifically, in the case of G1G, with an additional guanine residue added to the already present
-cap guanine, two GC base pairs induced the formation of the coaxially stacked structure between two RNA stems (green and red nucleotides in ) [Citation51]. On the contrary, the addition of two (G2G) and three (G3G) guanine residues to the innate
-cap guanine of the joint region RNA, resulted in weaker interactions between the TAR RNA and PolyA RNA stems in comparison to the G1G system [Citation51].
Studies on TAR RNA and Tat protein
While a significant role of the ARM in TAR RNA binding has been validated previously using biochemical studies [Citation115,Citation116], the solution structures of the HIV-1 Tat ARM bound to the HIV-1 TAR RNA and to the human 7SK RNA were resolved in 2018 () [Citation99]. These structures provided mechanistic details on the Tat protein which competed with the 7SK small nuclear ribonucleoprotein to displace HEXIM adapter protein and to recruit P-TEFb [Citation99,Citation117]. Specifically, the Tat protein adopted a bent configuration with three arginine residues interacting with 7SK RNA (highlighted in red; ). Conversely, Tat adopted a completely different linear configuration with two arginine residues interacting with TAR RNA (highlighted in red; ) [Citation99]. Interestingly, the fluorescence microscopy showed that TAR RNA promoted the folded state of the Tat protein which differed from the linear configuration of the Tat protein observed in the NMR structure () [Citation118].
Figure 4. Tat protein in complex with 7SK RNA and TAR RNA. Shown are the side-view snapshots of the Tat protein bound to (A) human 7SK RNA (PDB 6MCF) and (B) TAR RNA (PDB 6MCE) [Citation99]. In both snapshots, RNA is shown in a cartoon representation (gray), Tat backbone is shown in a tubular representation (yellow), the atoms of those arginine residues which participate in key interactions with both RNA molecules are shown in red spheres, while other arginine residues are shown in blue spheres. The zoomed snapshots correspond to specific interactions formed between the arginine residues in Tat (red sticks) and the RNA nucleotides.
![Figure 4. Tat protein in complex with 7SK RNA and TAR RNA. Shown are the side-view snapshots of the Tat protein bound to (A) human 7SK RNA (PDB 6MCF) and (B) TAR RNA (PDB 6MCE) [Citation99]. In both snapshots, RNA is shown in a cartoon representation (gray), Tat backbone is shown in a tubular representation (yellow), the Cα atoms of those arginine residues which participate in key interactions with both RNA molecules are shown in red spheres, while other arginine residues are shown in blue spheres. The zoomed snapshots correspond to specific interactions formed between the arginine residues in Tat (red sticks) and the RNA nucleotides.](/cms/asset/74d8959c-b96d-498d-9b23-26488137958d/krnb_a_2289709_f0004_oc.jpg)
Studies on TAR RNA and host-cell factors
Furthermore, upon the binding of Tat protein to TAR RNA, various host-cell factors are recruited to regulate the transcription process and identifying these factors could present novel targets for inhibitor development [Citation119]. The most crucial host-cell factors were various SEC components which have been crystallized in complex with TAR RNA and Tat protein [Citation102,Citation105]. These structures revealed mechanistic insights into transcriptional regulation and key interactions formed between TAR RNA and proteins [Citation102,Citation105]. Other host-cell factors which were proposed to interact with HIV-1 RNA and regulate its function were the nuclear factors 45 and 90 (NF45, NF90) [Citation119]. Through the in vitro overexpression studies, the NF45/NF90 proteins were identified to stabilize HIV-1 RNA and enhance gene expression [Citation119]. However, it could not be excluded that these factors were mediating the expression of other host factors while not directly interacting with TAR RNA and further studies are necessary to confirm the role of NF45/NF90 [Citation119]. The binding of the C-terminal domain of HIV-1 IN to TAR RNA was also proposed to induce structural rearrangements in the major groove of TAR RNA, which favoured the subsequent binding of Tat to TAR RNA while displacing IN from TAR RNA [Citation120].
Structural and biochemical studies on HIV-1 G-quadruplexes
G-quadruplex DNA or RNA motifs formed in the long terminal repeat (LTR) are considered as alternate targets for therapeutic intervention [Citation100,Citation101,Citation121,Citation122]. G-quadruplexes are the secondary structures formed by guanine-rich sequences (). A number of compounds have been developed for inhibiting the transcription process by targeting the HIV-1 LTR G-quadruplex DNA [Citation121]. To gain structural insights for altering the selectivity of ligands targeting G-quadruplexes, NMR was used to resolve the structure of a biologically relevant G-quadruplex DNA within the LTR region of HIV-1 [Citation100]. Another NMR structure of LTR-III DNA showed the formation of a G-quadruplex conformation in vitro () [Citation101]. The structure revealed a quadruplex-duplex hybrid consisting of a three-layer (3 + 1) G-quadruplex scaffold which included a 12-nucleotide diagonal loop between the residues G3 and T14, a 3-nucleotide lateral loop between the residues A22 and T24, and a V-shaped loop between the residues G25 and G26 (). The formation of this G-quadruplex element in the U3 promoter region of the LTR RNA was further confirmed by optical and thermodynamic experiments [Citation123]. In a different study [Citation122], 2177 complete HIV-1 genomes were screened to identify conserved G-quadruplexes, which can be inhibited with the porphyrin-based G4 binders. However, considering the high abundance of the G-quadruplex structures in human cells, it remains challenging to achieve high selectivity towards the HIV-1 G-quadruplexes [Citation100,Citation101].
Figure 5. The G-quadruplex conformation from LTR-3. Two snapshots of a G-quadruplex DNA structure from the LTR-3 along with the sequence of the LTR-3 domain are shown. DNA is depicted in a ribbon representation (gray); guanine, adenine, thymine, and cytosine bases are depicted in cyan, green, orange, and red stick representations, respectively. A pink arrow in the right panel follows the V-shape of the -end loop.

Structural and biochemical studies on HIV-1 RRE RNA
Brief overview of RRE RNA and its role in nuclear export
After transcription is completed, fully spliced, partially spliced, and unspliced RNAs in retroviruses are exported out of the nucleus into the cytoplasm for further translation and virion assembly (step 7; ). Fully spliced RNA molecules in general can be exported out of the nucleus without any restriction factors in mammalian cells [Citation130]. However, nuclear export of unspliced or partially spliced RNA transcripts is facilitated by two key viral elements, the regulator of expression of virion (Rev) protein and the highly structured and conserved RRE RNA which is present in all unspliced or partially spliced RNAs [Citation130,Citation131]. Rev is initially synthesized from spliced mRNA which is exported from the nucleus by the same pathway as the host-cell mRNA [Citation130,Citation132]. Rev molecules then diffuse to the nucleus and bind to the upper stem-loop IIB of the RRE RNA [Citation133] and are further relayed to specific binding sites in RRE that allow for Rev oligomerization, as confirmed by the native mass spectrometry [Citation134]. However, we still lack a complete understanding of the assembly mechanism of six Rev molecules on RRE RNA and if the oligomerization follows only a single pathway or multiple pathways [Citation133].
The resulting Rev/RRE complex recruits host factors CRM1 and RanGTP to enable nuclear export [Citation135,Citation136]. It was further demonstrated that PACS1 protein interacted with the Rev protein and its cofactor CRM1 and contributed to nuclear export of HIV-1 RNAs [Citation137]. Various structural studies have enhanced our understanding of (1) the interactions formed between RRE RNA and Rev protein, (2) the functional roles of RRE RNA and Rev protein, and (3) the oligomerization mechanism of the Rev protein induced by RRE RNA (). Other important factors to consider include the effect of the sequence of RRE RNA and Rev protein on the binding and oligomerization of Rev molecules, the dynamics behind oligomerization mechanism of Rev molecules at the interface with RRE RNA, and the development of novel inhibitors which target RRE RNA.
Table 3. Structural data on the HIV-1 RNA elements participating in nuclear export. Data similar to are shown for the HIV-1 RNA elements participating in nuclear export and the following additional abbreviations are used: Rev, regulator of expression of virion; RRE, Rev response element.
Studies on apo RRE RNA
The RRE RNA was proposed to fold into a structured RNA with five distinct stem-loops (I – V) [Citation31,Citation138]. However, other studies have proposed that the RRE RNA could form an alternate structure containing four stem-loops [Citation139–141]. Specifically, in the four stem-loop configuration, the SL III and IV of the five SL RRE combined forming a single stem-loop in the central loop [Citation141]. Biochemical and structural studies established a high affinity binding site in the stem-loop IIB of the RRE RNA () [Citation125,Citation126,Citation142]. The crystal structure of the apo RRE-IIB RNA was resolved at 1.6 Å resolution revealing a distorted A-form geometry with the A68 and U72 nucleotides in the flipped-out configurations [Citation125]. The conformation of the RRE RNA in the crystal structure had a similar overall shape to the NMR structure of the RRE RNA [Citation125,Citation143]. However, the G48-G71 base-pair in the crystal structure had an asymmetric configuration, while the NMR model of the free RRE had a symmetric G48-G71 base-pair. The asymmetric G48-G71 base-pair in the RRE crystal structure altered the local shape of the RRE RNA near the U72 nucleotide, making the major groove narrower [Citation125]. The differences across the two structures were proposed to result from the NMR-derived structure being less constrained [Citation125].
Figure 6. RRE-IIB RNA in complex with the helical peptides. Shown are (A) the sequences of the HIV-1 RRE-IIB RNA, the Rev peptide, and the RSG-1.2 peptide with key nucleotides and amino acids highlighted in blue and red, respectively; (B) the snapshots of the HIV-1 RRE-IIB RNA bound to Rev peptide (PDB 1ETF) [Citation124]; and (C) the snapshots of the HIV-1 RRE-2B RNA bound to the RSG-1.2 peptide (PDB 1G70) [Citation127]. In all snapshots, RNA is shown in a cartoon representation (light green) with the blue spheres corresponding to phosphorous atoms for those nucleotides which form crucial interactions with the peptides and with the green spheres corresponding to phosphorous atoms in the backbone of other RNA nucleotides; the peptide backbone is shown in a tubular representation (white) with red spheres corresponding to the atoms of key arginine residues. The snapshots of the entire RNA/peptide complexes are accompanied with snapshots highlighting the corresponding interactions between key arginine amino acids and RNA nucleotides which are represented as red and blue sticks, respectively.
![Figure 6. RRE-IIB RNA in complex with the helical peptides. Shown are (A) the sequences of the HIV-1 RRE-IIB RNA, the Rev peptide, and the RSG-1.2 peptide with key nucleotides and amino acids highlighted in blue and red, respectively; (B) the snapshots of the HIV-1 RRE-IIB RNA bound to Rev peptide (PDB 1ETF) [Citation124]; and (C) the snapshots of the HIV-1 RRE-2B RNA bound to the RSG-1.2 peptide (PDB 1G70) [Citation127]. In all snapshots, RNA is shown in a cartoon representation (light green) with the blue spheres corresponding to phosphorous atoms for those nucleotides which form crucial interactions with the peptides and with the green spheres corresponding to phosphorous atoms in the backbone of other RNA nucleotides; the peptide backbone is shown in a tubular representation (white) with red spheres corresponding to the Cα atoms of key arginine residues. The snapshots of the entire RNA/peptide complexes are accompanied with snapshots highlighting the corresponding interactions between key arginine amino acids and RNA nucleotides which are represented as red and blue sticks, respectively.](/cms/asset/7db24a2f-cd68-4db2-9c99-c98656e29089/krnb_a_2289709_f0006_oc.jpg)
Furthermore, in a separate study, it was proposed that the stem-loop IIB could form an ensemble of three conformations in vitro including two non-native excited states [Citation144]. These states could be potentially targeted with novel inhibitors, but further studies in cellular environment are necessary [Citation144]. Additionally, the m6A modification was shown to have a minimal effect on the stability, structure, and dynamics of the RRE stem IIB as well as its binding affinity to the Rev ARM [Citation145]. Therefore, the conformational flexibility at the stem-loop II of RRE RNA is crucial for the binding of both the Rev protein and the host-cell cofactors [Citation145]. A separate study of the impact of the nucleotide substitutions in the RRE RNA in an HIV-1 strain circulating in China revealed that the mutations at the sites 56, 57, and 186 could increase the viral mRNA transcription and the Rev-RRE activity [Citation146]. However, only mutation at the site 186 significantly improved the viral replication ability [Citation146].
Studies on RRE RNA and Rev peptide
Similar to Tat protein, Rev contains the ARM which binds at the major groove of the RRE-IIB RNA () [Citation124]. A solution structure of the short helical peptide corresponding to the ARM of the Rev protein was resolved using NMR [Citation124]. The peptide was shown to bind at the major groove in stem-loop IIB which was coupled with the formation of a number of salt-bridging and hydrogen-bonding interactions between the arginine residues and RNA nucleotides () [Citation124]. Specifically, key interactions were identified between the residues R35, R39, R44, R46, and R50, and the nucleotides G67, G71, U45, A68, and U72, respectively (inset; ). The importance of these arginine amino acids for the recognition of the RRE RNA by the Rev peptide was validated using mutational analysis [Citation124]. The R46 and R50 amino acids were identified to have significant contributions to the recognition of RRE RNA by the Rev peptide [Citation124]. A set of MD simulations were further utilized to probe these interactions, proposing shape-selectivity as a critical factor for the recognition of the Rev peptide by the RRE RNA [Citation147].
Additionally, cooperative binding of the Rev peptides to the RRE RNA has been established using the X-ray methods [Citation129]. The crystal structure of the Rev dimer-RRE complex revealed rearrangements of the Rev-dimer hydrophobic interface after binding to the RRE RNA [Citation129]. The oligomerization of Rev proteins was further enhanced by the binding of the cellular DDX1 proteins to the RRE RNA [Citation148]. The associated structural transitions were identified within the three-way junction of the stem-loop 2 of the RRE RNA which was known to be responsible for initial Rev binding [Citation148]. In another study [Citation149], novel amino acid substitutions were identified in the oligomerization domain of the Rev protein which affected the Rev-RRE functional activity. The substitutions of two amino acids at the positions 14 and 19 were shown to sufficiently alter the activity of the Rev protein [Citation149].
Furthermore, it was demonstrated that the CRM1 and the N×F1 host-cell cofactors mediated the nuclear export of HIV-1 RNA in the cell cytoplasm following the same diffusion transport mechanism [Citation150]. This finding further emphasized the flexible nature of the HIV-1 replication process, thereby highlighting the significance of studying all possible pathways of nuclear export and the interactions formed between the RRE/Rev complex and various host-cell cofactors [Citation150].
Studies on RRE RNA and RSG-1.2 peptide
In a different study, an RSG-1.2 peptide was designed to mimic Rev protein by forming a similar -helical configuration with multiple arginine residues and to target the major groove of the RRE-2B RNA () [Citation127,Citation128]. The RSG-1.2 peptide was shown to be stabilized in the binding site formed by the major groove of the HIV-1 RRE-2B RNA () [Citation127]. The RSG-1.2 peptide was reported to bind RRE RNA with seven-fold higher affinity and 15-fold higher specificity than the original Rev peptide [Citation127]. Similar to the Rev peptide, a network of salt-bridging and hydrogen-bonding interactions between the RSG-1.2 peptide and the HIV-1 RNA was characterized based on the NMR structure (right; ). Using non-equilibrium computer simulations, we proposed a preferred (un)binding mechanism of the RSG-1.2 peptide from RRE RNA [Citation151]. The preferred pathway was characterized by the formation of metastable states that resulted from a network of salt bridges formed between the arginine amino acids and the phosphate groups of the RNA backbone as well as from the hydrogen bonding [Citation151]. Furthermore, we identified three arginine residues (R8, R14, and R15) to be crucial for peptide recognition by the RRE RNA and revealed the role of water molecules in the binding pocket [Citation151].
Biochemical studies on HIV-1 mRNA
Brief overview of HIV-1 mRNA and its role in translation
The transcription and alternative splicing results in over 30 different mRNA species which are exported to the cell cytoplasm. Here, the translation of viral mRNA depends exclusively on the host-cell translational machinery (step 8; ) [Citation152]. The newly synthesized HIV-1 mRNAs display characteristics similar to the host-cell mRNAs. The unspliced, partially spliced, and fully spliced RNA transcripts encode various HIV-1 proteins which are synthesized in cellular ribosomes. Specifically, unspliced RNA transcripts could either encode Gag and Gag-Pol polyproteins or serve as gRNAs to be assembled into newly synthesized virus particles; partially spliced RNA transcripts could encode Env/Vpu, Vif, Vpr, and a truncated form of Tat; and fully spliced RNA transcripts could encode Rev, Tat, and Nef proteins [Citation153,Citation154]. The translation process of HIV-1 mRNA follows similar steps as the translation process of the host-cell mRNAs: initiation, elongation, termination, and ribosome recycling steps [Citation155].
However, contrary to other viruses which have the internal ribosome entry site (IRES)-dependent mechanism of translation, HIV-1 can use various mechanisms of ribosomal recruitment [Citation156]. These mechanisms include the cap-dependent mechanism, the IRES-dependent mechanism, and the methylation-dependent mechanism [Citation156]. Thus, the translation of HIV-1 mRNAs is a complex process requiring further characterization with multiple possible mechanisms of hijacking cellular ribosomes [Citation156]. Although structural studies have been carried out on the translation process of other viruses [Citation157–159], no experimentally resolved structures are available for the translation process in HIV-1. Here, we describe the results from biochemical studies (2016 through 2023) on this process. These studies provide valuable insights on various steps of the translation process, but in the future it could be complemented with structural data.
Biochemical studies of the initiation step
The initiation step is one of the most crucial steps in the HIV-1 translation process [Citation155,Citation160]. This step can be mediated either by a cap- or an IRES-dependent mechanism which again highlights the flexible nature of the HIV-1 replication [Citation152]. Therefore, it is important for us to understand which of these mechanisms is more crucial to the translation process or if they are equally important [Citation152,Citation161]. It has been previously shown that if the cap-dependent mechanism was compromised, the initiation of the HIV-1 RNA translation switched to the IRES-dependent mechanism [Citation161,Citation162]. By comparing the translational efficiency of different mRNA transcripts, it was proposed that the contribution of the IRES-dependent mechanism was negligible in comparison to the cap-dependent mechanism [Citation161]. In another study [Citation163], it was shown that Staufen1, a cellular dsRNA-binding protein (RBP), could bind the -UTR of HIV-1 and promote the cap-dependent mechanism of the HIV-1 mRNA translation. Importantly, it was further revealed that Staufen1 could also enhance the IRES-dependent mechanism of translation [Citation164]. Thus, Staufen1 could contribute to the cap-dependent translation as well as to the (IRES)-dependent mechanism [Citation164].
Biochemical studies of the -1PRF mechanism
After the initiation and the attachment of the viral mRNA to the host-cell ribosome steps are completed, the translation process in HIV-1 proceeds by the −1 programmed ribosomal frameshifting (PRF) mechanism [Citation165]. During the PRF, a ribosome is guided to an open reading frame (ORF) of mRNA bypassing the original stop codon and performing protein synthesis [Citation166]. Many viruses, including HIV-1 utilize the −1 PRF where frameshifting is shifted one nucleotide position downstream the mRNA sequence [Citation166]. Different models have been proposed describing the PRF mechanism with a common feature that mRNA should be under a mechanical tension while the ribosome is reading the ORF during frameshifting.
Therefore, a single-molecule force spectroscopy (SMFS) technique was applied to elucidate the mechanistic properties of the frameshift stimulating structure (FSS) hairpin of mRNA by mimicking the ribosome tension [Citation167]. The force applied to unfold the mRNA hairpin was then used to estimate the energy landscape and kinetic parameters of the unfolding process [Citation167]. The resulting kinetic and thermodynamic data established only a single unfolding pathway with a simple two-state behaviour [Citation167]. However, the refolding process was more complex, characterized by multiple refolding pathways with partially folded intermediate states [Citation167]. This conformational heterogeneity of the mRNA hairpin demonstrated the inherent flexibility of this motif [Citation167].
Additionally, the frameshifting efficiency was shown to be coupled with the thermodynamic stability of the FSS mRNA hairpin [Citation168]. Interestingly, certain mutations in the structure-stimulating hairpin increased the frameshifting efficiency but decreased the infectivity of HIV-1 [Citation168]. These insights on the properties of the structure-stimulating mRNA hairpin could be important for the development of novel therapeutic agents which can target the mRNA during the −1 PRF [Citation168]. However, the dynamics in the structure-stimulating mRNA hairpin and its link to the −1 PRF remain poorly understood. Therefore, continued efforts are necessary to establish a complete mechanism of the −1PRF in the HIV-1 infected cells.
Therapeutic agents inhibiting translation
A triazole-containing compound was designed to bind the FSS hairpin with high affinity and selectivity while retaining low toxicity in comparison to the previously developed compounds [Citation169]. Another strategy proposed to inhibit the HIV-1 translation process was increasing the number of the CpG dinucleotides in the HIV-1 RNA transcripts which encode the Gag polyprotein [Citation170,Citation171]. It was shown that an increase in the number of the CpG dinucleotides was coupled with the inhibition of various steps in the HIV-1 replication cycle [Citation170,Citation171]. Furthermore, the zinc-finger antiviral proteins (ZAP) were also shown to selectively target the CpG dinucleotides in HIV-1 RNA molecules and to degrade these RNA molecules [Citation172]. Therefore, a novel mechanism of the HIV-1 RNA degradation was proposed to utilize the poly(ADP-ribose) (PAR) polynucleotides which acted as regulators of the ZAP activity [Citation172].
Role of post-transcriptional modifications in translation
While a number of epitranscriptomic modifications of adenine and cytidine were detected in the HIV-1 RNA transcripts, their functions and effects on the translation process are not fully understood [Citation173–175]. For example, studies on the m6A modification revealed the increased number of methylated sites in mRNA resulted in enhanced translation efficiency [Citation173,Citation176]. The liquid chromatography-mass spectrometry analysis (LC/MS) of virion RNA also revealed high amounts of methylations at the N1 position (m1A) which were mostly present in tRNA packaged into the virion [Citation177]. Another variation of LC/MS, the ultra high-performance liquid chromatography linked to tandem mass spectrometry (UPLC-MS/MS) was utilized to demonstrate higher abundance of m5C modifications in HIV-1 gRNAs than in cellular mRNAs [Citation174]. The loss of m5C modification disrupted the alternative splicing of the mRNA transcripts and the translation process [Citation174]. The acetylations of the N4 position of cytidines (ac4C) by NAT10 enzyme were used by HIV-1 to promote translation of HIV-1 mRNAs [Citation178]. It was demonstrated that the NAT10 inhibitor remodelin could inhibit the viral replication process, suggesting ac4C as a potential target for antiviral drug development [Citation178].
Structural and biochemical studies on HIV-1 Psi RNA
Brief overview of Psi RNA and its role in genome dimerization
HIV-1 packages two copies of gRNA with the same genetic information for further production of an infectious viral particle during the late stage of the HIV-1 replication cycle (step 9; ) [Citation6,Citation200]. This is a result of the dimerization process during which two gRNA strands are non-covalently joined to each other through a number of intermolecular short- and long-range interactions formed between various gRNA motifs at the base pair level [Citation7,Citation201]. Dimerization and further packaging of gRNA are essential steps in the final HIV-1 virion assembly stage which occurs at the plasma membrane. The packaging and dimerization processes are proposed to be mediated by the Gag polyprotein, but the regulation mechanism and the binding location in the cell are still under debate [Citation202]. Specifically, it was determined that as the Gag concentration increased, gRNA shifted towards a new dimeric conformation, as evidenced by the in-gel SHAPE technique [Citation203]. Thus, it was proposed that a specific amount of Gag protein had to be translated to initiate the gRNA packaging mechanism [Citation203].
The genome dimerization process is initiated at a conserved element located within the packaging signal (psi or ) which is comprised of four stem-loops (SL1–SL4) in the
-leader of gRNA () [Citation7,Citation197]. Specifically, the stem-loop 1 (SL1) motif contains a four to six-nucleotide long GC-rich palindromic element, also referred to as the dimerization initiation site (dis; ) [Citation7,Citation197]. gRNA dimerization is proposed to follow an ordered series of structural rearrangements in gRNA conserved among various HIV-1 strains () [Citation204]. The primary dimerization step involves the formation of a kissing-loop complex through base pairing of six self-complementary nucleotides present in the DIS loops of two gRNA strands () [Citation205]. The interactions between the two stem-loops are preserved, while additional intermolecular interactions are then formed between the DIS stems of the kissing loop complex which results in an extended duplex of two gRNA strands () [Citation197,Citation206–208].
Figure 7. Structural features of the dimerization process. Shown are (A) the scheme and secondary structure of the dimerization process; (B) the snapshot of the overall kissing complex and the zoomed-in view of the kissing loop region (PDB 2D1B) [Citation183]; and (C) the example snapshots of two extended duplexes which display different configurations of the bulged nucleotides (PDBs 462D and 2D1A) [Citation183,Citation185]. The zoomed-in view on one of the bulged motifs is provided for the second extended duplex. In each panel, nucleotides of the DIS kissing loops (G274 to C279) participating in the recognition process are highlighted in darker colors.
![Figure 7. Structural features of the dimerization process. Shown are (A) the scheme and secondary structure of the dimerization process; (B) the snapshot of the overall kissing complex and the zoomed-in view of the kissing loop region (PDB 2D1B) [Citation183]; and (C) the example snapshots of two extended duplexes which display different configurations of the bulged nucleotides (PDBs 462D and 2D1A) [Citation183,Citation185]. The zoomed-in view on one of the bulged motifs is provided for the second extended duplex. In each panel, nucleotides of the DIS kissing loops (G274 to C279) participating in the recognition process are highlighted in darker colors.](/cms/asset/6f2313c0-748c-49f0-ab31-fae9f68104fe/krnb_a_2289709_f0007_oc.jpg)
In this section, we highlight the structures of different states of the HIV-1 DIS RNA during the dimerization process which have been characterized in the presence and absence of various ligands (). These structures provide us details on several steps of the HIV-1 gRNA dimerization process, but we still lack a detailed description of the key molecular factors which contribute to these processes as well as a detailed description of the full dynamic pathway which links all of these states. Furthermore, while no structural data are available on the recognition mechanism of gRNA by the Gag polyprotein, we highlight the understanding gained using biochemical studies on the assembly, release, and maturation steps.
Table 4. Structural data on the HIV-1 RNA elements participating in the gRNA dimerization. Data similar to are shown for the HIV-1 RNA elements participating in the gRNA dimerization and the following additional abbreviations are used: BM, bulge-out motif; DIS, dimerization initiation site; ED, extended duplex; KL, kissing loop; MD, molecular dynamics simulation; SL, stem-loop. Asterisk (*) indicates structures containing DNA.
Studies on apo DIS RNA
The NMR and X-ray methods have been utilized to resolve the 3D-structures of various configurations of the DIS RNA, specifically of the kissing loop complex [Citation191–194], of the extended duplexes in the absence [Citation184,Citation185,Citation188,Citation205] and in the presence of various metal ions [Citation186,Citation189], and of the bulge-out region [Citation180–182]. The resolved structures of the kissing complex demonstrated the formation of a coaxial stacking of the DIS hairpins (example in ) which was conserved across various HIV-1 subtypes [Citation195], and across RNA chains of varied length [Citation183]. The structures of the kissing loop complex are similar in general, except for the G273 nucleotide (or A273 in some structures). This nucleotide exhibited high flexibility and could be found either in the flipped out [Citation192] or flipped in conformations [Citation183]. This difference may be attributed either to the sequence differences of the stem adjacent to the loop and/or to the sample condition [Citation183].
The resolved structures of the extended duplex demonstrated a well-defined double stranded configuration with the flexibility around the junctions of the loop and the stem of the DIS RNA in vitro () [Citation183,Citation185]. Specifically, the flexibility could be observed at the bulged A272 nucleotide which is projected away from the helix (left; ) [Citation185] or at the bulged purine rich motif between the G284 and G289 nucleotides (right; ) [Citation183]. The longest dimer-structure of the HIV-1 DIS RNA was resolved using the cryo-EM at a 9 Å-resolution and refined using the NMR spectroscopy and molecular dynamics (MD) simulations [Citation190]. The structure revealed the formation of a right-handed superhelical twist and a flipped out configuration of the base in the conserved purine-rich bulge at each end of the duplex, thus confirming previous findings [Citation190]. While the extended duplex structures were resolved in vitro, there is little evidence of the formation of extended duplex conformation in vivo and further testing is necessary [Citation7].
The single molecule FRET assays were used to visualize the dimerization process, revealing that the annealing of the primer could promote the formation of the kissing loop complex [Citation209]. Importantly, the
primer stabilized only the kissing loop complex but not the extended duplex [Citation209]. On the contrary, the formation of the extended duplex was promoted by the NC protein, consistent with the proposed dimerization mechanism of the ordered series of structural rearrangements in gRNA [Citation209]. In a separate study, binding of the NC protein to the SL1 motif was confirmed by the in-gel SHAPE experiments [Citation210]. It was further hypothesized that multiple guanosine residues in the
-UTR could act as binding sites for the Gag polyprotein [Citation210]. Specifically, severe defects in genome packaging were observed upon mutation of multiple guanosines simultaneously, indicating potential importance of these residues in genome packaging [Citation210].
Studies on 
-UTR
Although early studies proposed that only a small region of -UTR containing the SL1 motif was responsible for the gRNA dimerization, more evidence is available to date showing that other elements in
-UTR could contribute to the dimerization process [Citation4,Citation6,Citation7]. Furthermore, it was proposed that the
-UTR could act as a conformational switch of the gRNA dimerization by transitioning between several conformational states [Citation4,Citation6,Citation7]. In one of the proposed RNA-switch models, the
-UTR transitioned between two monomeric states, the long-distance interaction (LDI) state and the branched multiple hairpin (BMH) state [Citation6,Citation211,Citation212]. In the LDI state, the SL1 motif was disrupted and engaged in base-pairing interactions with the polyA stem [Citation6]. The DIS motif in SL1 was hindered from potentially interacting with Gag and thereby promoting the translation process rather than dimerization [Citation6]. In the BMH state, the AUG codon interacted with the U5 motif, forming the U5:AUG interaction with multiple folded hairpins (e.g. SL1) and promoting gRNA dimerization [Citation6]. While the LDI-BMH model seemed appealing and was confirmed by various in vitro studies, the LDI state has not been detected in vivo [Citation59,Citation213,Citation214].
In 2015, a structure of the -UTR, which included PBS, Psi, DIS, and U5:AUG helices, was resolved using NMR to reveal the formation of a tandem (dimeric) three-way junction structure [Citation179]. The overall shape of the structure was determined to be quasi-tetrahedral, similar to the BMH state, with the U5:AUG and Psi-helices forming a plane that was nearly perpendicular to the plane formed by the PBS and DIS helices [Citation179]. The
-UTR adopted the tandem three-way junction configuration in vitro giving rise to another plausible switch model [Citation114,Citation179], but further in vivo evidence is necessary to support these structural models [Citation4,Citation7]. The U5:AUG interaction in the three-way junction configuration was similar to the BMH state, but the base-pairing interactions between two strands were further extended to include residues of the major splice donor site in SL2 [Citation179,Citation213]. Thus, the SL2 motif was proposed to form base-pairing interactions with the PBS RNA from the other gRNA molecule [Citation179]. However, various biochemical studies indicated that the SL2 motif formed a hairpin structure [Citation6,Citation201].
In 2020, the -UTR structure which included TAR, PolyA, PBS, DIS, and Psi RNA helices was resolved using NMR to probe the effect of the number of guanosine residues at the
-end [Citation114]. The structure corresponded to the RNA transcript with an additional guanosine residue at the
-end (G1G), revealing the formation of a dimeric multihairpin RNA conformation similar to the proposed BMH model [Citation114]. The effect of the number of guanosine residues at the
-end was observed to modulate the dimerization process by forcing the transition between mutually exclusive conformations [Citation114]. Specifically, the resolved G1G structure demonstrated sequestration of the
-cap, while simultaneously exposing the sites for the binding of Gag polyprotein [Citation114]. Additionally, the other two models (G2G and G3G) displayed an opposite behaviour by exposing the
-cap and sequestering the Gag binding sites, thereby inhibiting the dimerization process [Citation114]. In a separate study, the significance of the
-cap configuration was further confirmed [Citation215], proposing it as an essential determinant of gRNA packaging in a bipartite mechanism. The mechanism required both the exposure of the
-cap and of the Psi RNA binding sites to the NC protein [Citation215].
Studies on psi RNA elements and NC protein
Selective packaging of the HIV-1 genome during the assembly step was shown to be mediated by the interactions between NC and the packaging signal [Citation215]. Importantly, NC was shown to bind to different elements in the Psi region with similar binding affinities, despite these RNAs having different binding surfaces [Citation198,Citation199]. Tight binding of NC to Psi RNA was mediated by various hydrogen bonding, electrostatic, and hydrophobic interactions, as well as by the formation of specific intramolecular interactions within the NC domain induced by the binding to RNA [Citation198,Citation199]. Further studies of Gag specificity proposed that the basic amino acid sidechains in the NC domain of Gag contributed the most to gRNA binding [Citation216].
The structures of the -UTR and Psi RNA bound to the NC protein in conjunction with mutational experiments highlighted the potential role of multiple guanosines as binding sites for Gag or NC proteins [Citation114,Citation179]. The discrimination between Psi RNA and non-Psi RNA by the Gag polyprotein was further investigated using the fluorescence correlation spectroscopy [Citation217]. It was confirmed that Gag had a high specificity for Psi-containing RNA but it was obscured in physiological salt conditions by the introduction of the non-specific electrostatic interactions [Citation217]. These findings complemented prior results on the RSV which showed that the RSV Gag polyprotein effectively discriminated RSV Psi RNA from non-Psi RNA, thus showing that these interactions were conserved among a subset of retroviruses [Citation218].
Studies on DIS RNA and therapeutic agents
Furthermore, DIS RNA has been proposed as a potential target for the anti-HIV-1 drugs. Specifically, it was demonstrated that the HIV-1 DIS has structural similarities with the bacterial ribosomal A site and therefore could be targeted with aminoglycosides [Citation187,Citation196]. Binding of these compounds was shown to stabilize the kissing-loop complex or the extended duplex conformation by mediating contacts between two DIS RNA strands [Citation187,Citation196]. Alternatively, the dimerization and packaging processes could be perturbed by changes in the RNA genome sequence which lied downstream to the Psi region [Citation219,Citation220]. The sequence changes were proposed to induce misfolding of the Psi packaging signal in vitro and disrupt the RNA trafficking within cells [Citation219,Citation220].
Biochemical studies of gRNA-Gag interactions during packaging
While it was known that gRNA must be dimeric and present at the host-cell membrane in order to get packaged into a virion, the mechanism and the location of the gRNA dimerization is a challenging process to capture in vivo primarily due to the presence of low and transient amounts of unstable gRNA dimers [Citation6]. Based on the data from high-performance RNA imaging approaches, it was proposed that the HIV-1 gRNA dimerization first occurred in the cytosol of cells from where the dimeric gRNA could be transported to the plasma membrane [Citation221]. However, in a different study it was proposed that HIV-1 gRNA could only dimerize at the plasma membrane and not in the cytoplasm [Citation222]. While proposing different locations in the cell for dimerization to occur, both studies demonstrated that the Gag polyprotein played an important role in the gRNA dimerization process [Citation221,Citation222]. Furthermore, an artificial cell-free system was created by combining the plasma membrane, viral RNAs, and Gag polyprotein to reveal that Gag polyprotein needed to be attached to the plasma membrane to correctly package gRNA [Citation223]. These results were confirmed by the fluorescence-based experiments which showed that efficient genome packaging necessitated multimerization of Gag polyprotein in addition to the Gag attachment to the plasma membrane [Citation224]. Following the outlined mechanism, gRNA was proposed to act as a scaffold for Gag assembly while maintaining the stability of the subsequent gRNA/Gag complex [Citation225]. This mechanism was further confirmed by the atomic force microscopy (AFM) experiments [Citation226].
Multiple binding sites of Gag polyprotein within the packaging signal were identified using a stoichiometry analysis and the Kd values were estimated for each of the binding events [Citation227]. It was further confirmed that the internal loop of SL1 was the primary recognition site for Gag among various spliced and unspliced RNAs [Citation227]. Furthermore, Gag polyprotein and its NC-containing maturation products were shown to bind at different gRNA sites with distinct contributions to the gRNA structural maturation [Citation228]. Isothermal titration calorimetry (ITC) was used to show that major interactions between HIV-1 gRNA and Gag formed during virion assembly were energetically favourable [Citation229]. Furthermore, it was observed that the Gag binding with immature HIV A-rich RNA motifs had an increased enthalpy change in comparison to the Gag binding with the GU-containing RNA motif [Citation229]. Thus, based on the thermodynamic data, an additional regulation mechanism of the viral assembly was proposed to involve interactions between Gag and the A-rich RNA which could assist Gag in the packaging of the RNA genome in the immature virion [Citation229]. However, additional structural data are necessary to establish the role of these interactions in genome packaging. The recognition mechanism of Gag polyprotein by gRNA could be potentially explained by computational structural models of the SL1 motif which showed increased bubble shape of the hairpin loop of the SL1 motif, thus providing a binding site for Gag [Citation230].
Biochemical studies of gRNA during release and maturation
Following the release, the immature HIV-1 virion undergoes structural rearrangements termed as maturation (steps 11–12; ). During this step, CA proteins assemble in a mature capsid core which encapsulates RT, IN, and two copies of gRNA which are coated by the NC proteins [Citation231]. The crosslinking-immunoprecipitation sequencing (CLIP-seq) approach showed that HIV-1 IN bound to certain viral RNA elements in HIV-1 gRNA with high specificity [Citation232]. It was further demonstrated that a number of mutations in HIV-1 IN, termed as class II substitutions, could only disrupt the packaging function of HIV-1 IN. In that regard, these class II substitutions, which mostly included mutations of positively charged amino acid sidechains (e.g. R262, R263, R269, K273), led to the formation of eccentric viruses [Citation233]. However, the effect of these substitutions could be compensated by mutations of nearby negatively charged aspartic acid sidechains (e.g. D256, D278, and D279) to positively charged amino acids, thus restoring the ability of the HIV-1 IN to bind gRNA [Citation234]. Binding of NC and IN proteins to the HIV-1 -UTR was proposed to be orthogonal: while the NC protein bound to the UG-rich region proximal to the U5:AUG stem and the Psi RNA in the RNA genome, the IN protein bound the apical loop of TAR RNA and the polyA hairpin [Citation235]. Therefore, additional structural data which comprehensively describe the interactions formed between HIV-1 gRNA and IN or NC proteins are necessary to fully understand the maturation process of HIV-1.
HIV-1 TAR RNA as a model system in experimental approaches
Brief overview of structural techniques used to probe RNA dynamics
The diversity of RNA functions is rooted in its ability to undergo conformational changes which could lead to the formation of complex tertiary folds [Citation236]. These conformational changes could be induced by various factors, including the application of an external stimuli, by changes in physiological conditions, or by binding of various ligands [Citation237,Citation238]. The importance of dynamics in the function of RNA has led to characterization of the conformational landscape of RNA and the contributing intrinsic properties at the local base-pair level and at the global tertiary level [Citation239–241]. Since it is challenging to fully characterize all known RNA molecules and their RNA/ligand complexes, model systems combining well-defined RNA structural features and broad conformational landscapes are required [Citation241].
TAR RNA serves as a widely-used model system for probing local and global conformational transitions in RNA, for characterizing the interactions of RNA with various ligands (e.g. small-molecules, peptides, and proteins, etc.), and for testing the applicability of different experimental (e.g. NMR) and computational approaches (e.g. inhibitor screening) [Citation237,Citation242,Citation243]. TAR RNA was selected as a model system due to its small structure (29 nucleotides) and the presence of a flexible three-nucleotide bulge motif which connects two helices [Citation243,Citation244]. The intrinsic flexibility of TAR RNA leads to an ensemble of conformations which can recognize and accommodate small-molecules [Citation88–91], peptides [Citation86,Citation87,Citation92–96], proteins [Citation97–99,Citation102], and metal ions [Citation245]. Various experimental methods have been developed to characterize structures and dynamics in RNA.
Among different methods of structural characterization, NMR is the most common experimental technique for characterizing RNA dynamics at the atomic level [Citation237,Citation243]. It is of note that the spectroscopic and hydrodynamic properties of nucleic acids differ from those of proteins and therefore a reoptimization of protein-specific NMR methods is often required [Citation38]. Various NMR techniques rely on different measurements, including chemical shifts, spin relaxation, and residual dipolar couplings (RDCs) to provide a comprehensive description of an RNA system [Citation38,Citation246]. One significant advantage of the solution-state NMR spectroscopy is its ability in resolving the flexibility inherent to RNA molecules and their low-populated conformational states [Citation38,Citation246,Citation247]. However, one of the main disadvantages of solution-state NMR is resolving relatively large RNA structures due to the spectral overlap which arises with increased RNA size and molecular weight [Citation246]. This disadvantage can be circumvented by applying solid-state NMR which can generate structural information without molecular weight or size limitations [Citation246].
Alternatively, X-ray crystallography and cryo-EM techniques can be used to probe relatively large RNA or RNA/protein structures [Citation40,Citation41]. While both techniques have been extensively applied and refined on various protein systems, their applications to RNA have been relatively limited [Citation40,Citation41]. This could be due to the inherent flexibility of RNA resulting in multiple alternative conformations and functional states, which are challenging to resolve using X-ray crystallography and cryo-EM [Citation41]. Specifically, the main disadvantages of determining RNA structures using X-ray crystallography are low yields of properly folded RNA, difficulties in making crystals, and determining the intensities of diffracted waves [Citation40]. The main challenges in applying cryo-EM to RNA molecules include the lack of approaches for obtaining properly folded RNA molecules, flexibility of RNA, and the majority of RNA molecules being relatively small [Citation41].
While NMR is still the preferred method for characterizing RNA structures, X-ray crystallography and cryo-EM methods are continuing to improve, which thereby enables the determination of large RNA systems. This is done by incorporation of novel techniques and tools as described elsewhere [Citation38,Citation40,Citation41,Citation246]. It is likely that in the future combined approaches using the aforementioned structure-determination techniques and computer simulations could enhance our understanding of the RNA biophysics. Next, we provide an overview of the utility of TAR RNA as a model system in experimental approaches.
TAR RNA as a model system for probing conformational ensembles in RNA
The characterization of RNA conformational ensembles is crucial for understanding its biological functions and for developing novel therapeutic approaches. For example, new NMR methods were developed for characterizing short-lived and low-abundance conformations of RNA which were defined as the excited states (ESs) [Citation248,Citation249]. The ability to identify ESs was critical for establishing a comprehensive map of RNA structures since ESs were shown to act as conformational switches [Citation250] as well as intermediate states during RNA folding [Citation251]. NMR was further applied to study the stabilization of ESs with respect to the ground state due to the -O-Methyl (Nm) modification [Citation252]. Specifically, introducing the Nm modification in the TAR RNA nucleotides C24, U25, and A35 increased the abundance and the lifetimes of two ESs [Citation252].
The X-ray scattering interferometry (XSI) technique was also proposed as an alternative technique for determining RNA conformational ensembles [Citation253]. The resulting XSI-based models of TAR RNA were shown to resemble the models independently derived from the NMR data [Citation253]. Another technique specially designed to study folding/unfolding pathways of TAR RNA was a high-precision SMFS [Citation254]. The data generated from the SMFS experiments were comparable to the more commonly used AFM experiments while circumventing the technical barriers in other AFM-based methods applied to RNA [Citation254]. Thus, a number of experimental techniques have been designed for characterizing RNA conformational ensembles.
TAR RNA as a model system for characterizing HJH motifs
The bulge and internal loop motifs, which constitute the helix-junction-helix (HJH) motifs, are flexible building blocks in RNA, often joining the helical domains [Citation255,Citation256]. The HJH motifs are considered as excellent model systems for elucidating RNA conformational dynamics [Citation255,Citation256]. A combination of different NMR techniques was used to probe the effect of shortening (3 to 2 nucleotides) a bulge motif in TAR RNA on the motional modes of the HJH-containing RNA [Citation242]. Subsequently, the structure was shown to transition from a bent flexible conformation towards a coaxially-stacked conformation in which the bulge nucleotides were in a flipped-out configuration [Citation242]. Furthermore, it was shown that increasing of the length of bulge motifs (above three nucleotides) resulted in largely unstacked conformations of the TAR RNA helices [Citation257]. Based on these experiments, Mg2+ ions were proposed to stabilize a coaxially stacked conformation irrespective of the length of the bulge [Citation242,Citation257].
Alternatively, the effect of varying the length of a given residue sidechain in Tat-derived peptides on their binding to TAR RNA was probed using fluorescence experiments [Citation258]. It was revealed that increasing in the length of the residue sidechains improved the binding affinities of the peptides towards TAR RNA but reduced the degree of compactness in RNA at the bulge level [Citation258]. Two techniques (MaxOcc and MaxOR) were used to analyse the NMR RDC and MD datasets of TAR RNA [Citation259]. The resulting analysis confirmed the existence of two equilibrium states along with several ligand-bound conformations formed by the HJH motifs in TAR RNA [Citation259].
TAR RNA as a source of microRNAs
TAR RNA has also been proposed as a source for microRNAs (miRNAs), which are short RNA structures composed of 21 nucleotides. One of the functions of miRNAs is targeting mRNAs for cleavage or translational repression [Citation260]. However, details on the creation of miRNAs without disruption of the virus replication process remained elusive until it was demonstrated that the
end of TAR RNA could be processed into an miRNA-like small RNA [Citation261]. This miRNA was shown to repress the expression of mRNAs while also avoiding cleavage of the RNA genome [Citation261]. Additional details on the dynamics and conformational transitions in TAR RNA are provided later where TAR RNA is discussed in the context of being a robust model system for characterizing RNA dynamics.
TAR RNA as a model system for developing RNA targeted therapeutics
The inherent flexibility of TAR RNA, its ability to form an ensemble of conformations with transient binding pockets [Citation237,Citation243], and the presence of various secondary structure motifs (e.g. bulges, stem-loops, helices, etc.) have established TAR RNA as a model system for developing RNA targeted therapeutics. For example, the conformational ensemble of TAR RNA was used to test the applicability of a combination of NMR and high-performance virtual screening methods [Citation237]. These findings demonstrated that the experimentally resolved RNA structural ensembles complemented with MD data could improve and enrich the existing compound libraries [Citation237]. TAR RNA and RRE RNA were also used to evaluate the effects of three compounds from a novel class of RNA binders which non-specifically targeted stem-loops, bulges, and internal loops in RNA molecules [Citation262]. These compounds were reported to bind the HIV-1 RNA molecules, by potentially forming hydrogen-bonding and electrostatic interactions with the RNA molecules [Citation262].
TAR RNA was also used as a target model system for identifying key secondary structure elements which contribute to the recognition of ligands by RNA molecules [Citation263,Citation264]. By using the principle component analysis (PCA), it was revealed that the shapes of small molecules were essential to their recognition by RNA [Citation263,Citation264]. TAR RNA was also used for developing a novel RNA-binding scaffold based on the amiloride lead [Citation265]. These compounds were shown to predominantly bind TAR RNA at the apical loop and the bulge motifs [Citation265]. The amiloride-based scaffold was further designed to bind other HIV-1 targets, including RRE RNA, HIV-1 exonic splicing silencer (ESS), and HIV-2 TAR RNA [Citation266].
Computational studies
Brief overview of molecular simulations of HIV-1 RNA
Experimental methods such as the NMR spectroscopy, cryo-EM, and X-ray crystallography have demonstrated success in resolving the tertiary structures, base pairing interactions, and conformational ensembles in RNA [Citation237,Citation243,Citation299]. However, these high-resolution methods are often limited in describing fully resolved conformational dynamics due to a large number of parameters to be probed, time intensiveness, need for pure samples, and the difficulties in obtaining suitable crystals [Citation179,Citation299,Citation300]. Therefore, computational techniques bridge these gaps in our understanding of dynamics and interactions in RNA [Citation299]. Specifically, all-atom molecular dynamics (MD) simulations are a useful tool for probing biomolecular dynamics [Citation299]. These simulations predict the time-evolution of the motions in all atoms as they interact via an interatomic potential/force-field [Citation301–303].
One of the key factors determining the accuracy of MD simulations is the parameters defining the force-field, which are often derived using quantum mechanical calculations [Citation299,Citation303]. For RNA simulations, the CHARMM and AMBER family of force-fields are most commonly used and have been refined, optimized, and successfully used in various simulation studies [Citation299,Citation303]. Recent advances in the atomistic force-fields for RNA molecules and the development of enhanced sampling methods have allowed for more extensive and robust characterization of the structure-dynamics-function relationships for various RNA molecules [Citation299]. For example, a number of simulation studies have been carried out for HIV-1 RNA molecules and their interactions with the ligands ( [Citation106,Citation111,Citation113,Citation147,Citation151,Citation283–285,Citation292,Citation293,Citation297]. However, most of these studies employed conventional MD simulations, omitting the use of enhanced sampling approaches (). These approaches could be used to extend the sampling of the conformational space accessible to RNA molecules by probing those biophysical events that are otherwise challenging to characterize via conventional simulation methods [Citation299,Citation304,Citation305]. We now provide a brief overview of computational studies conducted on the HIV-1 RNA molecules ().
Table 5. A summary of simulation studies on the HIV-1 RNA. For each simulation study, listed is the simulated HIV-1 RNA element, corresponding simulation conditions, and the force-field used in RNA simulations. The cumulative simulation time-scale, the temperature, and simulation methods are also listed for each study. The RNA force-fields summarized are CHARMM27 [Citation267], CHARMM36 [Citation268], Cornell et al. [Citation269], parm98 [Citation270], parmbsc0 [Citation271], ff99OL3 [Citation271–273], and RNA.ROC [Citation274]. The missing simulation parameters or force-field details in the table is due to the absence of such information in some simulation studies [Citation258,Citation275–278]. The following abbreviations are used: CG, coarse-grained; DFT, density functional theory; FEP, free energy perturbation; KL, kissing loop; MD, molecular dynamics; MM-GBSA, molecular mechanics with generalized born and surface area solvation; PBE, Poisson–Boltzmann equation; QM, quantum mechanics-based reparameterization of the AMBER force field; RAM, replica-averaged metadynamics; SL1, stem loop 1; SMD, steered molecular dynamics. Furthermore, RNA/DNA indicates the structure containing RNA/DNA hybrid.
Role of base flipping in HIV-1 RNA
MD simulations have been applied to a variety of HIV-1 RNA systems, ranging from the kissing loop motifs to TAR RNA. While the majority of HIV-1 RNA simulation studies have focused on TAR RNA, several simulation studies have been directed on the HIV-1 RNA molecules which participate in the dimerization process. Specifically, a 10 ns all-atom MD simulation of the stem loop 1 (SL1) RNA was conducted using the AMBER98 (parm98) RNA force-field [Citation197]. It was observed that the flipping of bases in the loop motif resulted in an increase of the flexibility of the SL1 motif [Citation197]. In another study, all-atom MD simulations were conducted of the six-base-pair DIS RNA on a 5 ns timescale as well as of the two-base-pair kissing complex on a
16 ns timescale with the AMBER force-field [Citation279]. It was proposed that the kissing loop inner pocket was a major ion binding site and the unpaired bases could adopt a bulged-out configuration [Citation279].
Importantly, these observations were later confirmed by structural studies [Citation195]. Furthermore, a total of 800 ns long all-atom MD simulations with the AMBER and CHARMM force-fields were conducted to characterize the dynamics of the flanking bases in the DIS RNA kissing loops [Citation280]. While these simulations confirmed the ability of the unpaired bases to form a bulged-out state, these simulations also demonstrated the formation of the bulged-in states [Citation280]. Finally, a set of all-atom MD simulations (with a cumulative simulation time of 25 s) were conducted for the HIV-1 RNA kissing complexes with the AMBER ff99OL3 RNA force-field [Citation281]. The flipped inward configuration of the unpaired bases in the kissing complexes was again observed during MD simulations [Citation281]. This event could be a force-field artefact or a biochemically relevant conformation and additional testing is necessary [Citation281].
In a separate study, we applied non-equilibrium SMD simulations to characterize the binding/unbinding process of a small-molecule with inhibitory properties (acetylpromazine) from TAR RNA [Citation297]. We performed 100 non-equilibrium SMD simulations with the AMBER ff99OL3 RNA force-field [Citation272,Citation273] and revealed that rare conformational transitions in the bulge nucleobases were responsible for ligand binding [Citation297]. Specifically, we observed the inward flipping of the C24 nucleotide which formed base-pairing interactions with the A22 and U40 nucleotides [Citation297]. We further provided a detailed mechanistic description of the underlying conformational transitions using various conformational metrics [Citation297]. Collectively, these studies highlighted the importance of base flipping as a key biophysical event in HIV-1 RNA.
Conformational heterogeneity in recognition of ligands by HIV-1 RNA
MD simulations have also been applied to probe the interactions between TAR RNA and various ligands (). For example, a study on the interactions between argininamide and TAR RNA was one of the earliest simulation studies on HIV-1 RNA [Citation283]. Several 1 ns long MD trajectories were generated with/without argininamide to reveal that the ligand-bound TAR RNA structure was more rigid than the unliganded structure. This was due to the formation of a U27-A27-U38 base triple, consistent with the experimental data [Citation283]. The free energy analysis in the form of the Poisson – Boltzmann approximation was conducted to emphasize the importance of the stacking interactions between the ligand argininamide and RNA [Citation283]. A similar simulation study was then performed on the bovine immunodeficiency virus (BIV) Tat/TAR complex to observe structural transitions similar to the argininamide/TAR complex [Citation284]. In another study, a small peptide (L)Lys-(D)Lys-(L)Asn (KkN) with inhibitory properties was docked to TAR RNA and multiple 20 ns MD simulations were conducted for various docked poses with the AMBER98 RNA force-field [Citation285]. It was proposed that the KkN peptide potentially bound TAR RNA at the minor groove via the stacking, hydrogen-bonding, and electrostatic interactions between the peptide and TAR RNA [Citation285].
The design of peptides mimicking Tat protein and targeting TAR RNA has gained attention since the discovery of the ARM in Tat protein [Citation86,Citation92,Citation94,Citation95]. The arginine and lysine residues in the ARM of Tat were identified as critical for recognizing TAR RNA via hydrogen-bonding interactions [Citation294,Citation295]. MD simulations were applied to determine the changes in the TAR RNA structure and the ion distribution around it on binding of the L-22 peptide [Citation111]. It was demonstrated that the ions were displaced from the L-22 peptide and TAR RNA on complex formation [Citation111]. These findings were consistent with a previous simulation study where multiple 20 ns MD simulations of TAR RNA were performed in different ion solutions [Citation289].
Non-equilibrium simulations with the AMBER ff99SB-ILDN parmbc0 RNA force-field were also used to characterize the (un)binding process of a cyclic peptide (L-22) to TAR RNA [Citation292]. The net electrostatic interactions between the L-22 peptide and TAR RNA was used as a collective variable to perform the (un)binding processes [Citation292]. The presented methodology with the electrostatic term as the collective variable correctly reproduced the experimentally observed binding pose of the L-22 peptide [Citation292]. Additionally, a second binding pose was proposed based on these simulations [Citation292]. The interactions of two cyclic peptides, L-22 and KP-Z-41, with TAR RNA were also characterized using MD simulations coupled with the MM-GBSA calculations [Citation293]. Based on these calculations, it was concluded that the electrostatic interactions contributed more to the KP-Z-41 complex formation in comparison to the L-22 complex formation [Citation293]. The MM-GBSA thermodynamic analysis was also performed to reveal TAR-RNA binding effect of varying the length of a given residue sidechain in the peptides derived from the Tat protein [Citation258]. Specifically, the last 2 ns of 20 ns long MD simulations of several peptide/TAR complexes were extracted to suggest that increasing the length of a residue sidechain improved the binding affinities of the peptides but reduced the degree of compactness in RNA [Citation258].
We reported all-atom s-long MD simulations of TAR RNA in the apo and bound configurations with a diverse set of ligands ranging from small-molecules to cyclic peptides which were initiated from 27 distinct experimentally observed states () [Citation106]. In these simulations, we employed the AMBER ff99OL3 RNA force-field [Citation272,Citation273] and showed the conformational heterogeneity in TAR RNA which facilitates recognition of a diverse set of ligands [Citation106]. Furthermore, we observed that the initially-distinct unliganded structures of TAR RNA converged to similar conformations supporting the hypothesis that TAR RNA adopted unique conformations for binding of each ligand [Citation106].
The effect of monovalent ions (Na+ and K+) on the stability of TAR RNA was characterized using the AMBER parmbsc0 RNA force-field with all-atom SMD simulations [Citation298]. Specifically, a correlation between the RNA stability and the ion size was established where smaller sodium ions preferentially bound at the negatively charged phosphate groups while larger potassium ions were observed to bind at the major groove of TAR RNA [Citation298]. Therefore, smaller sodium ions could have access to larger surface area which led to reduced electrostatic repulsion in comparison to potassium ions. The authors hypothesized that these conclusions could be extended to divalent metal cations but further testing is required [Citation298].
Salt-bridging interactions in recognition of HIV-1 RNA by helical peptides
The RRE RNA molecule is considered as a good model system to probe the mechanisms of recognition of RNA by the helical peptides () [Citation306]. All-atom MD simulations were applied to characterize the interactions formed between two helical peptides, a Rev peptide and a lab-evolved peptide (RSG-1.2), and RRE RNA [Citation282]. It was proposed that additional salt-bridging interactions contributed to a tighter binding of the RSG-1.2 peptide to RRE RNA in comparison to the Rev peptide [Citation282]. The water molecules were also proposed to play a role in mediating the interactions between the peptides and the RRE RNA [Citation282]. Kumar and Vashisth further applied free energy perturbation (FEP) calculations to identify and energetically characterize critical interactions between the peptides and RRE RNA [Citation147]. Specifically, 50
s of free energy calculations and all-atom MD simulations with the CHARMM36 RNA force-field were conducted [Citation147]. It was observed that the majority of interactions between the Rev peptide and the RRE RNA involved the sidechains of amino-acid residues and the sugar – phosphate backbone of RRE RNA, suggesting shape-selectivity as a critical factor [Citation147]. On the contrary, the RSG-1.2 peptide employed a network of hydrogen-bonding interactions, salt-bridging interactions, and hydrophobic interactions for recognizing RRE RNA, which could be a contributing factor towards its higher binding affinity and specificity in comparison to the Rev peptide [Citation147].
We also applied non-equilibrium SMD simulations for resolving the (un)binding mechanism of the RSG-1.2 peptide from the RRE RNA [Citation151]. We generated a total of 3.9 s simulation data to reveal the mechanistic details of several unbinding pathways of the RSG-1.2 peptide and established a preferred pathway by comparing the free energy profiles along various pathways [Citation151]. Furthermore, we identified the R8, R14, and R15 amino acids as important for the recognition of the peptide by RRE RNA and established the role of the water molecules in the binding pocket [Citation151]. Collectively, these studies highlighted the importance of salt-bridging interactions, hydrogen-bonding interactions, and the role of water molecules in recognition of the helical peptides by RNA.
The HIV-1 nucleocapsid protein (NCp7) is a zinc-binding protein which promotes annealing of viral DNA and RNA. NCp7 was shown to adopt a helical shape when bound to the PBS vDNA, the SL2, and the SL3 domains in Psi region [Citation52,Citation198,Citation199]. MD simulations of the NCp7 bound to the PBS DNA and to the SL3 domain were conducted using the reparameterized AMBER force-field [Citation287]. These 10 ns long MD simulations confirmed that the interactions between the NCp7 and the viral genome were governed by the electrostatic interactions [Citation287]. Furthermore, MD simulations revealed the formation of a network of hydrophobic and hydrogen-bonding interactions which stabilized the NCp7/DNA and the NCp7/RNA complexes [Citation287].
HIV-1 RNA and proteins
Simulation efforts have also been directed towards characterizing the interactions of the HIV-1 RNA with proteins. The interactions between the ribonuclease H (RNase H) protein of the RTIC with its DNA – RNA hybrid substrate were studied using all-atom MD simulations conducted with the CHARMM27 RNA force-field [Citation275]. Independent simulations were conducted for the full reverse transcription complex, the free protein domain, and the RNA/DNA substrate [Citation275]. It was proposed that the catalytic site of the RTIC was preorganized in the protein domain prior to substrate binding [Citation275]. Additionally, the geometrical changes in the RNA/DNA helix were induced through the interactions with the protein upon completion of the binding step [Citation275]. The RTIC with the RNA/DNA substrate was also studied using all-atom MD simulations with the AMBER ff99OL3 RNA force-field combined with the biochemical studies [Citation276]. The results showed that the RNA/DNA substrate could interact simultaneously with both of the catalytic sites in RTIC [Citation276]. Furthermore, a set of all-atom MD simulations coupled with the single-molecule assays revealed the existence of a specific conformation of the HIV-1 RTIC that could modulate the enzymatic activity of the RNase H [Citation277].
The TAR RNA/TBP complex was another HIV-1 RNA/protein complex probed using simulation techniques [Citation113]. Specifically, Kumar and Vashisth probed the interactions formed at the binding site between all TBPs and TAR RNA (shown in ) using the FEP method and the CHARMM36 RNA force-field [Citation113]. Using a cumulative dataset of 16.4 s, the underlying energetics of the RNA/protein interactions was quantified and the contributions of individual arginine amino acids in the
2-
3 loop during their interactions with TAR RNA were determined [Citation113].
Combined NMR/MD approaches
All-atom MD simulations of TAR RNA were performed and compared against the RDC parameters derived from the NMR data in several studies [Citation95,Citation259,Citation278,Citation286,Citation288,Citation290,Citation291]. By using a combination of NMR and s-long MD simulations, it was possible to obtain and validate atomic resolution ensembles of TAR RNA conformations [Citation259,Citation278,Citation290,Citation291]. MD simulations conducted using both CHARMM and AMBER force-fields were reported to yield moderate-to-excellent agreement with the experimental data [Citation278,Citation290,Citation291]. These datasets further validated that the interhelical dynamics in TAR RNA occurred at the ns-
s timescale [Citation278,Citation290,Citation291]. The observed discrepancies between NMR and MD data were proposed to occur due to the motion of the most flexible residues in TAR RNA, specifically the bulge motif residues [Citation278,Citation290]. The RDC data from the NMR spectroscopy was also combined with the replica-averaged metadynamics simulations to establish several low-population intermediate structures of the L-22 peptide bound to TAR RNA [Citation95]. While combined NMR/MD approaches have demonstrated their usefulness in resolving RNA structures and expanding conformational ensembles of RNA, a potential limitation of these approaches could be rooted in the limited resolution of the experimental data which could lead to bias in the selection of the structures within an ensemble of conformations [Citation290,Citation291,Citation299].
HIV-1 RNA as a model system for predictive modeling
TAR RNA was also used as a model system for developing various modelling approaches to predict the folding/unfolding pathways, binding pockets, and secondary structure elements. A helix-based structure network approach was developed to model the RNA folding kinetics at the 2D structural level and was tested on the HIV-1 TAR RNA [Citation307]. The resulting predictions for TAR RNA were shown to agree with the experimental results and further revealed two parallel folding pathways [Citation307]. Another web-based platform was developed to predict the RNA folding using the high throughput chemical probing data [Citation308]. This platform was used to predict the folded structures from the HIV-1 and SARS-CoV-2 genomes [Citation308]. A method to detect potential ligand binding cavities based on machine learning classifiers was developed with TAR RNA as a model system to test this methodology [Citation309]. This approach demonstrated the ability to discriminate between cavities that could accommodate ligands and decoys. It also revealed several TAR RNA conformers which corresponded to the experimentally observed TAR structures [Citation309].
Coarse-grained simulations of HIV-1 RNA
Coarse-grained modelling is a commonly utilized approach in which a biomolecular system is represented by a reduced number of degrees of freedom in comparison with the all-atom representation as used in MD simulations [Citation310]. At the expense of these reduced degrees of freedom, a coarse-grained model can achieve higher simulation timescales and larger system size in comparison to all-atom MD simulations [Citation310]. RNA is represented as a chain of nucleotides, where each nucleotide is reduced to a specific number of beads which is smaller than the total number of atoms in a nucleotide. The coarse-grained modelling with implicit salt was applied to predict the 3D structure, stability, and the unfolding pathway for the HIV-1 TAR RNA at various ionic concentrations [Citation296]. In the coarse-grained model of RNA each nucleotide was represented as three beads corresponding to the phosphate group, the sugar ring, and the nitrogenous base [Citation296]. Based on these results, it was proposed that the ion concentration and the coaxial stacking were the key contributors to the unfolding process of TAR RNA which transitioned between different states during the unfolding process [Citation296]. In a different study, a set of coarse-grained computational models was used to test several hypotheses with regard to the initial configuration of the genomic RNA and the HIV-1 integrase [Citation311]. These models were able to capture the relative distribution of gRNA induced by the integrase in the immature virion and showed that the exclusion of the NC protein from the simulation system resulted in the asymmetric conformation of the dimerized UTR [Citation311].
Conclusions and perspectives
The replication cycle of HIV-1 involves a series of critical steps and processes through which the virus integrates into the host-cell and exploits its machinery to further generate infectious virus particles. The HIV-1 RNA elements have a significant role across various steps of the replication cycle, including reverse transcription, transcription, nuclear export, translation, and genome packaging. Significant progress in understanding the structures, dynamics, and functions of the HIV-1 RNA has been made since the first crystal structure of HIV-1 TAR RNA was resolved in 1995. In this review, we summarized the structural and computational studies on the crucial HIV-1 RNA elements which participate in various steps of the retroviral replication cycle. We further complemented details on these studies with the available biochemical data.
Although several experimentally resolved structures of the HIV-1 RNA are available, most of these structures were determined for a limited number of RNA molecules, e.g. for TAR RNA, Psi RNA, PBS RNA, and RRE RNA. Therefore, continuous effort in the field of structural biology, which already has a significant impact on HIV-1 research, is necessary to advance the field even further. Another important direction in the HIV-1 research is the emerging role of epitranscriptomics or post-transcriptional modifications. Specifically, it is crucial to characterize the mechanisms by which these modifications are incorporated in HIV-1 RNA by viral or host-cell enzymes. Furthermore, the effect of these modifications on the expression of viral genes and other viral processes is not fully understood. The knowledge about post-transcriptional modifications, as well as about the HIV-1 RNA in general, may assist in developing novel retroviral inhibitors.
Other important research topics for future work include: (1) a complete mechanistic description of the reverse transcription process and the role of stop-codons in this process; (2) a description of the HIV-1 translation, including structural data; (3) a detailed knowledge of the gRNA dimerization and gRNA packaging for further assembly of the HIV-1 particles; (4) characterization of interactions between the HIV-1 integrase and gRNA during the maturation process; and (5) development of novel inhibitors targeting various HIV-1 RNA elements. Computational methods which have been validated multiple times against the HIV-1 experimental data should serve as a useful tool to provide additional insights on the biophysical processes at the atomic level and augment the experimental data. The advances in the RNA force-field, the development of novel methodologies, and the growth of computational power will facilitate future research on further enhancing a structure-level knowledge of various steps where the HIV-1 RNA participates. However, computational methods usually rely on the input of structural data for conducting simulations and for validating the output. Therefore, combined efforts from the experimental and computational fields are necessary to address the mechanistic gaps in our understanding of the HIV-1 viral replication cycle.
Acronyms
ACB | = | Anticodon-binding domain |
AFM | = | Atomic force microscopy |
AIDS | = | Acquired immunodeficiency syndrome |
AMBER | = | Assisted Model Building with Energy Refinement |
ARL | = | A-rich loop |
ARM | = | Arginine rich motif |
ART | = | Antiretroviral therapy |
BMH | = | Branched multiple hairpin state |
CA | = | Capsid |
CDK9 | = | Cyclin-dependent kinase 9 |
CG | = | Coarse-grained |
CHARMM | = | Chemistry at Harvard Macromolecular Mechanics |
CLIP-seq | = | Crosslinking-immunoprecipitation sequencing |
CRISPR | = | Clustered regularly interspaced short palindromic repeats |
cryo-EM | = | Cryo-electron microscopy |
DFT | = | Density functional theory |
DIS | = | Dimerization initiation site |
DNA | = | Deoxyribonucleic acid |
dsRNA | = | Double-stranded ribonucleic acid |
DENV | = | Dengue Virus |
Env | = | Envelope-glycoprotein |
ES | = | Excited state |
ESS | = | Exonic splicing silencer |
gRNA | = | Genomic ribonucleic acid |
FEP | = | Free energy perturbation |
FSS | = | Frameshift stimulating structure |
Gag | = | Group-specific antigen |
HIV-1 | = | Type 1 Human Immunodeficiency Virus |
HJH | = | Helix-junction-helix |
hLysRS | = | Human lysyl-tRNA synthetase |
HCV | = | Hepatitis C Virus |
IN | = | Integrase |
IRES | = | Internal ribosome entry site |
ITC | = | Isothermal titration calorimetry |
lncRNA | = | Long non-coding ribonucleic acid |
LDI | = | Long-distance interaction state |
LTR | = | Long terminal repeat |
MA | = | Matrix |
MD | = | Molecular dynamics |
miRNA | = | Micro ribonucleic acid |
MM-GBSA | = | Molecular mechanics with generalized Born and surface area solvation |
mRNA | = | Messenger ribonucleic acid |
NC | = | Nucleocapsid |
NCp7 | = | Nucleocapsid protein 7 |
NF45 | = | Nuclear factor 45 |
NF90 | = | Nuclear factor 90 |
NMR | = | Nuclear magnetic resonance |
NNRTI | = | Non-nucleoside reverse transcriptase inhibitor |
ORF | = | Open reading frame |
P-TEFb | = | Positive transcript elongation factor b |
PAF-1 | = | Polymerase-associated factor 1 |
PAS | = | Primer activation site |
PBE | = | Poisson-Boltzmann equation |
PBS | = | Primer binding site |
PCA | = | Principle component analysis |
PDB | = | Protein Data Bank |
PRF | = | Programmed ribosomal frameshift |
Psi | = | Packaging signal |
RAM | = | Replica-averaged metadynamics |
RDC | = | Residual dipolar coupling |
Rev | = | Regulator of expression of virion |
RNA | = | Ribonucleic acid |
RNase H | = | Ribonuclease H |
RT | = | Reverse transcriptase |
RTIC | = | Reverse transcription initiation complex |
RRE | = | Rev response element |
RSV | = | Rous sarcoma virus |
SEC | = | Super elongation complex |
SHAPE | = |
|
SL1 | = | Stem-loop 1 |
SMD | = | Steered molecular dynamics |
SMFS | = | Single-molecule force spectroscopy |
ssRNA-RT | = | Positive sense single-stranded ribonucleic acid with deoxyribonucleic acid intermediates |
ssDNA | = | Single-stranded deoxyribonucleic acid |
SARS | = | Severe acute respiratory syndrome |
Tat | = | Transactivator of transcription |
TBP | = | TAR-binding proteins |
TLE | = | tRNA-like element |
tRNA | = | Transfer ribonucleic acid |
TAR | = | Transactivation response element |
ZIKV | = | Zika Virus |
URL | = | U-rich Loop |
UTR | = | Untranslated region |
vDNA | = | Viral deoxyribonucleic acid |
XSI | = | X-ray scattering interferometry |
Acknowledgments
We acknowledge the financial support from the National Institutes of Health (R35GM138217) and from the National Science Foundation (CBET-1554558).
Disclosure statement
No potential conflict of interest was reported by the authors.
Additional information
Funding
References
- Mahmoudabadi G, Phillips R. A comprehensive and quantitative exploration of thousands of viral genomes. Elife. 2018;7:e31955. doi:10.7554/eLife.31955
- López-Lastra M, Ramdohr P, Letelier A, et al. Translation initiation of viral mRnas. Rev Med Virol. 2010;20(3):177–195. doi:10.1002/rmv.649
- Ortn J, Parra F. Structure and function of RNA replication. Annu Rev Microbiol. 2006;60:305–326. doi:10.1146/annurev.micro.60.080805.142248
- Kun L, Heng X, Summers MF. Structural determinants and mechanism of HIV-1 genome packaging. J Mol Biol. 2011;410(4):609–633. doi:10.1016/j.jmb.2011.04.029
- Bushell M, Sarnow P. Hijacking the translation apparatus by RNA viruses. J Cell Bio. 2002;158(3):395–399. doi:10.1083/jcb.200205044
- Dubois N, Marquet R, Paillart J-C, et al. Retroviral RNA dimerization: from structure to functions. Front Microbiol. 2018;9:527. doi:10.3389/fmicb.2018.00527
- Moore MD, Wei-Shau H. HIV-1 RNA dimerization: it takes two to tango. AIDS Rev. 2009;11(2):91.
- Pflug A, Guilligay D, Reich S, et al. Structure of influenza a polymerase bound to the viral RNA promoter. Nature. 2014;516(7531):355–360. doi:10.1038/nature14008
- Berkhout B, Silverman RH, Jeang K-T. Tat trans-activates the human immunodeficiency virus through a nascent RNA target. Cell. 1989;59(2):273–282. doi:10.1016/0092-8674(89)90289-4
- Lee K-M, Chen C-J, Shih S-R. Regulation mechanisms of viral IRES-driven translation. Trends Microbiol. 2017;25(7):546–561. doi:10.1016/j.tim.2017.01.010
- Kumar M, Kuroda K, Dhangar K, et al. Potential emergence of antiviral-resistant pandemic viruses via environmental drug exposure of animal reservoirs. Environ Sci Technol. 2020;54(14):8503–8505. doi:10.1021/acs.est.0c03105
- Boerneke MA, Ehrhardt JE, Weeks KM. Physical and functional analysis of viral RNA genomes by SHAPE. Annu Rev Virol. 2019;6(1):93–117. doi: 10.1146/annurev-virology-092917-043315
- Campillo-Balderas JA, Lazcano A, Becerra A. Viral genome size distribution does not correlate with the antiquity of the host lineages. Front Ecol Environ. 2015;3:143. doi:10.3389/fevo.2015.00143
- Baltimore D. Expression of animal virus genomes. Bacteriol Rev. 1971;35(3):235–241. doi:10.1128/br.35.3.235-241.1971
- Coffin J, Blomberg J, Fan H, et al. ICTV virus taxonomy profile: Retroviridae 2021. J Gen Virol. 2021;102(12):2021. doi: 10.1099/jgv.0.001712
- Weiss RA. The discovery of endogenous retroviruses. Retrovirology. 2006;3(1):1–11. doi: 10.1186/1742-4690-3-67
- Temin HM, Mizutami S. RNA-dependent DNA polymerase in virions of Rous sarcoma virus. Nature. 1970;226(5252):1211–1213. doi: 10.1038/2261211a0
- Baltimore D. Viral RNA-dependent DNA polymerase: RNA-dependent DNA polymerase in virions of RNA tumour viruses. Nature. 1970;226(5252):1209–1211. doi:10.1038/2261209a0
- Temin HM. Nature of the provirus of Rous sarcoma. Nat Cancer Inst Monogr. 1964;17:557–570.
- Barré-Sinoussi F, Chermann J-C, Rey F, et al. Isolation of a T-lymphotropic retrovirus from a patient at risk for acquired immune deficiency syndrome (AIDS). Science. 1983;220(4599):868–871. doi: 10.1126/science.6189183
- Gallo RC, Salahuddin SZ, Popovic M, et al. Frequent detection and isolation of cytopathic retroviruses (HTLV-III) from patients with AIDS and at risk for AIDS. Science. 1984;224(4648):500–503. doi: 10.1126/science.6200936
- Crespo R, Rao S, Mahmoudi T, et al. HIV-1 RNA metabolism and viral latency. Front Cell Infect Microbiol. 2022;12:733. doi: 10.3389/fcimb.2022.855092
- Chameettachal A, Mustafa F, Rizvi TA. Understanding retroviral life cycle and its genomic RNA packaging. J Mol Biol. 2022;435(3):167924. doi: 10.1016/j.jmb.2022.167924
- Turner BG, Summers MF. Structural biology of HIV 1 1Edited by P. E. Wright. J Mol Biol. 1999;285(1):1–32. doi:10.1006/jmbi.1998.2354
- Engelman A, Cherepanov P. The structural biology of HIV-1: mechanistic and therapeutic insights. Nat Rev Microbiol. 2012;10(4):279–290. doi:10.1038/nrmicro2747
- Nisole S, Sab A. Early steps of retrovirus replicative cycle. Retrovirology. 2004;1(1):9–20. doi: 10.1186/1742-4690-1-9
- Jiang M, Mak J, Ladha A, et al. Identification of tRnas incorporated into wild-type and mutant human immunodeficiency virus type 1. J Virol. 1993;67(6):3246–3253. doi:10.1128/jvi.67.6.3246-3253.1993
- Maertens GN, Engelman AN, Cherepanov P. Structure and function of retroviral integrase. Nat Rev Microbiol. 2022;20(1):20–34. doi:10.1038/s41579-021-00586-9
- Müller TG, Zila V, Müller B, et al. Nuclear capsid uncoating and reverse transcription of HIV-1. Annu Rev Virol. 2022;9(1):261–284. doi: 10.1146/annurev-virology-020922-110929
- D’Souza V, Summers MF. How retroviruses select their genomes. Nat Rev Microbiol. 2005;3(8):643–655. doi:10.1038/nrmicro1210
- Watts JM, Dang KK, Gorelick RJ, et al. Architecture and secondary structure of an entire HIV-1 RNA genome. Nature. 2009;460(7256):711–716. doi:10.1038/nature08237
- Tomezsko PJ, Corbin VD, Gupta P, et al. Determination of RNA structural diversity and its role in HIV-1 RNA splicing. Nature. 2020;582(7812):438–442. doi: 10.1038/s41586-020-2253-5
- Tomezsko P, Swaminathan H, Rouskin S. Viral RNA structure analysis using DMS-MaPseq. Methods. 2020;183:68–75. doi:10.1016/j.ymeth.2020.04.001
- Desselberger U, Racaniello VR, Zazra JJ, et al. The 3ʹ and 5ʹ-terminal sequences of influenza A, B and C virus RNA segments are highly conserved and show partial inverted complementarity. Gene. 1980;8(3):315–328. doi:10.1016/0378-1119(80)90007-4
- Brierley I, Digard P, Inglis SC. Characterization of an efficient coronavirus ribosomal frameshifting signal: requirement for an RNA pseudoknot. Cell. 1989;57(4):537–547. doi:10.1016/0092-8674(89)90124-4
- Aldovini A, Young RA. Mutations of RNA and protein sequences involved in human immunodeficiency virus type 1 packaging result in production of noninfectious virus. J Virol. 1990;64(5):1920–1926. doi:10.1128/jvi.64.5.1920-1926.1990
- Jensen S, Randrup Thomsen A. Sensing of RNA viruses: a review of innate immune receptors involved in recognizing RNA virus invasion. J Virol. 2012;86(6):2900–2910. doi:10.1128/JVI.05738-11
- Al-Hashimi HM. NMR studies of nucleic acid dynamics. J Magn Reson. 2013;237:191–204. doi:10.1016/j.jmr.2013.08.014
- Liu B, Shi H, Al-Hashimi HM. Developments in solution-state NMR yield broader and deeper views of the dynamic ensembles of nucleic acids. Curr Opin Struct Biol. 2021;70:16–25. doi:10.1016/j.sbi.2021.02.007
- Jackson RW, Smathers CM, Robart AR. General strategies for RNA X-ray crystallography. Molecules. 2023;28(5):2111. doi:10.3390/molecules28052111
- Haiyun M, Jia X, Zhang K, et al. Cryo-EM advances in RNA structure determination. Signal Transduct Target Ther. 2022;7(1):58. doi:10.1038/s41392-022-00916-0
- Wei-Shau H, Hughes SH. HIV-1 reverse transcription. Cold Spring Harb Perspect Med. 2012;2(10):a006882. doi:10.1101/cshperspect.a006882
- Liu S, Comandur R, Jones CP, et al. Anticodon-like binding of the HIV-1 tRNA-like element to human lysyl-tRNA synthetase. RNA. 2016;22(12):1828–1835. doi:10.1261/rna.058081.116
- Cen S, Javanbakht H, Kim S, et al. Retrovirus-specific packaging of aminoacyl-tRNA synthetases with cognate primer tRnas. J Virol. 2002;76(24):13111–13115. doi:10.1128/JVI.76.24.13111-13115.2002
- Dewan V, Reader J, Forsyth K-M. Role of aminoacyl-tRNA synthetases in infectious diseases and targets for therapeutic development. Top Curr Chem. 2014;344:293–329.
- Bilbille Y, Vendeix FA, Guenther R, et al. The structure of the human tRNA3Lys anticodon bound to the HIV genome is stabilized by modified nucleosides and adjacent mismatch base pairs. Nucleic Acids Res. 2009;37(10):3342–3353. doi:10.1093/nar/gkp187
- Song Z, Gremminger T, Singh G, et al. The three-way junction structure of the HIV-1 PBS-segment binds host enzyme important for viral infectivity. Nucleic Acids Res. 2021;49(10):5925–5942. doi: 10.1093/nar/gkab342
- Das K, Martinez SE, DeStefano JJ, et al. Structure of HIV-1 RT/dsRNA initiation complex prior to nucleotide incorporation. Proc Natl Acad Sci, USA. 2019;116(15):7308–7313. doi:10.1073/pnas.1814170116
- Larsen KP, Kalyani Mathiharan Y, Kappel K, et al. Architecture of an HIV-1 reverse transcriptase initiation complex. Nature. 2018;557(7703):118–122. doi:10.1038/s41586-018-0055-9
- Das K, Martinez SE, Arnold E. Structural insights into HIV reverse transcriptase mutations Q151M and Q151M complex that confer multinucleoside drug resistance. Antimicrob Agents Chemother. 2017;61(6):e00224–17. doi:10.1128/AAC.00224-17
- Michiko Obayashi C, Shinohara Y, Masuda T, et al. Influence of the 5ʹ-terminal sequences on the 5ʹ-UTR structure of HIV-1 genomic RNA. Sci Rep. 2021;11(1):10920. doi:10.1038/s41598-021-90427-9
- Bourbigot S, Ramalanjaona N, Boudier C, et al. How the HIV-1 nucleocapsid protein binds and destabilises the (-)primer binding site during reverse transcription. J Mol Biol. 2008;383(5):1112–1128. doi:10.1016/j.jmb.2008.08.046
- Larsen KP, Choi J, Jackson LN, et al. Distinct conformational states underlie pausing during initiation of HIV-1 reverse transcription. J Mol Biol. 2020;432(16):4499–4522. doi:10.1016/j.jmb.2020.06.003
- Betty H, Larsen KP, Zhang J, et al. High-resolution view of HIV-1 reverse transcriptase initiation complexes and inhibition by NNRTI drugs. Nat Commun. 2021;12(1):2500. doi:10.1038/s41467-021-22628-9
- Das K, Martinez SE, Bandwar RP, et al. Structures of HIV-1 RT-RNA/DNA ternary complexes with dATP and nevirapine reveal conformational flexibility of RNA/DNA: insights into requirements for RNase H cleavage. Nucleic Acids Res. 2014;42(12):8125–8137. doi:10.1093/nar/gku487
- František Potužnk J, Cahová H, Prasad VR, et al. It’s the little things (in viral RNA). MBio. 2020;11(5):e02131–20. doi:10.1128/mBio.02131-20
- El Kazzi P, Rabah N, Chamontin C, et al. Internal RNA 2ʹO-methylation in the HIV-1 genome counteracts ISG20 nuclease-mediated antiviral effect. Nucleic Acids Res. 2023;51(6):2501–2515. doi: 10.1093/nar/gkac996
- Kroun Damgaard C, Sloth Andersen E, Knudsen B, et al. RNA interactions in the 5ʹ region of the HIV-1 genome. J Mol Biol. 2004;336(2):369–379. doi:10.1016/j.jmb.2003.12.010
- Wilkinson KA, Gorelick RJ, Vasa SM, et al. High-throughput SHAPE analysis reveals structures in HIV-1 genomic RNA strongly conserved across distinct biological states. PLoS Biol. 2008;6(4):e96. doi:10.1371/journal.pbio.0060096
- Coey A, Larsen K, Puglisi JD, et al. Heterogeneous structures formed by conserved RNA sequences within the HIV reverse transcription initiation site. RNA. 2016;22(11):1689–1698. doi:10.1261/rna.056804.116
- Gilboa E, Mitra SW, Goff S, et al. A detailed model of reverse transcription and tests of crucial aspects. Cell. 1979;18(1):93–100. doi:10.1016/0092-8674(79)90357-X
- Fukuda H, Chujo T, Wei F-Y, et al. Cooperative methylation of human tRNA3Lys at positions A58 and U54 drives the early and late steps of HIV-1 replication. Nucleic Acids Res. 2021;49(20):11855–11867. doi:10.1093/nar/gkab879
- Comandur R, Olson ED, Musier-Forsyth K. Conservation of tRNA mimicry in the 5ʹ-untranslated region of distinct HIV-1 subtypes. RNA. 2017;23(12):1850–1859. doi:10.1261/rna.062182.117
- Cantara WA, Pathirage C, Hatterschide J, et al. Phosphomimetic S207D lysyl — tRNA synthetase binds HIV-1 5ʹ UTR in an open conformation and increases RNA dynamics. Viruses. 2022;14(7):1556. doi:10.3390/v14071556
- Isel C, Ehresmann C, Marquet R. Initiation of HIV reverse transcription. Viruses. 2010;2(1):213–243. doi:10.3390/v2010213
- Kohlstaedt LA, Wang J, Friedman JM, et al. Crystal structure at 3.5 Å resolution of HIV-1 reverse transcriptase complexed with an inhibitor. Science. 1992;256(5065):1783–1790. doi:10.1126/science.1377403
- Jacobo-Molina A, Ding J, Nanni RG, et al. Crystal structure of human immunodeficiency virus type 1 reverse transcriptase complexed with double-stranded DNA at 3.0 a resolution shows bent DNA. Proc Natl Acad Sci, USA. 1993;90(13):6320–6324. doi:10.1073/pnas.90.13.6320
- Rodgers DW, Gamblin SJ, Harris BA, et al. The structure of unliganded reverse transcriptase from the human immunodeficiency virus type 1. Proc Natl Acad Sci, USA. 1995;92(4):1222–1226. doi:10.1073/pnas.92.4.1222
- Huang H, Chopra R, Verdine GL, et al. Structure of a covalently trapped catalytic complex of HIV-1 reverse transcriptase: implications for drug resistance. Science. 1998;282(5394):1669–1675. doi:10.1126/science.282.5394.1669
- Guangdi L, Wang Y, De Clercq E. Approved HIV reverse transcriptase inhibitors in the past decade. Acta Pharm Sin B. 2022;12(4):1567–1590. doi:10.1016/j.apsb.2021.11.009
- Lange MJ, Nguyen PD, Callaway MK, et al. RNA-protein interactions govern antiviral specificity and encapsidation of broad spectrum anti-HIV reverse transcriptase aptamers. Nucleic Acids Res. 2017;45(10):6087–6097. doi:10.1093/nar/gkx155
- Wang M-F, Yan L, Xu-Dan B, et al. Polypyridyl ruthenium complexes as bifunctional TAR RNA binders and HIV-1 reverse transcriptase inhibitors. J Inorg Biochem. 2022;234:111880. doi:10.1016/j.jinorgbio.2022.111880
- Guo Y-X, Liu M, Zhou Y-Q, et al. Terpyridyl ruthenium complexes as visible spectral probe for poly (A) RNA and bifunctional TAR RNA binders and HIV-1 reverse transcriptase inhibitors. Inorganica Chim Acta. 2022;539:121027. doi:10.1016/j.ica.2022.121027
- Wang Z, Wang W, Cheng Cui Y, et al. HIV-1 employs multiple mechanisms to resist Cas9/single guide RNA targeting the viral primer binding site. J Virol. 2018;92(20):e01135–18. doi:10.1128/JVI.01135-18
- Kaminski R, Chen Y, Salkind J, et al. Negative feedback regulation of HIV-1 by gene editing strategy. Sci Rep. 2016;6(1):31527. doi:10.1038/srep31527
- Beltz H, Clauss C, Piémont E, et al. Structural determinants of HIV-1 nucleocapsid protein for cTAR DNA binding and destabilization, and correlation with inhibition of self-primed DNA synthesis. J Mol Biol. 2005;348(5):1113–1126. doi:10.1016/j.jmb.2005.02.042
- Purohit Basu V, Song M, Gao L, et al. Strand transfer events during HIV-1 reverse transcription. Virus Res. 2008;134(1–2):19–38. doi:10.1016/j.virusres.2007.12.017
- Chen Y, Balakrishnan M, Roques BP, et al. Mechanism of minus strand strong stop transfer in HIV-1 reverse transcription. J Biol Chem. 2003;278(10):8006–8017. doi:10.1074/jbc.M210959200
- Ohi Y, Clever JL. Sequences in the 5ʹ and 3ʹ R elements of human immunodeficiency virus type 1 critical for efficient reverse transcription. J Virol. 2000;74(18):8324–8334. doi:10.1128/JVI.74.18.8324-8334.2000
- Belfetmi A, Zargarian L, Tisné C, et al. Insights into the mechanisms of RNA secondary structure destabilization by the HIV-1 nucleocapsid protein. RNA. 2016;22(4):506–517. doi: 10.1261/rna.054445.115
- McCauley MJ, Rouzina I, Jasmine L, et al. Significant differences in RNA structure destabilization by HIV-1 Gag∆p6 and NCp7 proteins. Viruses. 2020;12(5):484. doi:10.3390/v12050484
- Telesnitsky A, Goff SP. Reverse transcriptase and the generation of retroviral DNA. Retroviruses. 2011.
- Imamichi H, Dewar RL, Adelsberger JW, et al. Defective HIV-1 proviruses produce novel protein-coding RNA species in HIV-infected patients on combination antiretroviral therapy. Proc Natl Acad Sci, USA. 2016;113(31):8783–8788. doi:10.1073/pnas.1609057113
- Wiegand A, Spindler J, Hong FF, et al. Single-cell analysis of HIV-1 transcriptional activity reveals expression of proviruses in expanded clones during ART. Proc Natl Acad Sci, USA. 2017;114(18):E3659–E3668. doi:10.1073/pnas.1617961114
- Pasternak AO, Berkhout B. What do we measure when we measure cell-associated HIV RNA. Retrovirology. 2018;15(1):13. doi:10.1186/s12977-018-0397-2
- Shortridge MD, Wille PT, Jones AN, et al. An ultra-high affinity ligand of HIV-1 TAR reveals the RNA structure recognized by P-TEFb. Nucleic Acids Res. 2019;47(3):1523–1531. doi:10.1093/nar/gky1197
- Aboul-Ela F, Karn J, Varani G. The structure of the human immunodeficiency virus type-1 TAR RNA reveals principles of RNA recognition by Tat protein. J Mol Biol. 1995;253(2):313–332. doi:10.1006/jmbi.1995.0555
- Faber C, Sticht H, Schweimer K, et al. Structural rearrangements of HIV-1 Tat-responsive RNA upon binding of neomycin B. J Biol Chem. 2000;275(27):20660–20666. doi:10.1074/jbc.M000920200
- Zhihua D, Lind KE, James TL. Structure of TAR RNA complexed with a Tat-TAR interaction nanomolar inhibitor that was identified by computational screening. Chem Biol. 2002;9(6):707–712. doi:10.1016/S1074-5521(02)00151-5
- Murchie AI, Davis B, Isel C, et al. Structure-based drug design targeting an inactive RNA conformation: exploiting the flexibility of HIV-1 TAR RNA. J Mol Biol. 2004;336(3):625–638. doi: 10.1016/j.jmb.2003.12.028
- Davis B, Afshar M, Varani G, et al. Rational design of inhibitors of HIV-1 TAR RNA through the stabilisation of electrostatic “hot spots”. J Mol Biol. 2004;336(2):343–356. doi: 10.1016/j.jmb.2003.12.046
- Davidson A, Leeper TC, Athanassiou Z, et al. Simultaneous recognition of HIV-1 TAR RNA bulge and loop sequences by cyclic peptide mimics of Tat protein. Proc Natl Acad Sci, USA. 2009;106(29):11931–11936. doi:10.1073/pnas.0900629106
- Davidson A, Begley DW, Lau C, et al. A small-molecule probe induces a conformation in HIV TAR RNA capable of binding drug-like fragments. J Mol Biol. 2011;410(5):984–996. doi:10.1016/j.jmb.2011.03.039
- Davidson A, Patora-Komisarska K, Robinson JA, et al. Essential structural requirements for specific recognition of HIV TAR RNA by peptide mimetics of Tat protein. Nucleic Acids Res. 2011;39(1):248–256. doi:10.1093/nar/gkq713
- Borkar AN, Michael F Bardaro CC Jr, Aprile FA, et al. Structure of a low-population binding intermediate in protein-RNA recognition. Proc Natl Acad Sci, USA. 2016;113(26):7171–7176. doi:10.1073/pnas.1521349113
- Aboul-Ela F, Karn J, Varani G. Structure of HIV-1 TAR RNA in the absence of ligands reveals a novel conformation of the trinucleotide bulge. Nucleic Acids Res. 1996;24(20):3974–3981. doi:10.1093/nar/24.20.3974
- Shashank Chavali S, Mali SM, Jenkins JL, et al. Co-crystal structures of HIV TAR RNA bound to lab-evolved proteins show key roles for arginine relevant to the design of cyclic peptide TAR inhibitors. J Biol Chem. 2020;295(49):16470–16486. doi:10.1074/jbc.RA120.015444
- Belashov IA, Crawford DW, Cavender CE, et al. Structure of HIV TAR in complex with a lab-evolved RRM provides insight into duplex RNA recognition and synthesis of a constrained peptide that impairs transcription. Nucleic Acids Res. 2018;46(13):6401–6415. doi:10.1093/nar/gky529
- Pham VV, Salguero C, Nahar Khan S, et al. HIV-1 Tat interactions with cellular 7SK and viral TAR RNAs identifies dual structural mimicry. Nat Commun. 2018;9(1):4266. doi:10.1038/s41467-018-06591-6
- De Nicola B, Lech CJ, Heddi B, et al. Structure and possible function of a G-quadruplex in the long terminal repeat of the proviral HIV-1 genome. Nucleic Acids Res. 2016;44(13):6442–6451. doi:10.1093/nar/gkw432
- Butovskaya E, Heddi B, Bakalar B, et al. Major G-quadruplex form of HIV-1 LTR reveals a (3+1) folding topology containing a stem-loop. J Am Chem Soc. 2018;140(42):13654–13662. doi:10.1021/jacs.8b05332
- Schulze-Gahmen U, Hurley JH. Structural mechanism for HIV-1 TAR loop recognition by Tat and the super elongation complex. Proc Natl Acad Sci, USA. 2018;115(51):12973–12978. doi:10.1073/pnas.1806438115
- Huang W, Emani PS, Varani G, et al. Ultraslow domain motions in HIV-1 TAR RNA revealed by solid-state deuterium NMR. J Phys Chem B. 2017;121(1):110–117. doi:10.1021/acs.jpcb.6b11041
- Krawczyk K, Sim AY, Knapp B, et al. Tertiary element interaction in HIV-1 TAR. J Chem Inf Model. 2016;56(9):1746–1754. doi:10.1021/acs.jcim.6b00152
- Schulze-Gahmen U, Echeverria I, Stjepanovic G, et al. Insights into HIV-1 proviral transcription from integrative structure and dynamics of the Tat: AFF4: P-TEFb: TAR complex. Elife. 2016;5:e15910. doi:10.7554/eLife.15910
- Levintov L, Vashisth H. Role of conformational heterogeneity in ligand recognition by viral RNA molecules. Phys Chem Chem Phys. 2021;23(19):11211–11223. doi:10.1039/D1CP00679G
- Malina J, Hannon MJ, Brabec V. Iron (II) supramolecular helicates interfere with the HIV-1 Tat–TAR RNA interaction critical for viral replication. Sci Rep. 2016;6(1):29674. doi:10.1038/srep29674
- Cardo L, Nawroth I, Cail PJ, et al. Metallo supramolecular cylinders inhibit HIV-1 TAR-TAT complex formation and viral replication in cellulo. Sci Rep. 2018;8(1):13342. doi:10.1038/s41598-018-31513-3
- Alanazi A, Ivanov A, Kumari N, et al. Targeting Tat-TAR RNA interaction for HIV-1 inhibition. Viruses. 2021;13(10):2004. doi:10.3390/v13102004
- Selberg S, Zusinaite E, Herodes K, et al. HIV replication is increased by RNA methylation METTL3/METTL14/WTAP complex activators. ACS Omega. 2021;6(24):15957–15963. doi:10.1021/acsomega.1c01626
- Do TN, Ippoliti E, Carloni P, et al. Counterion redistribution upon binding of a Tat-protein mimic to HIV-1 TAR RNA. J Chem Theory Comput. 2012;8(2):688–694. doi:10.1021/ct2005769
- Crawford DW, Blakeley BD, Chen P-H, et al. An evolved RNA recognition motif that suppresses HIV-1 Tat/TAR-dependent transcription. ACS Chem Biol. 2016;11(8):2206–2215. doi:10.1021/acschembio.6b00145
- Kumar A, Vashisth H. Conformational dynamics and energetics of viral RNA recognition by lab-evolved proteins. Phys Chem Chem Phys. 2021;23(43):24773–24779. doi:10.1039/D1CP03822B
- Brown JD, Kharytonchyk S, Chaudry I, et al. Structural basis for transcriptional start site control of HIV-1 RNA fate. Science. 2020;368(6489):413–417. doi: 10.1126/science.aaz7959
- Calnan BJ, Tidor B, Biancalana S, et al. Arginine-mediated RNA recognition: the arginine fork. Science. 1991;252(5009):1167–1171. doi:10.1126/science.252.5009.1167
- Pitt SW, Majumdar A, Serganov A, et al. Argininamide binding arrests global motions in HIV-1 TAR RNA: comparison with Mg2±induced conformational stabilization. J Mol Biol. 2004;338(1):7–16. doi:10.1016/j.jmb.2004.02.031
- Olson SW, Turner A-MW, Winston Arney J, et al. Discovery of a large-scale, cell-state-responsive allosteric switch in the 7SK RNA using DANCE-MaP. Mol Cell. 2022;82(9):1708–1723. doi:10.1016/j.molcel.2022.02.009
- Min Kim J, Sun Choi H, Lin Seong B. The folding competence of HIV-1 Tat mediated by interaction with TAR RNA. RNA Biol. 2017;14(7):926–937. doi:10.1080/15476286.2017.1311455
- Yan L, Belshan M. NF45 and NF90 bind HIV-1 RNA and modulate HIV gene expression. Viruses. 2016;8(2):47. doi:10.3390/v8020047
- Rocchi C, Louvat C, Erica Miele A, et al. The HIV-1 integrase C-Terminal domain induces TAR RNA structural changes promoting Tat binding. Int J Mol Sci. 2022;23(22):13742. doi:10.3390/ijms232213742
- Perrone R, Doria F, Butovskaya E, et al. Synthesis, binding and antiviral properties of potent core-extended naphthalene diimides targeting the HIV-1 long terminal repeat promoter G-quadruplexes. J Med Chem. 2015;58(24):9639–9652. doi:10.1021/acs.jmedchem.5b01283
- Amrane S, Jaubert C, Bedrat A, et al. Deciphering RNA G-quadruplex function during the early steps of HIV-1 infection. Nucleic Acids Res. 2022;50(21):12328–12343. doi:10.1093/nar/gkac1030
- Harpster C, Boyle E, Musier-Forsyth K, et al. HIV-1 genomic RNA U3 region forms a stable quadruplex-hairpin structure. Biophys Chem. 2021;272:106567. doi:10.1016/j.bpc.2021.106567
- Battiste JL, Hongyuan Mao NSR, Ruoying Tan DM, et al. α helix-RNA major groove recognition in an HIV-1 Rev peptide-RRE RNA complex. Science. 1996;273(5281):1547–1551. doi:10.1126/science.273.5281.1547
- Ippolito JA, Steitz TA. The structure of the HIV-1 RRE high affinity rev binding site at 1.6 Å resolution. J Mol Biol. 2000;295(4):711–717. doi:10.1006/jmbi.1999.3405
- Hung L-W, Holbrook EL, Holbrook SR. The crystal structure of the Rev binding element of HIV-1 reveals novel base pairing and conformational variability. Proc Natl Acad Sci, USA. 2000;97(10):5107–5112. doi:10.1073/pnas.090588197
- Gosser Y, Hermann T, Majumdar A, et al. Peptide-triggered conformational switch in HIV-1 RRE RNA complexes. Nat Struct Biol. 2001;8(2):146–150. doi:10.1038/84138
- Zhang Q, Harada K, Cho HS, et al. Structural characterization of the complex of the Rev response element RNA with a selected peptide. Chem Biol. 2001;8(5):511–520. doi:10.1016/S1074-5521(01)00027-8
- Jayaraman B, Crosby DC, Homer C, et al. RNA-directed remodeling of the HIV-1 protein Rev orchestrates assembly of the Rev-Rev response element complex. Elife. 2014;3:e04120. doi:10.7554/eLife.04120
- Jackson PE, Dzhivhuho G, Rekosh D, et al. Sequence and functional variation in the HIV-1 Rev regulatory axis. Curr HIV Res. 2020;18(2):85–98. doi:10.2174/1570162X18666200106112842
- Malim MH, Hauber J, Shu-Yun L, et al. The HIV-1 rev trans-activator acts through a structured target sequence to activate nuclear export of unspliced viral mRNA. Nature. 1989;338(6212):254–257. doi:10.1038/338254a0
- Cullen BR. Nuclear mRNA export: insights from virology. Trends Biochem Sci. 2003;28(8):419–424. doi:10.1016/S0968-0004(03)00142-7
- Rausch JW, Le Grice SF. HIV Rev assembly on the Rev response element (RRE): a structural perspective. Viruses. 2015;7(6):3053–3075. doi:10.3390/v7062760
- Schneeberger E-M, Halper M, Palasser M, et al. Native mass spectrometry reveals the initial binding events of HIV-1 rev to RRE stem II RNA. Nat Commun. 2020;11(1):5750. doi:10.1038/s41467-020-19144-7
- Henderson BR, Percipalle P. Interactions between HIV Rev and nuclear import and export factors: the Rev nuclear localisation signal mediates specific binding to human importin-β. J Mol Biol. 1997;274(5):693–707. doi:10.1006/jmbi.1997.1420
- Askjaer P, Heick Jensen T, Nilsson J, et al. The specificity of the CRM1-Rev nuclear export signal interaction is mediated by RanGTP. J Biol Chem. 1998;273(50):33414–33422. doi:10.1074/jbc.273.50.33414
- Liu H, Pei-Wen H, Budhiraja S, et al. PACS1 is an HIV-1 cofactor that functions in Rev-mediated nuclear export of viral RNA. Virology. 2020;540:88–96. doi:10.1016/j.virol.2019.10.004
- Kjems J, Brown M, Chang DD, et al. Structural analysis of the interaction between the human immunodeficiency virus Rev protein and the Rev response element. Proc Natl Acad Sci, USA. 1991;88(3):683–687. doi:10.1073/pnas.88.3.683
- Mann DA, Mikaélian I, Zemmel RW, et al. A molecular rheostat: co-operative rev binding to stem I of the Rev-response element modulates human immunodeficiency virus type-1 late gene expression. J Mol Biol. 1994;241(2):193–207. doi: 10.1006/jmbi.1994.1488
- Charpentier B, Stutz F, Rosbash M. A dynamic in vivo view of the HIV-IRev-RRE interaction. J Mol Biol. 1997;266(5):950–962. doi:10.1006/jmbi.1996.0858
- Sherpa C, Rausch JW, Le Grice SF, et al. The HIV-1 Rev response element (RRE) adopts alternative conformations that promote different rates of virus replication. Nucleic Acids Res. 2015;43(9):4676–4686. doi:10.1093/nar/gkv313
- Zemmel RW, Kelley AC, Karn J, et al. Flexible regions of RNA structure facilitate co-operative Rev assembly on the Rev-response element. J Mol Biol. 1996;258(5):763–777. doi:10.1006/jmbi.1996.0285
- Peterson RD, Feigon J. Structural change in rev responsive element RNA of HIV-1 on binding rev peptide. J Mol Biol. 1996;264(5):863–877. doi:10.1006/jmbi.1996.0683
- Chu C-C, Plangger R, Kreutz C, et al. Dynamic ensemble of HIV-1 RRE stem IIB reveals non-native conformations that disrupt the Rev-binding site. Nucleic Acids Res. 2019;47(13):7105–7117. doi:10.1093/nar/gkz498
- Chu C-C, Liu B, Plangger R, et al. m6A minimally impacts the structure, dynamics, and Rev ARM binding properties of HIV-1 RRE stem IIB. PLoS One. 2019;14(12):e0224850. doi:10.1371/journal.pone.0224850
- Shi Y, Han J, Zhu B, et al. Limited nucleotide changes of HIV-1 subtype B Rev response element in China affect overall Rev-RRE activity and viral replication. Front Microbiol. 2022;13: doi: 10.3389/fmicb.2022.1044676
- Kumar A, Vashisth H. Role of mutations in differential recognition of viral RNA molecules by peptides. J Chem Inf Model. 2022;62(14):3381–3390. doi:10.1021/acs.jcim.2c00376
- Hammond JA, Lamichhane R, Millar DP, et al. A DEAD-box helicase mediates an RNA structural transition in the HIV-1 Rev response element. J Mol Biol. 2017;429(5):697–714. doi:10.1016/j.jmb.2017.01.018
- Dzhivhuho G, Holsey J, Honeycutt E, et al. HIV-1 Rev-RRE functional activity in primary isolates is highly dependent on minimal context-dependent changes in Rev. Sci Rep. 2022;12(1):18416. doi:10.1038/s41598-022-21714-2
- Chen J, Umunnakwe C, Sun DQ, et al. Impact of nuclear export pathway on cytoplasmic HIV-1 RNA transport mechanism and distribution. MBio. 2020;11(6):e01578–20. doi:10.1128/mBio.01578-20
- Levintov L, Vashisth H. Role of salt-bridging interactions in recognition of viral RNA by arginine-rich peptides. Biophys J. 2021;120(22):5060–5073. doi:10.1016/j.bpj.2021.10.007
- Balvay L, Lopez Lastra M, Sargueil B, et al. Translational control of retroviruses. Nat Rev Microbiol. 2007;5(2):128–140. doi:10.1038/nrmicro1599
- Tazi J, Bakkour N, Marchand V, et al. Alternative splicing: regulation of HIV-1 multiplication as a target for therapeutic action. FEBS J. 2010;277(4):867–876. doi:10.1111/j.1742-4658.2009.07522.x
- Karn J, Martin Stoltzfus C. Transcriptional and posttranscriptional regulation of HIV-1 gene expression. Cold Spring Harb Perspect Med. 2012;2(2):a006916. doi:10.1101/cshperspect.a006916
- Pelletier J, Sonenberg N. The organizing principles of eukaryotic ribosome recruitment. Annu Rev Biochem. 2019;88(1):307–335. doi: 10.1146/annurev-biochem-013118-111042
- De Breyne S, Ohlmann T. Focus on translation initiation of the HIV-1 mRNAS. Int J Mol Sci. 2019;20(1):101. doi:10.3390/ijms20010101
- Kieft JS. Viral IRES RNA structures and ribosome interactions. Trends Biochem Sci. 2008;33(6):274–283. doi:10.1016/j.tibs.2008.04.007
- Zhu J, Korostelev A, Costantino DA, et al. Crystal structures of complexes containing domains from two viral internal ribosome entry site (IRES) RNAs bound to the 70S ribosome. Proc Natl Acad Sci, USA. 2011;108(5):1839–1844. doi:10.1073/pnas.1018582108
- Bhatt PR, Scaiola A, Loughran G, et al. Structural basis of ribosomal frameshifting during translation of the SARS-CoV-2 RNA genome. Science. 2021;372(6548):1306–1313. doi: 10.1126/science.abf3546
- Ohlmann T, Mengardi C, López-Lastra M. Translation initiation of the HIV-1 mRNA. Translation. 2014;2(2):e960242. doi:10.4161/2169074X.2014.960242
- Smirnova VV, Terenin IM, Khutornenko AA, et al. Does HIV-1 mRNA 5ʹ-untranslated region bear an internal ribosome entry site? Biochimie. 2016;121:228–237. doi:10.1016/j.biochi.2015.12.004
- Amorim R, Mesquita Costa S, Pereira Cavaleiro N, et al. Edson Elias da Silva, and Luciana Jesus da Costa. HIV-1 transcripts use IRES-initiation under conditions where cap-dependent translation is restricted by poliovirus 2A protease. PLoS One. 2014;9(2):e88619. doi:10.1371/journal.pone.0088619
- Dugre-Brisson S, Elvira G, Boulay K, et al. Interaction of Staufen1 with the 5ʹ end of mRNA facilitates translation of these RNAs. Nucleic Acids Res. 2005;33(15):4797–4812. doi:10.1093/nar/gki794
- Ramos H, Monette A, Niu M, et al. The double-stranded RNA-binding protein, Staufen1, is an IRES-transacting factor regulating HIV-1 cap-independent translation initiation. Nucleic Acids Res. 2022;50(1):411–429. doi:10.1093/nar/gkab1188
- Guerrero S, Batisse J, Libre C, et al. HIV-1 replication and the cellular eukaryotic translation apparatus. Viruses. 2015;7(1):199–218. doi:10.3390/v7010199
- Ketteler R. On programmed ribosomal frameshifting: the alternative proteomes. Front Genet. 2012;3:242. doi:10.3389/fgene.2012.00242
- Ritchie DB, Cappellano TR, Tittle C, et al. Conformational dynamics of the frameshift stimulatory structure in HIV-1. RNA. 2017;23(9):1376–1384. doi:10.1261/rna.061655.117
- Garcia-Miranda P, Becker JT, Benner BE, et al. Stability of HIV frameshift site RNA correlates with frameshift efficiency and decreased virus infectivity. J Virol. 2016;90(15):6906–6917. doi:10.1128/JVI.00149-16
- Hilimire TA, Chamberlain JM, Anokhina V, et al. HIV-1 frameshift RNA-targeted triazoles inhibit propagation of replication-competent and multi-drug-resistant HIV in human cells. ACS Chem Biol. 2017;12(6):1674–1682. doi:10.1021/acschembio.7b00052
- Takata MA, Gonçalves-Carneiro D, Zang TM, et al. CG dinucleotide suppression enables antiviral defence targeting non-self RNA. Nature. 2017;550(7674):124–127. doi:10.1038/nature24039
- Antzin-Anduetza I, Mahiet C, Granger LA, et al. Increasing the CpG dinucleotide abundance in the HIV-1 genomic RNA inhibits viral replication. Retrovirology. 2017;14(1):1–18. doi: 10.1186/s12977-017-0374-1
- Xue G, Braczyk K, Gonçalves-Carneiro D, et al. Poly(adp-ribose) potentiates ZAP antiviral activity. PLOS Pathog. 2022;18(2):e1009202. doi: 10.1371/journal.ppat.1009202
- Kennedy EM, Bogerd HP, Kornepati AV, et al. Posttranscriptional m6A editing of HIV-1 mRNAs enhances viral gene expression. Cell Host Microbe. 2016;19(5):675–685. doi:10.1016/j.chom.2016.04.002
- Courtney DG, Tsai K, Bogerd HP, et al. Epitranscriptomic addition of m5C to HIV-1 transcripts regulates viral gene expression. Cell Host Microbe. 2019;26(2):217–227. doi:10.1016/j.chom.2019.07.005
- Ringeard M, Marchand V, Decroly E, et al. FTSJ3 is an RNA 2ʹ-O-methyltransferase recruited by HIV to avoid innate immune sensing. Nature. 2019;565(7740):500–504. doi:10.1038/s41586-018-0841-4
- Tirumuru N, Simen Zhao B, Wuxun L, et al. N6-methyladenosine of HIV-1 RNA regulates viral infection and HIV-1 Gag protein expression. Elife. 2016;5:e15528. doi:10.7554/eLife.15528
- Šimonová A, Svojanovská B, Trylčová J, et al. LC/MS analysis and deep sequencing reveal the accurate RNA composition in the HIV-1 virion. Sci Rep. 2019;9(1):8697. doi: 10.1038/s41598-019-45079-1
- Tsai K, Ayyappan Jaguva Vasudevan A, Martinez Campos C, et al. Acetylation of cytidine residues boosts HIV-1 gene expression by increasing viral RNA stability. Cell Host Microbe. 2020;28(2):306–312. doi:10.1016/j.chom.2020.05.011
- Keane SC, Heng X, Kun L, et al. Structure of the HIV-1 RNA packaging signal. Science. 2015;348(6237):917–921. doi: 10.1126/science.aaa9266
- Greatorex J, Gallego J, Varani G, et al. Structure and stability of wild-type and mutant RNA internal loops from the SL-1 domain of the HIV-1 packaging signal. J Mol Biol. 2002;322(3):543–557. doi:10.1016/S0022-2836(02)00776-3
- Lawrence DC, Stover CC, Noznitsky J, et al. Structure of the intact stem and bulge of HIV-1 ψ-RNA stem-loop SL1. J Mol Biol. 2003;326(2):529–542. doi:10.1016/S0022-2836(02)01305-0
- Yuan Y, Kerwood DJ, Paoletti AC, et al. Stem of SL1 RNA in HIV-1: structure and nucleocapsid protein binding for a 1× 3 internal loop. Biochemistry. 2003;42(18):5259–5269. doi:10.1021/bi034084a
- Baba S, Takahashi K-I, Noguchi S, et al. Solution RNA structures of the HIV-1 dimerization initiation site in the kissing-loop and extended-duplex dimers. J Biochem. 2005;138(5):583–592. doi:10.1093/jb/mvi158
- Girard F, Barbault F, Gouyette C, et al. Dimer initiation sequence of HIV-1Lai genomic RNA: NMR solution structure of the extended duplex. J Biomol Struct Dyn. 1999;16(6):1145–1157. doi:10.1080/07391102.1999.10508323
- Ennifar E, Yusupov M, Walter P, et al. The crystal structure of the dimerization initiation site of genomic HIV-1 RNA reveals an extended duplex with two adenine bulges. Structure. 1999;7(11):1439–1449. doi:10.1016/S0969-2126(00)80033-7
- Ennifar E, Walter P, Dumas P. A crystallographic study of the binding of 13 metal ions to two related RNA duplexes. Nucleic Acids Res. 2003;31(10):2671–2682. doi:10.1093/nar/gkg350
- Freisz S, Lang K, Micura R, et al. Binding of aminoglycoside antibiotics to the duplex form of the HIV-1 genomic RNA dimerization initiation site. Angew Chem Int Ed Engl. 2008;47(22):4110–4113. doi:10.1002/anie.200800726
- Ulyanov NB, Mujeeb A, Zhihua D, et al. NMR structure of the full-length linear dimer of stem-loop-1 RNA in the HIV-1 dimer initiation site. J Biol Chem. 2006;281(23):16168–16177. doi:10.1074/jbc.M601711200
- Ennifar E, Walter P, Dumas P. Cation-dependent cleavage of the duplex form of the subtype-B HIV-1 RNA dimerization initiation site. Nucleic Acids Res. 2010;38(17):5807–5816. doi:10.1093/nar/gkq344
- Zhang K, Keane SC, Zhaoming S, et al. Structure of the 30 kDa HIV-1 RNA dimerization signal by a hybrid cryo-EM, NMR, and molecular dynamics approach. Structure. 2018;26(3):490–498. doi:10.1016/j.str.2018.01.001
- Mujeeb A, Clever JL, Billeci TM, et al. Structure of the dimer a initiation complex of HIV-1 genomic RNA. Nat Struct Mol Biol. 1998;5(6):432–436. doi:10.1038/nsb0698-432
- Ennifar E, Walter P, Ehresmann B, et al. Crystal structures of coaxially stacked kissing complexes of the HIV-1 RNA dimerization initiation site. Nat Struct Biol. 2001;8(12):1064–1068. doi:10.1038/nsb727
- Barbault F, Huynh-Dinh T, Paoletti J, et al. A new peculiar DNA structure: NMR solution structure of a DNA kissing complex. J Biomol Struct Dyn. 2002;19(4):649–658. doi:10.1080/07391102.2002.10506771
- Kieken F, Paquet F, Brule F, et al. A new NMR solution structure of the SL1 HIV-1 lai loop–loop dimer. Nucleic Acids Res. 2006;34(1):343–352. doi:10.1093/nar/gkj427
- Ennifar E, Dumas P. Polymorphism of bulged-out residues in HIV-1 RNA DIS kissing complex and structure comparison with solution studies. J Mol Biol. 2006;356(3):771–782. doi:10.1016/j.jmb.2005.12.022
- Ennifar E, Paillart J-C, Bodlenner A, et al. Targeting the dimerization initiation site of HIV-1 RNA with aminoglycosides: from crystal to cell. Nucleic Acids Res. 2006;34(8):2328–2339. doi:10.1093/nar/gkl317
- Kieken F, Arnoult E, Barbault F, et al. HIV-1Lai genomic RNA: combined used of NMR and molecular dynamics simulation for studying the structure and internal dynamics of a mutated SL1 hairpin. European Biophys J. 2002;31(7):521–531. doi: 10.1007/s00249-002-0251-1
- Amarasinghe GK, De Guzman RN, Turner RB, et al. NMR structure of the HIV-1 nucleocapsid protein bound to stem-loop SL2 of the Ψ-RNA packaging signal. Implications for genome recognition. J Mol Biol. 2000;301(2):491–511. doi:10.1006/jmbi.2000.3979
- De Guzman RN, Zheng Rong W, Stalling CC, et al. Structure of the HIV-1 nucleocapsid protein bound to the SL3 Ψ-RNA recognition element. Science. 1998;279(5349):384–388. doi:10.1126/science.279.5349.384
- Comas-Garcia M, Davis SR, Rein A. On the selective packaging of genomic RNA by HIV-1. Viruses. 2016;8(9):246. doi:10.3390/v8090246
- Liqing Y, Gribling-Burrer A-S, Bohn P, et al. Short-and long-range interactions in the HIV-1 5ʹ UTR regulate genome dimerization and packaging. Nat Struct Mol Biol. 2022;29(4):306–319. doi:10.1038/s41594-022-00746-2
- Vamva E, Griffiths A, Vink CA, et al. A novel role for gag as a cis-acting element regulating RNA structure, dimerization and packaging in HIV-1 lentiviral vectors. Nucleic Acids Res. 2022;50(1):430–448. doi:10.1093/nar/gkab1206
- D’Souza AR, Jayaraman D, Long Z, et al. HIV-1 packaging visualised by in-gel SHAPE. Viruses. 2021;13(12):2389. doi:10.3390/v13122389
- Blakemore RJ, Burnett C, Swanson C, et al. Stability and conformation of the dimeric HIV-1 genomic RNA 5ʹ UTR. Biophys J. 2021;120(21):4874–4890. doi:10.1016/j.bpj.2021.09.017
- Skripkin E, Paillart J-C, Marquet R, et al. Identification of the primary site of the human immunodeficiency virus type 1 RNA dimerization in vitro. Proc Natl Acad Sci, USA. 1994;91(11):4945–4949. doi:10.1073/pnas.91.11.4945
- Ganser LR, Al-Hashimi HM. HIV-1 leader RNA dimeric interface revealed by NMR. Proc Natl Acad Sci, USA. 2016;113(47):13263–13265. doi:10.1073/pnas.1615789113
- Keane SC, Van V, Frank HM, et al. NMR detection of intermolecular interaction sites in the dimeric 5ʹ-leader of the HIV-1 genome. Proc Natl Acad Sci, USA. 2016;113(46):13033–13038. doi:10.1073/pnas.1614785113
- Mujeeb A, Parslow TG, Zarrinpar A, et al. NMR structure of the mature dimer initiation complex of HIV-1 genomic RNA. FEBS Lett. 1999;458(3):387–392. doi:10.1016/S0014-5793(99)01183-7
- Brigham BS, Kitzrow JP, Reyes J-PC, et al. Intrinsic conformational dynamics of the HIV-1 genomic RNA 5ʹ UTR. Proc Natl Acad Sci, USA. 2019;116(21):10372–10381. doi:10.1073/pnas.1902271116
- Nikolaitchik OA, Somoulay X, Rawson JM, et al. Unpaired guanosines in the 5′ untranslated region of HIV-1 RNA act synergistically to mediate genome packaging. J Virol. 2020;94(21):e00439–20. doi:10.1128/JVI.00439-20
- Berkhout B, Van Wamel JL. The leader of the HIV-1 RNA genome forms a compactly folded tertiary structure. RNA. 2000;6(2):282–295. doi:10.1017/S1355838200991684
- Huthoff H, Berkhout BEN. Two alternating structures of the HIV-1 leader RNA. RNA. 2001;7(1):143–157. doi:10.1017/S1355838201001881
- Mailler E, Bernacchi S, Marquet R, et al. The life-cycle of the HIV-1 Gag–RNA complex. Viruses. 2016;8(9):248. doi:10.3390/v8090248
- Paillart J-C, Dettenhofer M, Xiao-Fang Y, et al. First snapshots of the HIV-1 RNA structure in infected cells and in virions. J Biol Chem. 2004;279(46):48397–48403. doi:10.1074/jbc.M408294200
- Ding P, Kharytonchyk S, Kuo N, et al. 5ʹ-Cap sequestration is an essential determinant of HIV-1 genome packaging. Proc Natl Acad Sci, USA. 2021;118(37):e2112475118. doi:10.1073/pnas.2112475118
- Kroupa T, Datta SA, Rein A. Distinct contributions of different domains within the HIV-1 Gag polyprotein to specific and nonspecific interactions with RNA. Viruses. 2020;12(4):394. doi:10.3390/v12040394
- Comas-Garcia M, Datta SA, Baker L, et al. Dissection of specific binding of HIV-1 Gag to the ’packaging signal’ in viral RNA. Elife. 2017;6:e27055. doi:10.7554/eLife.27055
- Rye-McCurdy T, Olson ED, Liu S, et al. Functional equivalence of retroviral MA domains in facilitating psi RNA binding specificity by Gag. Viruses. 2016;8(9):256. doi:10.3390/v8090256
- Kharytonchyk S, Brown JD, Stilger K, et al. Influence of gag and RRE sequences on HIV-1 RNA packaging signal structure and function. J Mol Biol. 2018;430(14):2066–2079. doi:10.1016/j.jmb.2018.05.029
- Boeras I, Seufzer B, Brady S, et al. The basal translation rate of authentic HIV-1 RNA is regulated by 5 ’UTR nt-pairings at junction of R and U5. Sci Rep. 2017;7(1):6902. doi:10.1038/s41598-017-06883-9
- Ferrer M, Clerté C, Chamontin C, et al. Imaging HIV-1 RNA dimerization in cells by multicolor super-resolution and fluctuation microscopies. Nucleic Acids Res. 2016;44(16):7922–7934. doi:10.1093/nar/gkw511
- Chen J, Abdul Rahman S, Nikolaitchik OA, et al. HIV-1 RNA genome dimerizes on the plasma membrane in the presence of Gag protein. Proc Natl Acad Sci, USA. 2016;113(2):E201–E208. doi:10.1073/pnas.1518572113
- Carlson L-A, Bai Y, Keane SC, et al. Reconstitution of selective HIV-1 RNA packaging in vitro by membrane-bound Gag assemblies. Elife. 2016;5:e14663. doi:10.7554/eLife.14663
- Duchon A, Santos S, Chen J, et al. Plasma membrane anchoring and Gag: gag multimerization on viral RNA are critical properties of HIV-1 Gag required to mediate efficient genome packaging. MBio. 2021;12(6):e03254–21. doi:10.1128/mbio.03254-21
- Yang Y, Qu N, Tan J, et al. Roles of Gag-RNA interactions in HIV-1 virus assembly deciphered by single-molecule localization microscopy. Proc Natl Acad Sci, USA. 2018;115(26):6721–6726. doi:10.1073/pnas.1805728115
- Chen S, Jun X, Mingyue Liu AR, et al. Investigation of HIV-1 Gag binding with RNAs and lipids using atomic force microscopy. PLoS One. 2020;15(2):e0228036. doi:10.1371/journal.pone.0228036
- Bernacchi S, Abd El-Wahab EW, Dubois N, et al. HIV-1 Pr55Gag binds genomic and spliced RNAs with different affinity and stoichiometry. RNA Biol. 2017;14(1):90–103. doi:10.1080/15476286.2016.1256533
- Gilmer O, Mailler E, Paillart J-C, et al. Structural maturation of the HIV-1 RNA 5ʹ untranslated region by Pr55Gag and its maturation products. RNA Biol. 2022;19(1):191–205. doi:10.1080/15476286.2021.2021677
- Tanwar HS, Khoo KK, Garvey M, et al. The thermodynamics of Pr55Gag-RNA interaction regulate the assembly of HIV. PLOS Pathog. 2017;13(2):e1006221. doi:10.1371/journal.ppat.1006221
- Sakuragi S, Yokoyama M, Shioda T, et al. SL1 revisited: functional analysis of the structure and conformation of HIV-1 genome RNA. Retrovirology. 2016;13(1):1–13. doi: 10.1186/s12977-016-0310-9
- Freed EO. HIV-1 assembly, release and maturation. Nat Rev Microbiol. 2015;13(8):484–496. doi:10.1038/nrmicro3490
- Kessl JJ, Kutluay SB, Townsend D, et al. HIV-1 integrase binds the viral RNA genome and is essential during virion morphogenesis. Cell. 2016;166(5):1257–1268. doi: 10.1016/j.cell.2016.07.044
- Elliott JL, Eschbach JE, Koneru PC, et al. Integrase-RNA interactions underscore the critical role of integrase in HIV-1 virion morphogenesis. Elife. 2020;9:e54311. doi:10.7554/eLife.54311
- Shema Mugisha C, Dinh T, Kumar A, et al. Emergence of compensatory mutations reveals the importance of electrostatic interactions between HIV-1 integrase and genomic RNA. MBio. 2022;13(5):e00431–22. doi:10.1128/mbio.00431-22
- Liu S, Koneru PC, Wen L, et al. HIV-1 integrase binding to genomic RNA 5ʹ-UTR induces local structural changes in vitro and in virio. Retrovirology. 2021;18:1–16. doi:10.1186/s12977-021-00582-0
- Wang X-W, Liu C-X, Chen L-L, et al. RNA structure probing uncovers RNA structure-dependent biological functions. Nat Chem Biol. 2021;17(7):755–766. doi:10.1038/s41589-021-00805-7
- Ganser LR, Lee J, Rangadurai A, et al. High-performance virtual screening by targeting a high-resolution RNA dynamic ensemble. Nat Struct Mol Biol. 2018;25(5):425–434. doi:10.1038/s41594-018-0062-4
- Leulliot N, Varani G. Current topics in RNA-protein recognition: control of specificity and biological function through induced fit and conformational capture. Biochemistry. 2001;40(27):7947–7956. doi:10.1021/bi010680y
- Dethoff EA, Chugh J, Mustoe AM, et al. Functional complexity and regulation through RNA dynamics. Nature. 2012;482(7385):322–330. doi:10.1038/nature10885
- Halder S, Bhattacharyya D. RNA structure and dynamics: a base pairing perspective. Prog Biophys Mol Biol. 2013;113(2):264–283. doi:10.1016/j.pbiomolbio.2013.07.003
- Mustoe AM, Brooks CL, Al-Hashimi HM. Hierarchy of RNA functional dynamics. Annu Rev Biochem. 2014;83(1):441–466. doi: 10.1146/annurev-biochem-060713-035524
- Merriman DK, Xue Y, Yang S, et al. Shortening the HIV-1 TAR RNA bulge by a single nucleotide preserves motional modes over a broad range of time scales. Biochemistry. 2016;55(32):4445–4456. doi:10.1021/acs.biochem.6b00285
- Shi H, Rangadurai A, Abou Assi H, et al. Rapid and accurate determination of atomistic RNA dynamic ensemble models using NMR and structure prediction. Nat Commun. 2020;11(1):5531. doi:10.1038/s41467-020-19371-y
- Mustoe AM, Al-Hashimi HM, Brooks CL III. Coarse grained models reveal essential contributions of topological constraints to the conformational free energy of RNA bulges. J Phys Chem B. 2014;118(10):2615–2627. doi:10.1021/jp411478x
- Pyle A. Metal ions in the structure and function of RNA. J Biol Inorg Chem. 2002;7(7–8):679–690. doi: 10.1007/s00775-002-0387-6
- Marušič M, Toplishek M, Plavec J. NMR of RNA-Structure and interactions. Curr Opin Struct Biol. 2023;79:102532. doi:10.1016/j.sbi.2023.102532
- Bing L, Cao Y, Westhof E, et al. Advances in RNA 3D structure modeling using experimental data. Front Genet. 2020;11:574485. doi:10.3389/fgene.2020.574485
- Ganser LR, Chu C-C, Bogerd HP, et al. Probing RNA conformational equilibria within the functional cellular context. Cell Rep. 2020;30(8):2472–2480. doi:10.1016/j.celrep.2020.02.004
- Clay MC, Ganser LR, Merriman DK, et al. Resolving sugar puckers in RNA excited states exposes slow modes of repuckering dynamics. Nucleic Acids Res. 2017;45(14):e134–e134. doi:10.1093/nar/gkx525
- Andreas Juen M, Hermann Wunderlich C, Nußbaumer F, et al. Excited states of nucleic acids probed by proton relaxation dispersion NMR spectroscopy. Angew Chem. 2016;128(39):12187–12191. doi:10.1002/ange.201605870
- Tian S, Cordero P, Kladwang W, et al. High-throughput mutate-map-rescue evaluates SHAPE-directed RNA structure and uncovers excited states. RNA. 2014;20(11):1815–1826. doi:10.1261/rna.044321.114
- Abou Assi H, Rangadurai AK, Shi H, et al. 2ʹ-O-Methylation can increase the abundance and lifetime of alternative RNA conformational states. Nucleic Acids Res. 2020;48(21):12365–12379. doi:10.1093/nar/gkaa928
- Shi X, Walker P, Harbury PB, et al. Determination of the conformational ensemble of the TAR RNA by X-ray scattering interferometry. Nucleic Acids Res. 2017;45(8):e64–e64. doi:10.1093/nar/gkw1352
- Walder R, Van Patten WJ, Ritchie DB, et al. High-precision single-molecule characterization of the folding of an HIV RNA hairpin by atomic force microscopy. Nano Lett. 2018;18(10):6318–6325. doi:10.1021/acs.nanolett.8b02597
- Hermann T, Patel DJ. RNA bulges as architectural and recognition motifs. Structure. 2000;8(3):R47–R54. doi:10.1016/S0969-2126(00)00110-6
- Batey RT, Rambo RP, Doudna JA. Tertiary motifs in RNA structure and folding. Angew Chem Int Ed Engl. 1999;38(16):2326–2343. doi:10.1002/(SICI)1521-3773(19990816)38:16<2326:AID-ANIE2326>3.0.CO;2-3
- Merriman DK, Yuan J, Shi H, et al. Increasing the length of poly-pyrimidine bulges broadens RNA conformational ensembles with minimal impact on stacking energetics. RNA. 2018;24(10):1363–1376. doi:10.1261/rna.066258.118
- Sbicca L, López González A, Gresika A, et al. Exploring the impact of the side-chain length on peptide/RNA binding events. Phys Chem Chem Phys. 2017;19(28):18452–18460. doi:10.1039/C7CP03726K
- Andrałojć W, Ravera E, Salmon L, et al. Inter-helical conformational preferences of HIV-1 TAR-RNA from maximum occurrence analysis of NMR data and molecular dynamics simulations. Phys Chem Chem Phys. 2016;18(8):5743–5752. doi:10.1039/C5CP03993B
- Bennasser Y, Shu-Yun L, Lung Yeung M, et al. HIV-1 encoded candidate micro-RNAs and their cellular targets. Retrovirology. 2004;1(1):1–5. doi:10.1186/1742-4690-1-43
- Harwig A, Jongejan A, van Kampen AH, et al. Tat-dependent production of an HIV-1 TAR-encoded miRNA-like small RNA. Nucleic Acids Res. 2016;44(9):4340–4353. doi:10.1093/nar/gkw167
- Kelly ML, Chu C-C, Shi H, et al. Understanding the characteristics of nonspecific binding of drug-like compounds to canonical stem–loop RNAs and their implications for functional cellular assays. RNA. 2021;27(1):12–26. doi:10.1261/rna.076257.120
- Eubanks CS, Forte JE, Kapral GJ, et al. Small molecule-based pattern recognition to classify RNA structure. J Am Chem Soc. 2017;139(1):409–416. doi:10.1021/jacs.6b11087
- Bagnolini G, Luu TB, Hargrove AE. Recognizing the power of machine learning and other computational methods to accelerate progress in small molecule targeting of RNA. RNA. 2023;29(4):473–488. doi:10.1261/rna.079497.122
- Patwardhan NN, Ganser LR, Kapral GJ, et al. Amiloride as a new RNA-binding scaffold with activity against HIV-1 TAR. MedChemcomm. 2017;8(5):1022–1036. doi:10.1039/C6MD00729E
- Patwardhan NN, Cai Z, Umuhire Juru A, et al. Driving factors in amiloride recognition of HIV RNA targets. Org Biomol Chem. 2019;17(42):9313–9320. doi:10.1039/C9OB01702J
- Alexander D MacKerell NB Jr, Foloppe N, Foloppe N. Development and current status of the CHARMM force field for nucleic acids. Biopolymers. 2000;56(4):257–265. doi:10.1002/1097-0282(2000)56:4<257:AID-BIP10029>3.0.CO;2-W
- Denning EJ, Deva Priyakumar U, Nilsson L, et al. Impact of 2ʹ-hydroxyl sampling on the conformational properties of RNA: update of the CHARMM all-atom additive force field for RNA. J Comput Chem. 2011;32(9):1929–1943. doi:10.1002/jcc.21777
- Cornell WD, Cieplak P, Bayly CI, et al. A second generation force field for the simulation of proteins, nucleic acids, and organic molecules. J Am Chem Soc. 1995;117(19):5179–5197. doi:10.1021/ja00124a002
- Cheatham TE III, Cieplak P, Kollman PA. A modified version of the Cornell et al. force field with improved sugar pucker phases and helical repeat. J Biomol Struct Dyn. 1999;16(4):845–862. doi:10.1080/07391102.1999.10508297
- Pérez A, Marchán I, Svozil D, et al. Refinement of the AMBER force field for nucleic acids: improving the description of α/γ conformers. Biophys J. 2007;92(11):3817–3829. doi:10.1529/biophysj.106.097782
- Banás P, Hollas D, Zgarbová M, et al. Performance of molecular mechanics force fields for RNA simulations: stability of UUCG and GNRA hairpins. J Chem Theory Comput. 2010;6(12):3836–3849. doi:10.1021/ct100481h
- Zgarbová M, Otyepka M, Sponer J, et al. Refinement of the Cornell et al. nucleic acids force field based on reference quantum chemical calculations of glycosidic torsion profiles. J Chem Theory Comput. 2011;7(9):2886–2902. doi:10.1021/ct200162x
- Aytenfisu AH, Spasic A, Grossfield A, et al. Revised RNA dihedral parameters for the amber force field improve RNA molecular dynamics. J Chem Theory Comput. 2017;13(2):900–915. doi:10.1021/acs.jctc.6b00870
- Suresh G, Deva Priyakumar U. Atomistic details of the molecular recognition of DNA-RNA hybrid duplex by ribonuclease H enzyme. J Chem Sci. 2015;127(10):1701–1713. doi: 10.1007/s12039-015-0942-7
- Figiel M, Krepl M, Poznański J, et al. Coordination between the polymerase and RNase H activity of HIV-1 reverse transcriptase. Nucleic Acids Res. 2017;45(6):3341–3352. doi:10.1093/nar/gkx004
- Figiel M, Krepl M, Park S, et al. Mechanism of polypurine tract primer generation by HIV-1 reverse transcriptase. J Biol Chem. 2018;293(1):191–202. doi:10.1074/jbc.M117.798256
- Salmon L, Giambasu GM, Nikolova EN, et al. Modulating RNA alignment using directional dynamic kinks: application in determining an atomic-resolution ensemble for a hairpin using NMR residual dipolar couplings. J Am Chem Soc. 2015;137(40):12954–12965. doi:10.1021/jacs.5b07229
- Réblová K, Spackova N, Sponer JE, et al. Molecular dynamics simulations of RNA kissing-loop motifs reveal structural dynamics and formation of cation-binding pockets. Nucleic Acids Res. 2003;31(23):6942–6952. doi:10.1093/nar/gkg880
- Réblová K, Fadrná E, Sarzynska J, et al. Conformations of flanking bases in HIV-1 RNA DIS kissing complexes studied by molecular dynamics. Biophys J. 2007;93(11):3932–3949. doi:10.1529/biophysj.107.110056
- Havrila M, Zgarbová M, Jurecka P, et al. Microsecond-scale MD simulations of HIV-1 DIS kissing-loop complexes predict bulged-in conformation of the bulged bases and reveal interesting differences between available variants of the AMBER RNA force fields. J Phys Chem B. 2015;119(49):15176–15190. doi:10.1021/acs.jpcb.5b08876
- Michael LA, Chenault JA, Miller BR III, et al. Water, shape recognition, salt bridges, and cation–pi interactions differentiate peptide recognition of the HIV Rev-responsive element. J Mol Biol. 2009;392(3):774–786. doi:10.1016/j.jmb.2009.07.047
- Nifosì R, Reyes CM, Kollman PA. Molecular dynamics studies of the HIV-1 TAR and its complex with argininamide. Nucleic Acids Res. 2000;28(24):4944–4955. doi:10.1093/nar/28.24.4944
- Reyes CM, Nifosì R, Frankel AD, et al. Molecular dynamics and binding specificity analysis of the bovine immunodeficiency virus biv tat-tar complex. Biophys J. 2001;80(6):2833–2842. doi:10.1016/S0006-3495(01)76250-9
- Yuguang M, Stock G. Conformational dynamics of RNA-peptide binding: a molecular dynamics simulation study. Biophys J. 2006;90(2):391–399. doi:10.1529/biophysj.105.069559
- Frank AT, Stelzer AC, Al-Hashimi HM, et al. Constructing RNA dynamical ensembles by combining MD and motionally decoupled NMR RDCs: new insights into RNA dynamics and adaptive ligand recognition. Nucleic Acids Res. 2009;37(11):3670–3679. doi:10.1093/nar/gkp156
- Mori M, Dietrich U, Manetti F, et al. Molecular dynamics and DFT study on HIV-1 nucleocapsid protein-7 in complex with viral genome. J Chem Inf Model. 2010;50(4):638–650. doi:10.1021/ci100070m
- Musselman C, Zhang Q, Al-Hashimi H, et al. Referencing strategy for the direct comparison of nuclear magnetic resonance and molecular dynamics motional parameters in RNA. J Phys Chem B. 2010;114(2):929–939. doi:10.1021/jp905286h
- Sethaphong L, Singh A, Marlowe AE, et al. The sequence of HIV-1 TAR RNA helix controls cationic distribution. J Phys Chem C. 2010;114(12):5506–5512. doi:10.1021/jp906147q
- Salmon L, Bascom G, Andricioaei I, et al. A general method for constructing atomic-resolution RNA ensembles using NMR residual dipolar couplings: the basis for interhelical motions revealed. J Am Chem Soc. 2013;135(14):5457–5466. doi:10.1021/ja400920w
- Musiani F, Rossetti G, Capece L, et al. Molecular dynamics simulations identify time scale of conformational changes responsible for conformational selection in molecular recognition of HIV-1 transactivation responsive RNA. J Am Chem Soc. 2014;136(44):15631–15637. doi:10.1021/ja507812v
- Do TN, Carloni P, Varani G, et al. RNA/Peptide binding driven by electrostatics — insight from bidirectional pulling simulations. J Chem Theory Comput. 2013;9(3):1720–1730. doi:10.1021/ct3009914
- Chun Hua L, Cheng Zuo Z, Ji Guo S, et al. The interactions and recognition of cyclic peptide mimetics of Tat with HIV-1 TAR RNA: a molecular dynamics simulation study. J Biomol Struct Dyn. 2013;31(3):276–287. doi:10.1080/07391102.2012.698248
- Ronsard L, Rai T, Rai D, et al. In silico analyses of subtype specific HIV-1 Tat-TAR RNA interaction reveals the structural determinants for viral activity. Front Microbiol. 2017;8:1467. doi:10.3389/fmicb.2017.01467
- Ronsard L, Ganguli N, Singh VK, et al. Impact of genetic variations in HIV-1 Tat on LTR-mediated transcription via TAR RNA interaction. Front Microbiol. 2017;8:706. doi:10.3389/fmicb.2017.00706
- Zhang B-G, Qiu H-H, Jiang J, et al. 3D structure stability of the HIV-1 TAR RNA in ion solutions: a coarse-grained model study. J Chem Phys. 2019;151(16):165101. doi:10.1063/1.5126128
- Levintov L, Vashisth H. Ligand recognition in viral RNA necessitates rare conformational transitions. J Phys Chem Lett. 2020;11(14):5426–5432. doi:10.1021/acs.jpclett.0c01390
- Henning-Knechtel A, Thirumalai D, Kirmizialtin S. Differences in ion-RNA binding modes due to charge density variations explain the stability of RNA in monovalent salts. Sci Adv. 2022;8(29):eabo1190. doi:10.1126/sciadv.abo1190
- Sponer J, Bussi G, Krepl M, et al. RNA structural dynamics as captured by molecular simulations: a comprehensive overview. Chem Rev. 2018;118(8):4177–4338. doi:10.1021/acs.chemrev.7b00427
- Duss O, Lukavsky PJ, H-T Allain F. Isotope labeling and segmental labeling of larger RNAs for NMR structural studies. Adv Exp Med Biol. 2012;992:121–144.
- Andrew McCammon J, Gelin BR, Karplus M. Dynamics of folded proteins. Nature. 1977;267(5612):585–590. doi:10.1038/267585a0
- Adcock SA, Andrew McCammon J. Molecular dynamics: survey of methods for simulating the activity of proteins. Chem Rev. 2006;106(5):1589–1615. doi:10.1021/cr040426m
- Karplus M, Andrew McCammon J. Molecular dynamics simulations of biomolecules. Nat Struct Biol. 2002;9(9):646–652. doi:10.1038/nsb0902-646
- Paul S, Nair NN, Vashisth H. Phase space and collective variable based simulation methods for studies of rare events. Mol Simul. 2019;45(14–15):1273–1284. doi:10.1080/08927022.2019.1634268
- Vashisth H, Skiniotis G, Brooks CL III. Collective variable approaches for single molecule flexible fitting and enhanced sampling. Chem Rev. 2014;114(6):3353–3365. doi:10.1021/cr4005988
- Iwazaki T, Xianglan L, Harada K. Evolvability of the mode of peptide binding by an RNA. RNA. 2005;11(9):1364–1373. doi:10.1261/rna.2560905
- Xiaojun X, Jin L, Xie L, et al. Landscape zooming toward the prediction of RNA cotranscriptional folding. J Chem Theory Comput. 2022;18(3):2002–2015. doi:10.1021/acs.jctc.1c01233
- Jurich CP, Brivanlou A, Rouskin S, et al. Web-based platform for analysis of RNA folding from high throughput chemical probing data. Nucleic Acids Res. 2022;50(W1):W266–W271. doi:10.1093/nar/gkac435
- Xie J, Frank AT. Mining for ligandable cavities in RNA. ACS Med Chem Lett. 2021;12(6):928–934. doi:10.1021/acsmedchemlett.1c00068
- Kmiecik S, Gront D, Kolinski M, et al. Coarse-grained protein models and their applications. Chem Rev. 2016;116(14):7898–7936. doi:10.1021/acs.chemrev.6b00163
- Goodsell DS, Jewett A, Olson AJ, et al. Integrative modeling of the HIV-1 ribonucleoprotein complex. PLoS Comput Biol. 2019;15(6):e1007150. doi:10.1371/journal.pcbi.1007150