Abstract
Per- and polyfluoroalkyl substances (PFASs) are a large class of compounds used in a variety of processes and consumer products. Their unique chemical properties make them ubiquitous and persistent environmental contaminants while also making them economically viable and socially convenient. To date, several reviews have been published to synthesize information regarding the immunotoxic effects of PFASs on the adaptive immune system. However, these reviews often do not include data on the impact of these compounds on innate immunity. Here, current literature is reviewed to identify and incorporate data regarding the effects of PFASs on innate immunity in humans, experimental models, and wildlife. Known mechanisms by which PFASs modulate innate immune function are also reviewed, including disruption of cell signaling, metabolism, and tissue-level effects. For PFASs where innate immune data are available, results are equivocal, raising additional questions about common mechanisms or pathways of toxicity, but highlighting that the innate immune system within several species can be perturbed by exposure to PFASs. Recommendations are provided for future research to inform hazard identification, risk assessment, and risk management practices for PFASs to protect the immune systems of exposed organisms as well as environmental health.
Introduction
Per- and polyfluoroalkyl substances (PFAS) are a large class of fluorinated compounds that are used in the production of nonstick cookware, food contact materials, water-, oil-, and stain-resistant fabrics and coatings, and aqueous film-forming foams (AFFF), among other products (reviewed in Glüge et al. Citation2020). PFAS are defined by the Organization for Economic Co-operation and Development (OECD) as consisting ‘of a fully (per) or partly (poly) fluorinated carbon chain connected to different functional groups’ (Buck et al. Citation2011; Wang, Buser, et al. Citation2021). This class of compounds is incredibly diverse, with nearly 5000 different PFASs registered globally, according to an OECD database (Organization for Economic Co-operation and Development (OECD) Citation2018). However, an expansive list from the United States Environmental Protection Agency (USEPA) greatly expands this number to more than 14,000 compounds when other chemical moieties containing a carbon-fluorine bond are considered (Williams et al. Citation2017; USEPA Citation2023a). A subset of roughly 1200 PFAS appear in the United States Toxic Substances Control Act (TSCA) Chemical Inventory, with > 600 described as commercially active (USEPA Citation2019).
PFAS entered large-scale production in the 1950s, and at the time, the most common PFAS intentionally produced were perfluorooctanoic acid (PFOA) and perfluorooctane sulfonic acid (PFOS) (Buck et al. Citation2011; Lindstrom et al. Citation2011). However, as more studies observed the toxicities exerted by these two ‘long-chain legacy’ PFAS, a subset of fluorochemical manufacturers sought, in part due to a PFOA Stewardship Agreement with USEPA, to phase out these compounds with novel replacements with fewer carbons (i.e., ‘short-chain’) that were thought to possess more favorable toxicity profiles (USEPA Citation2023b). However, these alternatives were relatively untested before entering the market, and the manufacture of some of these replacement compounds led to the creation of other PFAS as by-products (Hopkins et al. Citation2018). Therefore, even though emissions of long-chain legacy PFAS had decreased, emissions of new, short-chain replacement PFAS, their by-products, and their degradants have now increased (Hopkins et al. Citation2018). Notable members of these new PFAS include a sub-family known as per- and polyfluorinated ether acids (PFEA), which possess ether linkages in the carbon backbone of the molecule (Hopkins et al. Citation2018; Cousins et al. Citation2020). While some studies of how these ether linkages impact persistence, bioaccumulation, and toxicity have been performed, many are ongoing. Structures of the PFAS discussed in this review are presented in .
Figure 1. Chemical structures of PFAS discussed in this review. Structures were made using ChemDraw professional (v16.0.1.4) and standard international union of pure and applied chemistry (IUPAC) nomenclature. Structures are organized according to a previously described scheme (Wang et al. Citation2017).
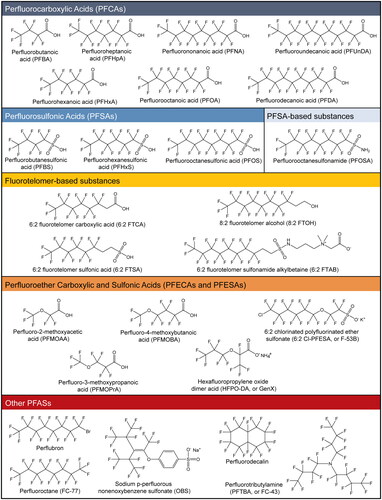
Exposure to PFAS can occur through multiple routes. Most notably, humans are exposed to PFAS through drinking water that is contaminated from industrial sources, use of AFFF at airports and military bases, and runoff from landfill leachate or land-applied biosolids that contain PFAS (Hu et al. Citation2016; Boone et al. Citation2019; Domingo and Nadal Citation2019). It recently has been estimated that the drinking water of > 200 million Americans is contaminated with PFAS (Andrews and Naidenko Citation2020). Exposure to PFAS also can occur through consumption of contaminated food, inhalation of indoor dust, and dermal exposure with PFAS-treated textiles (Boronow et al. Citation2019; Chen et al. Citation2020; Poothong et al. Citation2020). Fetuses are exposed to PFASs in utero, and infants can be exposed to PFAS through breast milk (Blake and Fenton Citation2020). Given the distinct chemical characteristics of PFAS and their well-documented, widespread exposure, questions have been raised of the hazard and risk that PFASs contribute to human and environmental health.
Others have reviewed the toxicity of these compounds in humans, experimental animal models, and wildlife (DeWitt Citation2015; Agency for Toxic Substances and Disease Registry [ATSDR] 2021; Fenton et al. Citation2021; Bangma et al. Citation2022), which includes liver damage, endocrine disruption, developmental toxicity, and cancer. Of particular interest, many studies have noted the immunotoxicity of these compounds, which also has been reviewed extensively (Chang et al. Citation2016; National Toxicology Program (NTP)) Citation2016; DeWitt et al. Citation2019; Ehrlich et al. Citation2023). Adverse immune-related outcomes associated with exposure to PFASs include ulcerative colitis, allergic sensitization, altered inflammatory processes, and reduced response to vaccinations. Indeed, the immune system appears to be a sensitive target of PFAS, and immunotoxicity has been used to drive risk assessments and statewide regulations across the United States (Cordner et al. Citation2019). In fact, the recent interim health advisory levels for PFOA and PFOS were calculated based on data showing that these compounds have adverse effects on the developing human immune system (USEPA Citation2022). While many recent reviews remain relevant (Chang et al. Citation2016; NTP Citation2016; DeWitt et al. Citation2019; Ehrlich et al. Citation2023; Zhang, Louie, et al. Citation2023), there remains a heightened interest in PFAS-induced immunotoxicity, mechanisms leading to such immunotoxicity, and concerns about understudied PFASs inducing immunotoxicity.
In the present review of the literature (portions of which appeared in the DWP doctoral dissertation [Phelps Citation2022]), however, it is noted that there is a gap in previous reviews that did not consider data indicating that PFAS impact innate immunity. Here, the impacts of PFAS exposure on innate immunity in humans, experimental animal models, and ecotoxicological models and wildlife are reviewed. In doing so, current data are synthesized and data incongruencies examined, revealing where data gaps exist. The toxicity of most PFAS remains unknown, and filling these gaps is necessary to understand their hazard and risk to human and environmental health. There are even larger data gaps with regards to these compounds’ effects on innate immunity, despite it being well-established that several PFAS are immunotoxic. The scope of this review will be limited to previous reports that investigate innate immunity on several fronts: immunophenotyping of innate immune cell populations, changes in concentrations of cytokines relevant to innate immunity, and functional assays of innate immune cells. Finally, an attempt is made to glean what the reviewed studies reveal about potential mechanisms of PFAS-induced immunotoxicity. The goal of this review is to expand the knowledge base of what is known about PFAS in an oft-overlooked branch of the immune system when it comes to chemical hazard identification and risk assessment.
Evidence of innate immune modulation by PFASs in humans, primary human leukocytes, and human cell lines
Several studies have sought to understand the impacts of PFAS exposure in the general population as well as populations exposed to high levels of PFAS as a result of large-scale or long-term contamination or occupational exposure (summarized in ). Most reports have investigated the effects of PFAS exposure on adaptive immunity in these populations, but a few have asked essential questions about effects on innate immunity. Immunophenotyping has shown that increasing serum levels of PFOA, PFOS, perfluorodecanoic acid (PFDA), perfluorononanoic acid (PFNA), and perfluorohexane sulfonic acid (PFHxS) are correlated with decreases in innate immune cell populations in blood, including the number of monocytes, neutrophils, eosinophils, basophils, and/or natural killer (NK) cells, though results are not consistent across studies (Emmett et al. Citation2006; Knudsen et al. Citation2018; Goodrich et al. Citation2021; Lopez-Espinosa et al. Citation2021). Zhao et al. (Citation2022) also found a significant correlation between PFOA and PFOS concentrations and leukopenia, i.e. a decrease in white blood cells, in a cohort of rheumatoid arthritis patients. It has been reported that total serum PFASs, when analyzed as a mixture, were linked to increases in the number of basophils in the blood (Oulhote et al. Citation2017). These studies illustrate that several PFASs are able to impact levels of innate immune cells in circulation; however, the lack of consensus in this endpoint makes further conclusions difficult.
Table 1. Summary of innate immune modulation associated with exposure to PFASs in humans, primary human leukocytes, and human cell lines.
Serum cytokines are relatively easy to measure from a technical perspective; however, the impact of PFAS exposure on serum cytokine levels varies across different studies. PFOA has been linked to both decreases and increases in serum levels of interferon (IFN)-γ (Bassler et al. Citation2019; Abraham et al. Citation2020; Nian et al. Citation2022). These observations point to this PFAS having the ability to impact this pathway, but inconsistencies in the directionality of this endpoint make interpretations challenging. Bassler et al. (Citation2019) noted that increased serum concentrations of PFOA, PFOS, PFHxS, and PFNA were associated with decreased tumor necrosis factor (TNF)-α and/or interleukin (IL)-8. The lack of consistent directionality in cytokine levels associated with PFAS exposures may be due to differences in exposure levels and duration, interactions of mixtures of PFAS, interactions with other toxicants, the timing of when cytokine levels were measured, and/or genetic differences in one population to another. It is also unknown how recent infections or other environmental conditions may be shaping these data. It must also be noted that, due to the design of these studies, it is difficult to ascertain from which cellular source cytokines were secreted. Given that epithelial cells and lymphocytes, as well as other cell types, may produce these cytokines, this response may not reflect a direct impact on innate immune cells. More studies are necessary to elucidate the immunological and mechanistic implications from these epidemiological cytokine findings.
In experimental systems, PFAS have been studied to a greater extent because of the flexibility that is offered by human cell lines in vitro and primary cells ex vivo. Many studies to date have focused on cytokine expression at the transcript and protein level. In whole blood and THP-1 cells (a human monocytic leukemia cell line), PFOA, PFOS, perfluorobutane sulfonic acid (PFBS), perfluorooctane sulfonamide (PFOSA), PFDA, and 8:2 fluorotelomer alcohol (FTOH) exposures down-regulated various cytokines, including TNFα, as well as IL-4, −6, 8, and −10 (Brieger et al. Citation2011; Corsini et al. Citation2011, Citation2012). However, PFOA also induced TNFα, as well as IL-6, −8, and -1β in HMC-1 cells, a human mast cell leukemia cell line (Singh et al. Citation2012). Though this may point to differences in susceptibility between monocytic and granulocytic cells following exposure to PFAS, a recent study revealed that the two cell types may share a target, as IL-1β also was up-regulated in THP-1 cells (Wang, Liu, et al. Citation2021).
Functional assays using cellular systems, such as THP-1 and HMC-1 cells, have largely focused on PFAS-mediated disruption of nuclear factor (NF)-κB activation and peroxisome proliferator-activated receptor-α (PPARα) pathways (see below). Other in vitro and ex vivo functional investigations in primary human neutrophils have shown that exposure to perflubron, perfluorodecalin (as an ingredient of Fluosol-DA), perfluorotributylamine (PFTBA), PFHxA, GenX, or mixtures of several PFASs can suppress and activate the production of reactive oxygen species (ROS) (Virmani et al. Citation1984; Berntsen et al. Citation2022; Phelps et al. Citation2023), inhibit phagocytosis (Virmani et al. Citation1984; Fernandez et al. Citation2001), and inhibit chemotaxis (Fernandez et al. Citation2001). NK cell cytolysis was also suppressed by exposure to PFOS (Brieger et al. Citation2011).
While in vitro exposure paradigms often employ high concentrations of test compounds, it must be stated that modeling chronic exposure to persistent, bioaccumulative toxicants in a short-lived experimental model in vitro is often difficult. In the human epidemiological studies described above, serum concentrations of the described PFASs are typically reported within the nanomolar (ng/ml) range, except for Emmett et al. (Citation2006) which reported a single study participant with a serum level of PFOA greater than 4000 ng/ml (∼9.66 µM). The in vitro studies in human cells described above, conversely, work with concentrations up to 80 µM. This may represent a challenge when extrapolating experimental systems to real-world exposure scenarios; however recent advances with in vitro to in vivo extrapolation (IVIVE) may provide a route forward for risk assessment and management of PFAS (Chang et al. Citation2022; Najjar et al. Citation2022). Regardless, the studies described above – epidemiological, in vitro, and ex vivo – illuminate the massive data gaps that remain regarding the effects of PFAS on innate immunity in humans. While variations in outcome measures across epidemiological studies, as well as in vitro and ex vivo experiments, make it difficult to reach firm conclusions about what the affected outcomes mean from a disease or clinical standpoint, it is clear that exposures to a range of PFASs can impact various innate immune parameters in humans.
Evidence of innate immune modulation by PFASs in mammalian models
Effects of PFAS exposure on innate immunity have been investigated in several mammalian model systems. (summarized in ). Exposure to several PFAS, including PFOA, PFOS, PFNA, PFDA, perfluoroundecanoic acid (PFUnDA), PFTBA, perfluorobutanoic acid (PFBA), or perfluoromethoxybutanoic acid (PFMOBA), has been associated with changes in neutrophil, macrophage, and NK cell numbers in blood and tissues of rodent and rabbit models (Virmani et al. Citation1983; Fang et al. Citation2008; Qazi et al. Citation2009, Citation2010a, Citation2010b, Citation2012; Bodin et al. Citation2016; Han et al. Citation2018a, Citation2018b; Frawley et al. Citation2018; NTP Citation2019; Guo et al. Citation2021; Torres et al. Citation2021; Woodlief et al. Citation2021; Weatherly et al. Citation2021). At low doses meant, in part, to recapitulate what has been observed in the general population, PFOS was reported to have no effect on the immunophenotype of various immune compartments in mice (Pierpont et al. Citation2023). In contrast, PFOA exposure was reported to change the number of neutrophils and macrophages in mice; however, while neutrophil decreases were observed in the blood, increases in granulocytes were observed in the liver (Qazi et al. Citation2009, Citation2010a). This indicates immune compartments may be differentially affected by PFAS exposure, potentially due to how PFAS themselves distribute in vivo. Without further study, however, it is difficult to ascertain whether the increase of these innate immune cells in the liver is an inflammatory response caused by damage to the liver or a coincidental pro-inflammatory state caused in the immune cells independent of changes in the liver. Regardless, alterations in immunophenotype remains a sensitive but nonspecific endpoint by which PFASs may alter the immune system.
Table 2. Summary of innate immune modulation by exposure to PFASs in experimental mammals.
As with human studies, cytokine levels are often studied in animal models because they are technically easy to collect and measure from serum and tissues. Recent data have shown that PFOA, PFOS, and PFNA, along with three of their novel alternatives, 8:2 FTOH, 6:2 fluorotelomer carboxylic acid (6:2 FTCA) and 6:2 fluorotelomer sulfonic acid (6:2 FTSA), alter expression levels of TNFα, IFNγ, as well as IL-4, −6, and −10 (Qazi et al. Citation2009, Citation2010a; Rockwell et al. Citation2013, Citation2017; Sheng et al. Citation2017; Han et al. Citation2018a, Citation2018b; Kong et al. Citation2019; Shane et al. Citation2020; Guo et al. Citation2021; Tian et al. Citation2021). Many of these cytokines can be suppressed or increased in response to exposure to different PFAS. Exposure routes as well as immunological compartments matter in assessing the effects of PFAS on the innate immune system. It was observed that after dietary exposure to PFOA or PFOS and challenge with lipopolysaccharide (LPS) in mice, TNFα and IL-6 were increased in the bone marrow and peritoneum; however, TNFα was suppressed in the spleen in PFOS-exposed animals but increased in the spleen in PFOA-exposed animals (Qazi et al. Citation2009). Similarly, a follow-up study from this laboratory reported TNFα, IFNγ, and IL-4 reductions in the liver after PFOA or PFOS exposure in mice (Qazi et al. Citation2010a). In a different laboratory, however, increases of TNFα, IL-1β, and IL-6 were observed in the spleen of mice exposed to PFOS via oral gavage (Dong et al. Citation2011). Dermal exposure to PFOA in mice led to an increase in IL-1β in the skin (Shane et al. Citation2020), albeit this may have resulted from an indirect inflammatory response. Importantly, because these types of studies are conducted in vivo, it is difficult to distinguish which cells are producing these cytokines. As mentioned previously, cytokines can be produced by innate immune cells as well as other cell types including adaptive immune cells and non-immune cells, making it likely that multiple cell types are contributing to the observed phenotypes. More work is needed to elucidate these phenomena, their specificity, and how observed changes in cytokine levels may apply to functional immunological outcomes.
Relatively few functional innate immune outcomes have been investigated using these mammalian models. Exposure to PFDA, PFUnDA, or a mixture of several PFAS was linked to reductions in phagocytosis by macrophages from mice and rats (Bodin et al. Citation2016; Berntsen et al. Citation2018; Frawley et al. Citation2018), whereas exposure to PFOA was linked to increased phagocytosis by macrophages in mice (Guo et al. Citation2021). PFOA and PFOS have also been found to reduce phagocytosis by peripheral blood cells (Vetvicka and Vetvickova Citation2013). Alterations in NK cell cytolysis have been observed after PFOA and PFOS exposure (Keil et al. Citation2008; Peden-Adams et al. Citation2008; Dong et al. Citation2009; Zheng et al. Citation2009), although the directionality of this response appears to be dependent on exposure duration, dose, and PFAS. No changes in NK cytolysis were observed with exposure to novel, short-chain per- and polyfluorinated ether carboxylic acids, perfluoro-methoxyacetic acid (PFMOAA), perfluoromethoxypropanoic acid (PFMOPrA), and PFMOBA in mice (Woodlief et al. Citation2021). While not entirely functional, studies of signal transduction within the NF-κB pathway have been performed after PFAS exposure, with findings ranging from no effect with PFNA in mice (Fang et al. Citation2008), activation of the pathway with PFOA, 6:2 FTCA, 6:2 FTSA in mice and rats (Singh et al. Citation2012; Sheng et al. Citation2017; Han et al. Citation2018a, Citation2018b), and suppression of the pathway with PFOA in mice (Shane et al. Citation2020). Induction of the inflammasome has also been observed in the liver after PFOS exposure in mice (Qin et al. Citation2022). Gene expression studies after PFAS exposure have also provided some insight, resulting in up-regulation of immune-related genes in mouse liver after exposure to PFAS mixtures (Lv et al. Citation2018), up-regulation of damage-associated molecular pattern (DAMP) genes in the skin after exposure to PFBA in mice (Weatherly et al. Citation2021), and down-regulation of macrophage activation genes in rat liver after perfluoroheptanoic acid (PFHpA), PFOA, or PFDA exposure (McMillian et al. Citation2004).
Evidence of innate immune modulation by PFASs in the zebrafish model
One oft-overlooked model that has been used in the experimental investigation of the immunotoxicity of PFASs is the zebrafish (Danio rerio). Zebrafish are an excellent model for toxicology studies due to their small size, external and rapid development, and high fecundity (Planchart et al. Citation2016). A recent review specifically highlighted the utility of the zebrafish model for PFAS-induced toxicity across multiple organ systems (Rericha et al. Citation2023). Furthermore, given that they possess orthologs for 70% of all protein-encoding genes in humans (Howe et al. Citation2013), zebrafish are an excellent model for human health. Immunologically, zebrafish possess all of the same major immune lineages as mammals, allowing for use as a comparative model (Traver et al. Citation2003; Traver and Yoder Citation2020). Their adaptive immune system, however, is not fully functional until ∼3 wk post-fertilization, providing an experimental window when vertebrate innate immunity can be studied in a medium- to high-throughput manner independent from B- and T-lymphocytes.
Larval zebrafish can be used as a screening tool for identifying compounds that are immunotoxic to the innate immune system (Phelps et al. Citation2020). In a recent publication (Phelps et al. Citation2023), this work was expanded upon to screen nine PFASs, and these data were recapitulated using a human cell line and primary human cells, indicating there are conserved mechanisms of PFAS-induced immunotoxicity across species. This validates the use of zebrafish for translational immunotoxicity studies, especially for PFAS. Despite this, zebrafish have been under-utilized as a model for the study of PFAS immunotoxicity (summarized in ). Most studies in zebrafish to date have focused on cytokine production at the transcript level, reporting that levels of various cytokines, including IL-6, IL-8, IL-10, IL-1β, TNFα, and IFNΦ1 [a well-studied interferon in zebrafish (Altmann et al. Citation2003; Aggad et al. Citation2009)] have either increased or decreased in response to PFOA, PFOS, 6:2 FTSA, 6:2 fluorotelomer sulfonamide alkylbetaine (6:2 FTAB), F-53B, or sodium p-perfluorous nonenoxybenzene sulfonate (OBS) exposure (Liu et al. Citation2013, Citation2021; Zhang et al. Citation2014; Shi et al. Citation2018; Guo et al. Citation2021; Huang et al. Citation2021; Zhang, Ren, et al. Citation2023). Transcriptomic studies after PFOA, PFOS, or 6:2 FTSA exposure using zebrafish have been performed and showed similar results, along with alterations in pathways related to innate immunity, inflammation, and infection (Martínez et al. Citation2019; Yu et al. Citation2022; Zhang, Ren, et al. Citation2023), and these findings were supported by studies of the NF-κB pathway after exposure to PFOA, PFOS, or F-53B, a novel PFOS replacement (Zhang et al. Citation2014; Guo et al. Citation2019; Yang et al. Citation2020).
Table 3. Summary of innate immune modulation by exposure to PFASs in zebrafish.
Functional assays in zebrafish are limited but nevertheless illuminating. Exposure to PFOA in larval zebrafish reduced neutrophil chemotaxis to a wound site (Pecquet et al. Citation2020). PFOS exposure induced granulomatous lesions in the liver (Keiter et al. Citation2012), indicating likely immune dysregulation. PFOS also has been shown to increase ROS production in adult zebrafish liver through an increase in myeloperoxidase activity, which also was accompanied by increased lysozyme activity (Guo et al. Citation2019); however, this response may be due to an inflammatory response and recruitment of neutrophils. F-53B also induced ROS production in zebrafish larvae, including nitric oxide (Liu et al. Citation2021). In contrast, recently published work indicates that PFOS exposure does not alter ROS production in zebrafish larvae at some concentrations administered (Phelps et al. Citation2023). PFHxA and GenX suppressed ROS production in larval zebrafish, which was then validated using a human neutrophil-like cell line (Phelps et al. Citation2023).
It must be noted that, in these functional assessments, a wide range of test concentrations have been used. Chronic or subchronic studies in adult zebrafish used concentrations in the nanomolar range, while studies of zebrafish larvae utilized concentrations up to 80 µM. Modeling the toxicity of persistent, bioaccumulative compounds for human health with a short exposure time in larval zebrafish is difficult. As discussed above with extrapolation between in vitro studies to in vivo studies for humans, similar challenges exist to extrapolate between species. The toxicokinetics of PFAS in the zebrafish model are not fully understood and present a data gap for future exploration. Direct comparisons of serum levels to those in human populations may be difficult; in addition to species-specific differences, zebrafish are also typically exposed to test compounds via immersion, which may allow for uptake of test compounds through oral, dermal, and/or branchial routes. Even still, recent studies have sought to elucidate the uptake of PFASs in larval zebrafish (Han et al. Citation2021), and the known toxicokinetics of these compounds were recently reviewed (Rericha et al. Citation2023).
Regardless, experimental animals modeling human disease, from rodents to zebrafish, have been useful in understanding hazards posed to innate immunity by exposure to PFAS. More research is needed to resolve conflicting data from these models and to confirm these models reasonably reflect the impact of PFAS exposure on human immune response. Additional functional assays are also necessary to further elucidate immunotoxic outcomes after exposure to PFAS with little toxicity data.
Evidence of innate immune modulation by PFASs in wildlife and ecotoxicological models
Finally, the limited amount of data regarding innate immunity in wildlife and ecotoxicological models provides a glimpse into the toxicity induced by PFAS (summarized in ). Wildlife not only provide insight into environmental health, but can also serve as sentinels for human health, as they often are developmentally and/or chronically exposed to similar concentrations of persistent and ubiquitous environmental contaminants. To date, PFAS-induced immunotoxicity in these models has not been extensively reviewed.
Table 4. Summary of innate immune modulation by exposure to PFASs in wildlife and ecotoxicological models.
In a population of bottlenose dolphins (Tursiops truncatus), increasing serum levels of PFAS were linked to decreases in circulating eosinophils but increases in phagocytosis by granulocytes and monocytes (Fair et al. Citation2013). Increased lysozyme activity has been observed both in striped bass (Morone saxatilis) (Guillette et al. Citation2020) and white leghorn chickens (Gallus gallus domesticus) (Peden-Adams et al. Citation2009) with increasing PFOS serum levels. Similarly, increasing serum concentrations of PFAS in American alligators (Alligator mississippiensis) were associated with increased lysozyme activity and serum complement activity (Guillette et al. Citation2022). White-tailed eagles (Haliaeetus albicilla) with elevated PFHxS and total PFAS levels also had suppressed ROS production in a leukocyte respiratory burst assay (Hansen et al. Citation2020). Transcriptomic analyses of wild fish species have shown altered expression of innate immune genes after exposure to PFOS or a mixture of several PFAS (Rodríguez-Jorquera et al. Citation2019; Zhang et al. Citation2020), although the directionality of these responses varies among studies and genes of interest. The lack of data regarding PFAS exposure and innate immunity in wildlife reveals how much work remains to be done to understand the ramifications of PFAS contamination on ecological health.
Molecular insights into PFAS-induced immunotoxicity in innate immune cells
One major question remaining unanswered is how, on a molecular level, PFAS disrupt innate immune function. A variety of studies in recent years, on both the cellular and tissue level, have allowed molecular pathways to emerge. Many of these utilized non-targeted approaches, such as transcriptomics and metabolomics, while others probed specific immune pathways, such as the NF-κB pathway discussed below. Of the more than 25 studies found addressing how PFAS disrupt innate immune signaling pathways on the cellular level, more than half focused on macrophages, monocytes, or microglia. For these three cell types, graphics were created summarizing the molecular disruptions found due to PFAS exposure (), along with a second set of graphics summarizing innate immune mechanisms suggested by other studies (e.g. those done with liver tissue or whole blood) that may also be occurring in innate immune cells (). A table listing the endpoints and studies used to build these figures is provided in Supplemental Table S1. Studies exploring molecular mechanisms of PFAS immunotoxicity specifically in neutrophils, eosinophils, NK cells, or dendritic cells were not found. Further research into effects of PFASs on these/other critical innate immune cells is strongly encouraged.
Figure 2. Visual summary of mechanisms of PFAS toxicity observed in monocytes, microglia, and macrophages. PFAS have been shown to disrupt immune signaling and other pathways in (A) monocytes (Corsini et al. Citation2011, Citation2012, Citation2021; Racchi et al. Citation2017; Masi et al. Citation2022), (B) microglia (Yang et al. Citation2015; Wang et al. Citation2015; Zhu et al. Citation2015; Ge et al. Citation2016; Lin et al. Citation2022), and (C) macrophages (Chang et al. Citation2005; Miyano et al. Citation2012; Kong et al. Citation2019; Wang, Liu et al. Citation2021; Tian et al. Citation2021; Yu et al. Citation2022; Lee et al. Citation2022; Wang, Tan, et al. Citation2023). Black arrows and red lines with a ‘T’ end indicate normal function (activating and inhibiting, respectively). PFAS-induced endpoints for each gene/protein are shown graphically as red up arrows (increase in signal and/or expression), blue down arrows (decrease in signal/expression), dash (no observed change), checkmark (binding between protein and any PFAS observed in vitro), checkmark with question mark (binding between protein and any PFAS suggested in silico, but not demonstrated in vitro). Details are provided in Supplemental Table S1. AIM2: absent in melanoma 2; arg-1: Arginase 1; ASC: Apoptosis-associated speck-like protein containing a CARD; BAK: Bcl-2 homologous antagonist killer; BAX: Bcl-2-associated X protein; BCL-2: B-cell lymphoma 2; BIP: Binding immunoglobulin protein; Casp1: Caspase 1; Casp3: Caspase 3; CCL2: Chemokine ligand 2, also called MCP-1; CXCL1: Chemokine (CXC motif) ligand 1; CD: Cluster of differentiation (multiple types); COX2: Cytochrome c oxidase II; cyt C: Cytochrome C; ERK: Extracellular signal-regulated kinase; H2-Aa: Histocompatibility 2, class II antigen A, alpha; H2-K1: histocompatibility 2, K1, K region; IFNγ: interferon-γ; IκB: nuclear factor of κ-light polypeptide gene enhancer in B-cells inhibitor; IL: Interleukin (several types); iNOS: Inducible nitric oxide synthase; JNK: c-Jun N-terminal kinase; KO: knockout; LPS: Lipopolysaccharide; MAPK: Mitogen-activated protein kinase; MMP9: Matrix metallopeptidase-9; mtDNA: Mitochondrial DNA; NF-κB: Nuclear factor κ-light-chain-enhancer of activated B-cells; NLRP3: NOD-like receptor family pyrin domain containing 3; OPN: Osteopontin; PARP: Poly (ADP-ribose) polymerase; PGE-2: Prostaglandin E2; PI3K: Phosphoinositide 3-kinase; PKCβ: protein kinase Cβ; PPARα: peroxisome proliferator-activated receptor-α; RACK1: Receptor for activated C kinase 1; RCS: Reactive carbonyl species; ROS: Reactive oxygen species; STAT3: Signal transducer and activator of transcription-3; TNFα: tumor necrosis factor-α.
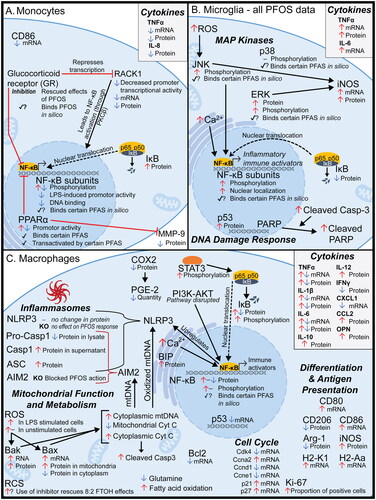
Figure 3. Visual summary of mechanisms suggested in tissue-level, acellular, and other studies not specifically in innate immune cells. PFAS have been shown to disrupt (A) Toll-like receptor signaling (Singh et al. Citation2012; Zhang et al. Citation2014; Zhang et al. Citation2021; Sheng et al. Citation2017; Chen et al. Citation2018; Han et al. Citation2018b; Castaño-Ortiz et al. Citation2019; Guo et al. Citation2019; Shane et al. Citation2020; Zhang et al. Citation2020; Zhong et al. Citation2020; Xie et al. Citation2021; Huang et al. Citation2022; Wan et al. Citation2022; Xu et al. Citation2022; Liu et al. Citation2023, Tang et al. Citation2023) and (B) PPAR signaling (Corsini et al. Citation2012; Pennings et al. Citation2016; Sheng et al. Citation2017; Wu et al. Citation2017; Rodríguez-Jorquera et al. Citation2019; Shane et al. Citation2020; Christofides et al. Citation2021; Khazaee et al. Citation2021; Weatherly et al. Citation2021; Zhang, Dong, et al. Citation2021; Camdzic et al. Citation2022, Chen et al. Citation2022; Evans et al. Citation2022; Wang et al. Citation2022; Xu et al. Citation2022; Sun et al. Citation2023), but the effect of PFAS on these pathways in innate immune cells remained understudied. Black arrows and red lines with a ‘T’ end indicate normal function (activating and inhibiting, respectively). PFAS-induced endpoints for each gene/protein are shown graphically as red up arrows (increase in signal/expression), blue down arrows (decrease in signal/expression), checkmark (binding between protein and any PFAS observed in vitro), checkmark with question mark (binding between protein and any PFAS suggested in silico, but not demonstrated in vitro). Details are provided in Supplemental Table S1. Abbreviations: CD36: Cluster of differentiation 36; E-FABP: Fatty acid binding protein [epidermal type]; HMGB1: High mobility group box 1 protein; IκB: Nuclear factor of κ-light polypeptide gene enhancer in B-cells inhibitor; IRAK: IL-1 receptor-associated kinase; MyD88: Myeloid differentiation primary response 88; NF-κB: Nuclear factor κ-light-chain-enhancer of activated B-cells; PPAR: Peroxisome proliferator-activated receptor; TLR: Toll-like receptor; TRAF6: TNF receptor associated factor-6.
![Figure 3. Visual summary of mechanisms suggested in tissue-level, acellular, and other studies not specifically in innate immune cells. PFAS have been shown to disrupt (A) Toll-like receptor signaling (Singh et al. Citation2012; Zhang et al. Citation2014; Zhang et al. Citation2021; Sheng et al. Citation2017; Chen et al. Citation2018; Han et al. Citation2018b; Castaño-Ortiz et al. Citation2019; Guo et al. Citation2019; Shane et al. Citation2020; Zhang et al. Citation2020; Zhong et al. Citation2020; Xie et al. Citation2021; Huang et al. Citation2022; Wan et al. Citation2022; Xu et al. Citation2022; Liu et al. Citation2023, Tang et al. Citation2023) and (B) PPAR signaling (Corsini et al. Citation2012; Pennings et al. Citation2016; Sheng et al. Citation2017; Wu et al. Citation2017; Rodríguez-Jorquera et al. Citation2019; Shane et al. Citation2020; Christofides et al. Citation2021; Khazaee et al. Citation2021; Weatherly et al. Citation2021; Zhang, Dong, et al. Citation2021; Camdzic et al. Citation2022, Chen et al. Citation2022; Evans et al. Citation2022; Wang et al. Citation2022; Xu et al. Citation2022; Sun et al. Citation2023), but the effect of PFAS on these pathways in innate immune cells remained understudied. Black arrows and red lines with a ‘T’ end indicate normal function (activating and inhibiting, respectively). PFAS-induced endpoints for each gene/protein are shown graphically as red up arrows (increase in signal/expression), blue down arrows (decrease in signal/expression), checkmark (binding between protein and any PFAS observed in vitro), checkmark with question mark (binding between protein and any PFAS suggested in silico, but not demonstrated in vitro). Details are provided in Supplemental Table S1. Abbreviations: CD36: Cluster of differentiation 36; E-FABP: Fatty acid binding protein [epidermal type]; HMGB1: High mobility group box 1 protein; IκB: Nuclear factor of κ-light polypeptide gene enhancer in B-cells inhibitor; IRAK: IL-1 receptor-associated kinase; MyD88: Myeloid differentiation primary response 88; NF-κB: Nuclear factor κ-light-chain-enhancer of activated B-cells; PPAR: Peroxisome proliferator-activated receptor; TLR: Toll-like receptor; TRAF6: TNF receptor associated factor-6.](/cms/asset/d6a385c2-afbb-488d-b9fe-0e9b070a9dcb/iimt_a_2343362_f0003_c.jpg)
Macrophages
Several mechanistic studies have been performed in macrophages, and they report disruptions in NF-κB signaling, MAPK signaling, cell cycle, and/or immunometabolism. Chang et al. (Citation2005) reported that FC-77 (perfluorooctane) suppressed the NF-κB pathway in a murine macrophage cell line (RAW 264.7). They observed decreases in NF-κB and Cox2 protein levels, along with an increase in IκB protein, which corresponded to decreases in the secretion of inflammatory cytokines IL-1β, IL-6, and TNFα, and an increase in anti-inflammatory IL-10. However, other studies have reported increased NF-κB activation with PFAS exposure. Wang, Tan, et al. (Citation2023) observed increases in p65 protein expression and phosphorylation with PFOS exposure in RAW 264.7 murine macrophages, along with increases in iNOS, TNFα, IL-1β, IL-6, and IL-10 protein levels. Interestingly, decreases in CD206 and Arg-1, which are markers of M2 macrophage polarization were also observed. These effects were all rescued by the use of NF-κB inhibitor, pointing to the key role that transcription factors may play in mediating PFAS-induced immunotoxic phenotypes.
In a study of mice exposed to GenX via inhalation, the alveolar macrophage cell cycle was disrupted, resulting in a proliferative phenotype, as determined by Ki67 staining and mitotic index (Lee et al. Citation2022). Cell cycle disruptions were also reported in RAW264.7 murine macrophages exposed to 8:2 FTOH, albeit this study observed cell cycle arrest (Kong et al. Citation2019). In both of these studies, changes in immunological endpoints were also observed, including increased expression of antigen-presenting genes (Kong et al. Citation2019), decreased cytokine expression at the transcript level (Kong et al. Citation2019), and increased phosphorylation of STAT3 (Lee et al. Citation2022). It remains unclear how cell cycle disruption may lead to immunotoxicity in these models, but this phenotype has also been observed in other cell types exposed to PFAS (Pierozan and Karlsson Citation2018; Pierozan et al. Citation2018).
A study exploring mechanisms of PFAS-induced immunotoxicity in innate immune cells from Wang, Lui, et al. (2021) investigated the effect of PFOS exposure on macrophages through parallel studies in human macrophage-like THP-1 cells and murine bone marrow-derived macrophages (BMDM). It was observed that increased levels of cytosolic calcium and the protein BiP, an indicator of ER stress, initiated PKC signaling. This, in turn, activated NF-κB, contributing to the activation of inflammatory pathways. The study also observed that PFOS-induced activation of the AIM2 inflammasome was mediated by mitochondrial dysfunction and the release of mitochondrial DNA (mtDNA), also contributing to the activation of inflammatory and pyroptotic pathways.
Studies of PFOA suggest that PFAS immunotoxicity in macrophages may be mediated, in part, through altered metabolism. Using non-targeted metabolomics, disruptions in glutamine, glutathione, and fatty acid metabolism were observed in the murine macrophage RAW264.7 cell line after PFOA exposure, along with increases in ROS production (Tian et al. Citation2021). An independent single-cell RNA-Seq study of PFOA-exposed larval zebrafish, observed 17 differentially-expressed genes (DEG) in macrophages; many of the genes were involved in metabolic pathways (e.g. of fatty acids, lipids, starches and sugars, pyruvate), as well as the PI3K-Akt pathway (Yu et al. Citation2022). Several studies have shown that the metabolic micro-environment of a macrophage, along with its intracellular metabolism, can modulate its activation state (Yan and Horng Citation2020). For example, increased abundance of certain fatty acids and increased β-oxidation can activate PPARγ signaling, shifting the macrophage toward an alternatively activated, anti-inflammatory state.
Kupffer cells
Five percent of all liver cells are resident macrophages known as Kupffer cells (Racanelli and Rehermann Citation2006). Studies suggest that Kupffer cells may be responsible for PFAS-induced liver inflammation and hepatocyte proliferation (Fang et al. Citation2012a, Citation2012b; Han et al. Citation2018b). A study on PFOS-exposed rats (Han et al. Citation2018b) indicated Kupffer cells were activated after exposure to PFOS, as determined by increased phosphorylation of NF-κB, IκB, and JNK, and increased secretion of TNFα and IL-6, which drive hepatocyte proliferation. This study also reported that Kupffer cells produce substantially higher levels of these cytokines than hepatocytes in response to PFOS exposure. Earlier studies by Fang et al. (Citation2012a, Citation2012b) found that PFNA also increases inflammatory cytokine production by Kupffer cells.
Microglia
Presently, all published mechanistic studies performed in microglia have been done under conditions of PFOS exposure. A study in a rat microglial cell line (HAPI cells) observed disruptions in JNK and ERK, but not p38, MAPK signaling upon exposure to PFOS (Wang et al. Citation2015). This study also observed increases in iNOS and elevated ROS levels. Another study in the same cell line also reported NF-κB activation and increased calcium levels (Yang et al. Citation2015). Co-treatment with PKC or calcium inhibitors rescued the increase in NF-κB, suggesting that increased calcium signaling is activating the NF-κB pathway in PFOS-exposed microglia. Increases in JNK and ERK phosphorylation, along with NF-κB, were also observed in the BV2 mouse microglial cell line (Zhu et al. Citation2015).
Monocytes
In contrast with other cell types, decreased NF-κB activity has been observed in monocytes exposed to PFAS, potentially through the influence of PPARα and the glucocorticoid receptor (GR). Corsini et al. (Citation2011) found that both PFOS and PFOA exposure in the THP-1 human monocytic cell line decreased NF-κB p65 phosphorylation and promoter activity. PFOS exposure also decreased p65 DNA binding and increased IκB protein levels. These changes in NF-κB signaling resulted in decreased secretion of TNFα and IL-8, and for PFOA, decreased MMP-9. They showed that silencing of PPARα via siRNA rescued effects of PFOA but not PFOS. Follow-up studies in the same cell line (Corsini et al. Citation2012) found that PFOA increased PPARα promoter activity, while 8:2 FTOH and PFOS decreased it. This study also found that PFBS, PFDA, PFOSA, and 8:2 FTOH reduced LPS-induced NF-κB promoter activity, and that PFOA, PFOS, PFBS, PFDA, PFOSA, and 8:2 FTOH reduced NF-κB subunit p65 protein levels.
PFOS has also been shown to suppress monocytic cytokine production through glucocorticoid receptor (GR) signaling (Masi et al. Citation2022). Also using THP-1 monocytes, the study reported reductions in TNFα and IL-8 production, similar to the previously described studies, along with decreased expression of CD86. Investigating whether Receptor for Activated C Kinase-1 (RACK1), an endocrine responsive mediator of immune function, could play a role, the study reported decreased RACK1 promoter transcriptional activity, along with decreased RACK1 mRNA and protein levels. Co-treatment with mifepristone (a GR inhibitor) rescued the effects. Follow-up molecular docking experiments indicated that PFOS could be a GR agonist, in part through formation of a salt bridge between the GR active site and the sulfonic head group of PFOS.
Promyelocytes
8:2 FTOH was found to cause G1 cell cycle arrest in promyelocytic leukemic HL-60 cells and decreases in cell cycle related genes CCNA1, CCNA2, CCND2, and CCNE2 (Wang et al. Citation2019). When these promyelocytes were differentiated into granulocytes with all-trans retinoic acid, levels of transcripts related to the differentiation of granulocytes, including CD11b, CSF3R, PU.1, and C/EBPε, decreased after exposure to 8:2 FTOH relative to unexposed controls. The same research group also found cell cycle disruptions in RAW264.7 macrophage-like cells (Kong et al. Citation2019). While this study reported no change in ROS levels, it was observed that use of the reactive carbonyl species (RCS) inhibitor, hydralazine hydrochloride, rescued the cytotoxicity caused by 8:2 FTOH.
Mast cells
Several mast cell signaling pathways have been seen to be disrupted by PFAS exposure. Increases in NF-κB activity were measured in a human mast cell line (HMC-1) exposed to PFOA, along with increased phosphorylation of MAPK subunit p38, caspase-1, and Cox-2 (Singh et al. Citation2012). Another study using the HMC-1 cell line along with murine bone marrow-derived mast cells (BMMC) suggested that PFOS disrupted the Fyn-Lyn-Syk pathway (Park et al. Citation2021). In addition to increased phosphorylation of Fyn, Lyn, and Syk, knockdown of these three genes rescued PFOS-induced increases in β-hexosaminidase release, intracellular calcium levels, and phosphorylation of ERK, Akt, and PLCγ1. The study also observed that PFOA and, to a lesser extent, PFHxS could induce activation in BMMC.
Three studies used the rat basophilic cell line RBL-2H3 to model the effects of PFAS on mast cells. As these cells exhibit attributes of both basophils and mast cells (Passante and Frankish Citation2009), mechanistic studies using the RBL-2H3 cell line may lend insights into how both basophil and mast cell function may be impacted by PFAS exposure. As seen in HMC-1 cells, PFOA elevated nuclear NF-κB levels in RBL-2H3 cells (Lee et al. Citation2017). The study also found decreased levels of IκBα and increased TNFɑ secretion at non-cytotoxic levels. At cytotoxic levels, increased secretion of IL-1β, IL-6, IL-8, histamine, and β-hexosaminidase were also seen, along with elevated levels of intracellular calcium. Similar findings were found in a second RBL-2H3 study that explored the role of PFAS chain length in mast cell degranulation (Lee and Kim Citation2018). At non-cytotoxic levels, PFDA and PFUnDA, but not PFHpA or PFNA, were found to increase β-hexosaminidase, histamine, and TNFα release along with increased intracellular calcium. This may be mediated through the actions of NF-κB, as the study also found increased activation using a luciferase reporter and increased transcript levels of tnfa, il6, il8, and il1b. PFAS-mediated changes in calcium signaling was suggested by another study reporting that PFOS- and PFOA-induced β-hexosaminidase could be ameliorated by depleting extracellular calcium levels or through pre-activation of Protein Kinase C (Yamaki and Yoshino Citation2010). This study also suggested that histamine and β-hexosaminidase release at cytotoxic levels occurred through the surfactant action of PFOS and PFOA on cell membranes. Together, these studies suggest that the effects of PFAS on basophils and mast cells may be partially mediated through altered calcium signaling and NF-κB signaling.
Pertinent findings from non-immune cell-based studies
Studies done using tissues (e.g. liver) and examining PFAS interactions with specific receptors can yield insights into how innate immune signaling may be affected. For example, some studies suggest that Toll-like receptors (TLRs) and the signaling component MyD88 are disrupted by PFAS exposure (). These are known activators of NF-κB in immune cells. TLRs recognize and engage pathogen associated molecular patterns (PAMPs) such as viral RNA or components of bacterial cell walls. Several of these studies showed that exposure to PFOA, GenX, and/or 6:2 FTSA causes increases in TLR2 or TLR4 protein and mRNA levels in zebrafish spleen (Zhang et al. Citation2014; Zhong et al. Citation2020) and kidney (Zhang, Shen, et al. Citation2021) as well as in mouse liver (Sheng et al. Citation2017; Xu, Chen, Zhang, et al. Citation2022) and GI tract (Xie et al. Citation2021). Some of these studies also observed up- or down-regulation of MyD88 mRNA (Zhang et al. Citation2014; Zhang, Shen, et al. Citation2021; Zhong et al. Citation2020; Xu, Chen, Zhang, et al. Citation2022). Altered baseline levels of these receptors, along with disruption in downstream NF-κB signaling, could affect the immune response and levels of inflammation. Additionally, pathway analyses of RNA-Seq data from frog and fish livers, rat blood, and whole larval zebrafish exposed to assorted PFAS have suggested other immune pathways, including NOD-like receptor signaling and a plethora of viral response pathways, are also impacted by PFAS exposure (Zhang et al. Citation2020; Lin et al. Citation2022; Wang, Zhao, et al. Citation2023; Zhang, Ren, et al. Citation2023).
Other studies have reported that PFAS bind to and/or activate fatty acid metabolism-related proteins expressed in innate immune cells (). A major family in this category is the peroxisome proliferator-activated receptors (PPAR). These nuclear receptors are well-known for their role in mediating inflammation and are expressed in several immune cell types, including macrophages, dendritic cells, mast cells, and adaptive immune cells (Christofides et al. Citation2021). Several studies have demonstrated the ability of various PFAS to bind different PPAR and modulate their activity (Corsini et al. Citation2011, Khazaee et al. Citation2021, Evans et al. Citation2022). PFAS have also been shown to modulate PPAR mRNA levels in mouse skin and liver, human blood, and fathead minnow blood (Pennings et al. Citation2016, Sheng et al. Citation2017, Rodríguez-Jorquera et al. Citation2019, Shane et al. Citation2020). Other fatty acid receptors are likely also involved. While fatty acid transporter and scavenger receptor CD36 is understudied with respect to PFASs and the immune system, there is evidence that it can transport multiple PFAS into cells (Camdzic et al. Citation2022), potentially leading to altered lipid metabolism and activation of PPARγ in macrophages, monocytes, dendritic cells, and microglia (Chen et al. Citation2022). Several PFASs have also been shown to bind human fatty acid binding protein 5 (FABP5, also known E-FABP) (Zhang, Shen, et al. Citation2021), which influences immune signaling in antigen-presenting cells (Xu, Chen, Zhan, et al. Citation2022). More work is needed to understand the influence of PFAS on innate immune cell metabolism and potential downstream effects on immune cell activation and function.
Molecular docking and other in silico experiments suggest that PFAS can bind to several other receptors found in innate immune cells. Besides the PFOS and glucocorticoid receptor study mentioned previously (Masi et al. Citation2022), in silico studies have suggested that PFOS and/or PFOA can bind to select TLR, MAP kinases, and NF-κB in multiple species. An in silico study investigating possible binding of PFBA, PFOA, and PFNA to human TLR1, TLR2, TLR4, and TLR5 predicted that TLR1 and TLR2 can bind all three PFAS (Tang et al. Citation2023). To a lesser extent, TLR4 was also suggested to bind to PFOA and PFNA, but not PFBA. PFAS also have been suggested to bind downstream signaling mediators. For example, an in silico study suggested that PFOS, PFOA, and 6:2 Cl-PFESA can bind to MAP kinases ERK, JNK, and p38 from Rana nigromaculata (black-spotted frog) (Lin et al. Citation2022). A second docking study using proteins from the same species indicated that PFOA and PFOS could bind both NF-κB1 and NF-κB2 (Liu et al. Citation2023). This is supported by an in silico study that predicted that PFOS, as well as two of its alternatives, OBS and F-53B, can bind zebrafish NF-κB directly (Huang et al. Citation2022). Assuming these findings are confirmed using in vitro and in vivo experiments, it remains unclear how this binding may affect the activity of these proteins.
A recent report investigated if PFASs interfere with myeloperoxidase (MPO), an enzyme involved in neutrophil respiratory burst (Phelps et al. Citation2023). No significant effect was observed on the chlorination activity of MPO (through which halogenated ROS are produced) after treatment with GenX, PFHxA, or PFOA. However, it was observed that PFOA and PFHxA exposure increased MPO peroxidation activity, through which it returns to a ‘ground state.’ At this time, it is unclear how these results contribute to the observed immunosuppression, if at all.
Mechanistic themes and data gaps
One major theme of these studies is that the NF-κB signaling pathway is disrupted by many PFAS, in many cell types, and in diverse species. While not discussed thoroughly above, disruption of NF-κB and several of its upstream regulators, as measured by mRNA, protein levels, and/or phosphorylation, has been observed in diverse tissue types, including dermal tissue, liver, spleen, and colon (select references include: Zhang et al. Citation2014; Sheng et al. Citation2017; Guo et al. Citation2019; Shane et al. Citation2020; Xie et al. Citation2021; Xu, Chen, Zhang, et al. Citation2022). NF-κB is perhaps the best-studied gene/protein in PFAS-exposed innate immune cells, and is consistently disrupted by PFAS exposure. It is possible that binding of NF-κB could be the molecular initiating event (MIE) of PFAS-induced immunotoxicity in the innate immune system, particularly since two independent computational studies have predicted that various PFAS can bind NF-κB (Huang et al. Citation2022; Liu et al. Citation2023). However, the MIE may be upstream of NF-κB, given that genes such as MyD88 and several TLRs have been disrupted by PFAS exposure, as previously discussed. Further, certain TLR and MAPK, both of which modulate NF-κB activation, also have been suggested to bind PFAS. Of course, there are other candidates for the MIE, including various PPAR. These nuclear receptors, isoforms of which are present in most immune cells, are known to be activated by select PFAS (Evans et al. Citation2022; Søderstrøm et al. Citation2022) and interact with NF-κB (Toobian et al. Citation2021). For example, PPARγ is known to inhibit NF-κB activity through competitive binding on promoters (Bonfield et al. Citation2008). Establishing different MIE for immu-notoxicity induced by PFAS will allow for the creation of adverse outcome pathways (AOP) which can provide insight into tissue, organ, and organismal health.
A second emerging theme is that basic cell functions are being disrupted. Several studies have found exposure to various PFAS increased levels of ROS and other reactive species, altered cell cycle progression, and disrupted metabolism. These effects may influence immune cell counts and interfere with the ability of immune cells to perform basic functions when challenged. Note that while certain immune cells, such as neutrophils, produce ROS intentionally to ward off infection, many of these studies reported increased ROS in unstimulated immune cells. Given that cell stress, metabolism, and cell cycle pathways are conserved across many cell types, not just immune cells, these findings could point to a universal target of PFAS toxicity.
Indeed, evidence of metabolic disruption by PFAS exposure has been found in adaptive immune cells, cord blood, serum, and hepatocytes (Rowan-Carroll et al. Citation2021; Ehrlich et al. Citation2023, India-Aldana et al. Citation2023; Taylor et al. Citation2023). This suggests that when it comes to innate immune cells, macrophages will not be the only ones with disrupted metabolism. In various innate immune cells, altered metabolism can influence activation state and function, and vice versa (Jiang et al. Citation2022; Surace and di Santo Citation2022). While the effect of PFAS on cell cycle, metabolism, and oxidative stress are being studied in diverse cell types, more work is needed to understand how various PFAS affect basic cell functions in innate immune cells and the downstream effects on immune function.
Another theme is that the directionality of PFAS-induced effects is not consistent. The levels or activity of a given protein may increase or decrease depending on the specific PFAS, the dose, the dose duration, the cell type, and the species. For example, a study of MyD88 mRNA in zebrafish spleen found PFOA exposure increased levels over shorter exposures and decreased levels over longer exposures (Zhang, Shen, et al. Citation2021). One potential explanation for this is endocrine disruption, potentially through various PPAR. In a study using a human monocyte cell line, PFOA was found to increase promoter activity of PPARα, while PFOSA and 8:2 FTOH decreased it (Corsini et al. Citation2012). Other studies have suggested that thyroid, estrogen, and glucocorticoid signaling may be involved (Rodríguez-Jorquera et al. Citation2019; Evans et al. Citation2022; Masi et al. Citation2022; Coperchini et al. Citation2023). The interaction of some PFAS with differing PPAR and other endocrine receptors could explain why some studies find both activation and suppression of immune cell functions in a dose-dependent manner. These variations in response present a challenge to predicting outcomes of both mixtures and chronic exposures.
The data gaps regarding the effects of PFAS on the innate immune system are significant. In this review, evidence was identified for 25 PFAS where effects were observed in the innate immune system, representing < 1% of all known PFAS. While limited, this dataset suggests that PFAS can act through multiple molecular targets to cause toxicity. While some less well-studied PFAS may act through mechanisms similar to PFOS and PFOA, it remains unclear whether this will indeed be the case for all PFASs. While PFASs share a set of structural motifs (e.g., perfluorinated carbons), they vary substantially in other structural attributes (e.g. chain length, types of functional groups) (Wang, DeWitt, et al. 2017; Wang, Buser et al. Citation2021). This structural diversity may cause some PFAS subsets to bind better to certain receptors, while other PFAS subsets bind better to other types of receptors, leading to multiple distinct modes of action. For example, while many of the perfluorosulfonic and per-fluorocarboxylic acids can transactivate PPARα and PPARγ but not the estrogen receptor (ER), the fluorotelomer alcohols are much more likely to transactivate ER than either PPAR (Evans et al. Citation2022). This will likely lead to different downstream effects on immune function. More side-by-side studies of structurally diverse PFAS should be done to address the question of how many distinct molecular pathways can be impacted by this large chemical class, and which PFAS are operating through shared modes of action. Additionally, more mixtures studies are needed to better understand the impact of real-world exposures on innate immune function.
Further, not enough is yet known to determine whether these molecular mechanisms are shared across cell types. While different mechanisms seem to be emerging, this may be the result of multiple independent studies being done in different labs addressing the impact of different PFAS on different cell types. Even though macrophages, monocytes, and microglia currently dominate the mechanistic studies, there is not enough data to determine whether each of those closely related cell types are all affected in the same way by the same PFAS. For example, the effect of PFAS on MAPK signaling in innate immune cells has primarily been studied in microglia and, to a lesser extent, mast cells. Presently, mechanistic data for the effect of PFAS on several key innate immune cell types, including neutrophils, eosinophils, and natural killer cells, are not available. While many immune signaling pathways are shared across immune cells, different cell types may respond differently to the same stimuli due to differences in receptor expression and other nuanced differences among immune cells. NF-κB, for example, can be activated through at least four distinct signaling pathways in immune cells (Vallabhapurapu and Karin Citation2009), each of which may be differentially affected by PFAS exposure.
It is also not yet clear to what extent these mechanisms will be shared across species. The bulk of mechanistic work has been done in rodent or human models, with a few studies in zebrafish and other species. While the innate immune system is remarkably conserved across species, there are still differences that may affect PFAS immunotoxicity. For example, due to differences in expression, rodents are much more sensitive than humans when it comes to PFAS that act through PPARα (DeWitt et al. Citation2009). When it comes to assessing the effects of PFAS exposure on wildlife, there is a substantial data gap. More work is needed on diverse models, particularly those relevant for wildlife health (fish, birds, reptiles, etc.).
Additionally, while NF-κB is clearly disrupted by PFAS exposure, other important immune signaling pathways are understudied or not studied at all. Components of JAK-STAT and MAPK signaling pathways, where studied in innate immune cells, seem to also be disrupted by PFAS exposure, yet there are only a handful of studies investigating these pathways. This may be due to a biased approach, as many of these studies measured the expression of a limited number of genes and/or proteins. Increased usage of unbiased approaches such as RNA-Seq will help address this discrepancy while working toward a mode (or modes) of action for PFAS immunotoxicity.
Another major data gap is that immune receptors are underrepresented and understudied in binding and activation assays. The bulk of this work so far has been carried out with hepatotoxicity in mind. While there is some overlap in proteins expressed by liver cells and those expressed by immune cells (e.g., PPARα, NF-κB, and CD36), PFAS-protein binding experiments are often lacking for variants found in immune cells. As an example, macrophages express adipose- and epidermal-fatty acid binding proteins (FABP4 and 5, respectively) (Jin et al. Citation2021), but the bulk of work done with PFAS binding in this family is with liver-FABP (FABP1).
Conclusions
Much remains to be learned about the impacts of PFAS exposure on innate immunity. It can be concluded that as a class, PFAS can suppress and activate innate immune functions, as has been reported for components of the adaptive immune system. However, the observed effects must be dissected further to determine the doses/concentrations, exposure windows, individual PFAS, and PFAS mixtures that may contribute to the immunotoxicity of these compounds. While some studies have sought to elucidate universal mechanisms of PFAS toxicity, few have been successful; given that PFAS target multiple organ systems via several different receptors, multiple mechanisms are likely at play. Of course, this makes defining MIE difficult when working to create AOP. Given the expanding number of PFAS and their structural diversity, there is a need for new high-throughput methods that can assess innate immune function. Moving forward, the field should work to establish functional assays that can be used to infer the hazard potential of PFAS in the innate immune system in humans, experimental animal models, and wildlife. In addition to establishing functional assays, the field should also increase the monitoring of human populations with high serum levels of PFAS through epidemiological studies to better understand the implications of findings in the in vitro and in vivo immunotoxicity studies. Innate immunity is critical for organismal and population health, and given the scale of PFAS contamination across the globe, it is imperative to work efficiently to answer the essential questions of PFAS-induced toxicities to protect human and environmental health.
Supplemental Material
Download MS Excel (51.8 KB)Acknowledgements
The Authors apologize to those colleagues whose work could not be cited because of space limitations. Note: portions of this manuscript were included as part of DWP doctoral dissertation (Phelps Citation2022).
Disclosure statement
DWP was paid consulting fees by the Center for Environmental Health (Oakland, CA), for consulting regarding a petition for testing of certain PFAS (May 2019–August 2022) as well as to provide toxicological expertise regarding the PFAS detected in fluorinated high-density polyethylene (March–May 2023). DWP was also paid to provide literature support to JCD for a PFAS expert witness report (May 2023). JCD serves/has served as a plaintiff’s expert witness for several cases involving PFAS manufacturers. All other authors declare no competing interests relevant to the content of this article.
Additional information
Funding
References
- Abraham K, Mielke H, Fromme H, Völkel W, Menzel J, Peiser M, Zepp F, Willich S, Weikert C. 2020. Internal exposure to perfluoroalkyl substances (PFAS) and biological markers in 101 healthy 1-year-old children: associations between levels of perfluorooctanoic acid (PFOA) and vaccine response. Arch Toxicol. 94(6):2131–2147. doi: 10.1007/s00204-020-02715-4.
- Agency for Toxic Substances and Disease Registry [ATSDR]. 2021. Toxicological profile for perfluoroalkyls. Atlanta: U.S. Department of Health and Human Services.
- Aggad D, Mazel M, Boudinot P, Mogensen K, Hamming O, Hartmann R, Kotenko S, Herbomel P, Lutfalla G, Levraud JP. 2009. The two groups of zebrafish virus-induced interferons signal via distinct receptors with specific and shared chains. J Immunol. 183(6):3924–3931. doi: 10.4049/jimmunol.0901495.
- Altmann S, Mellon M, Distel D, Kim C. 2003. Molecular and functional analysis of an interferon gene from the zebrafish, Danio rerio. J Virol. 77(3):1992–2002. doi: 10.1128/jvi.77.3.1992-2002.2003.
- Andrews D, Naidenko O. 2020. Population-wide exposure to per- and polyfluoroalkyl substances from drinking water in the United States. Environ Sci Technol Lett. 7(12):931–936. doi: 10.1021/acs.estlett.0c00713.
- Bangma J, Guillette T, Bommarito P, Ng C, Reiner J, Lindstrom A, Strynar M. 2022. Understanding the dynamics of physiological changes, protein expression, and PFAS in wildlife. Environ Int. 159:107037. doi: 10.1016/j.envint.2021.107037.
- Bassler J, Ducatman A, Elliott M, Wen S, Wahlang B, Barnett J, Cave MC. 2019. Environmental perfluoroalkyl acid exposures are associated with liver disease characterized by apoptosis and altered serum adipocytokines. Environ Pollut. 247:1055–1063. doi: 10.1016/j.envpol.2019.01.064.
- Berntsen H, Bodin J, Øvrevik J, Berntsen CF, Østby G, Brinchmann B, Ropstad E, Myhre O. 2022. A human relevant mixture of persistent organic pollutants induces reactive oxygen species formation in isolated human leucocytes: involvement of the β2-adrenergic receptor. Environ Int. 158:106900. doi: 10.1016/j.envint.2021.106900.
- Berntsen HF, Bølling AK, Bjørklund CG, Zimmer K, Ropstad E, Zienolddiny S, Becher R, Holme JA, Dirven H, Nygaard UC, et al. 2018. Decreased macrophage phagocytic function due to xenobiotic exposures in vitro, difference in sensitivity between various macrophage models. Food Chem Toxicol. 112:86–96. doi: 10.1016/j.fct.2017.12.024.
- Blake B, Fenton S. 2020. Early life exposure to per- and polyfluoroalkyl substances (PFAS) and latent health outcomes: a review including the placenta as a target tissue and possible driver of peri- and post-natal effects. Toxicology. 443:152565. doi: 10.1016/j.tox.2020.152565.
- Bodin J, Groeng E, Andreassen M, Dirven H, Nygaard U. 2016. Exposure to perfluoroundecanoic acid (PFUnDA) accelerates insulitis development in a mouse model of Type 1 diabetes. Toxicol Rep. 3:664–672. doi: 10.1016/j.toxrep.2016.08.009.
- Bonfield T, Thomassen M, Farver C, Abraham S, Koloze M, Zhang X, Mosser D, Culver D. 2008. Peroxisome proliferator-activated receptor (PPAR)-γ regulates expression of alveolar macrophage macrophage colony-stimulating factor. J Immunol. 181(1):235–242. doi: 10.4049/jimmunol.181.1.235.
- Boone J, Vigo C, Boone T, Byrne C, Ferrario J, Benson R, Donohue J, Simmons J, Kolpin D, Furlong E, et al. 2019. Per- and polyfluoroalkyl substances in source and treated drinking waters of the United States. Sci Total Environ. 653:359–369. doi: 10.1016/j.scitotenv.2018.10.245.
- Boronow K, Brody J, Schaider L, Peaslee G, Havas L, Cohn B. 2019. Serum concentrations of PFASs and exposure-related behaviors in African American and non-Hispanic white women. J Expo Sci Environ Epidemiol. 29(2):206–217. doi: 10.1038/s41370-018-0109-y.
- Brieger A, Bienefeld N, Hasan R, Goerlich R, Haase H. 2011. Impact of perfluorooctanesulfonate and perfluorooctanoic acid on human peripheral leukocytes. Toxicol In Vitro. 25(4):960–968. doi: 10.1016/j.tiv.2011.03.005.
- Buck R, Franklin J, Berger U, Conder J, Cousins I, de Voogt P, Jensen A, Kannan K, Mabury S, van Leeuwen S. 2011. Perfluoroalkyl and polyfluoroalkyl substances in the environment: terminology, classification, and origins. Integr Environ Assess Manag. 7(4):513–541. doi: 10.1002/ieam.258.
- Camdzic M, Aga D, Atilla-Gokcumen G. 2022. cellular interactions and fatty acid transporter CD36-mediated uptake of per- and polyfluorinated alkyl substances (PFAS). Chem Res Toxicol. 35(4):694–702. doi: 10.1021/acs.chemrestox.2c00078.
- Castaño-Ortiz J, Jaspers V, Waugh C. 2019. PFOS mediates immunomodulation in an avian cell line that can be mitigated via a virus infection. BMC Vet Res. 15(1):214. doi: 10.1186/s12917-019-1953-2.
- Chang E, Adami H, Boffetta P, Wedner H, Mandel J. 2016. A critical review of perfluorooctanoate and perfluorooctanesulfonate exposure and immunological health conditions in humans. Crit Rev Toxicol. 46(4):279–331. doi: 10.3109/10408444.2015.1122573.
- Chang H, Kuo F, Lai Y, Chou T. 2005. Inhibition of inflammatory responses by FC-77, a perfluorochemical, in lipopolysaccharide-treated RAW 264.7 macrophages. Intensive Care Med. 31(7):977–984. doi: 10.1007/s00134-005-2652-y.
- Chang X, Tan Y, Allen D, Bell S, Brown P, Browning L, Ceger P, Gearhart J, Hakkinen P, Kabadi S, et al. 2022. IVIVE: facilitating the use of in vitro toxicity data in risk assessment and decision-making. Toxics. 10(5):232. doi: 10.3390/toxics10050232.
- Chen J, Tang L, Chen W-Q, Peaslee GF, Jiang D. 2020. Flows, stock, and emissions of poly- and perfluoroalkyl substances in California carpet in 2000-2030 under different scenarios. Environ Sci Technol. 54(11):6908–6918. doi: 10.1021/acs.est.9b06956.
- Chen X, Nie X, Mao J, Zhang Y, Yin K, Sun P, Luo J, Liu Y, Jiang S, Sun L. 2018. Perfluorooctane sulfonate mediates secretion of IL-1β through PI3K/AKT NF-κB pathway in astrocytes. Neurotoxicol Teratol. 67:65–75. doi: 10.1016/j.ntt.2018.03.004.
- Chen Y, Zhang J, Cui W, Silverstein RL. 2022. CD36, a signaling receptor and fatty acid transporter that regulates immune cell metabolism and fate. J Exp Med. 219:e20211314.
- Christofides A, Konstantinidou E, Jani C, Boussiotis V. 2021. The role of peroxisome proliferator-activated receptors (PPAR) in immune responses. Metabolism. 114:154338. doi: 10.1016/j.metabol.2020.154338.
- Coperchini F, de Marco G, Croce L, Denegri M, Greco A, Magri F, Tonacchera M, Imbriani M, Rotondi M, Chiovato L. 2023. PFOA, PFHxA and C6O4 differently modulate the expression of CXCL8 in normal thyroid cells and in thyroid cancer cell lines. Environ Sci Pollut Res Int. 30(23):63522–63534. doi: 10.1007/s11356-023-26797-6.
- Cordner A, De La Rosa V, Schaider L, Rudel R, Richter L, Brown P. 2019. Guideline levels for PFOA and PFOS in drinking water: role of scientific uncertainty, risk assessment decisions, and social factors. J Expo Sci Environ Epidemiol. 29(2):157–171. doi: 10.1038/s41370-018-0099-9.
- Corsini E, Avogadro A, Galbiati V, dell’Agli M, Marinovich M, Galli CL, Germolec DR. 2011. In vitro evaluation of immunotoxic potential of perfluorinated compounds (PFCs). Toxicol Appl Pharmacol. 250(2):108–116. doi: 10.1016/j.taap.2010.11.004.
- Corsini E, Buoso E, Galbiati V, Racchi M. 2021. Role of protein kinase C in immune cell activation and its implication chemical-induced immunotoxicity. Adv Exp Med Biol. 1275:151–163. doi: 10.1007/978-3-030-49844-3_6.
- Corsini E, Sangiovanni E, Avogadro A, Galbiati V, Viviani B, Marinovich M, Galli CL, Dell’Agli M, Germolec DR. 2012. In vitro characterization of the immunotoxic potential of several perfluorinated compounds (PFC). Toxicol Appl Pharmacol. 258(2):248–255. doi: 10.1016/j.taap.2011.11.004.
- Cousins IT, DeWitt JC, Glüge J, Goldenman G, Herzke D, Lohmann R, Miller M, Ng CA, Scheringer M, Vierke L, et al. 2020. Strategies for grouping per- and polyfluoroalkyl substances (PFAS) to protect human and environmental health. Environ Sci Process Impacts. 22(7):1444–1460. doi: 10.1039/d0em00147c.
- DeWitt J, Blossom S, Schaider L. 2019. Exposure to per-fluoroalkyl and polyfluoroalkyl substances leads to immunotoxicity:epidemiological and toxicological evidence. J Expo Sci Environ Epidemiol. 29(2):148–156. doi: 10.1038/s41370-018-0097-y.
- DeWitt J, editor. 2015. Toxicological effects of perfluoroalkyl and polyfluoroalkyl substances. London: Humana Press.
- DeWitt JC, Shnyra A, Badr MZ, Loveless SE, Hoban D, Frame SR, Cunard R, Anderson SE, Meade BJ, Peden-Adams MM, et al. 2009. Immunotoxicity of perfluorooctanoic acid and perfluorooctane sulfonate and role of PPAR-α. Crit Rev Toxicol. 39(1):76–94. doi: 10.1080/10408440802209804.
- Domingo J, Nadal M. 2019. Human exposure to per- and polyfluoroalkyl substances (PFAS) through drinking water: a review of the recent scientific literature. Environ Res. 177:108648. doi: 10.1016/j.envres.2019.108648.
- Dong G, Liu M, Wang D, Zheng L, Liang Z, Jin Y. 2011. Sub-chronic effect of perfluorooctane-sulfonate (PFOS) on the balance of Type 1 and Type 2 cytokine in adult C57Bl/6 mice. Arch Toxicol. 85(10):1235–1244. doi: 10.1007/s00204-011-0661-x.
- Dong G, Zhang Y, Zheng L, Liu W, Jin Y, He Q. 2009. Chronic effects of perfluorooctane-sulfonate exposure on immunotoxicity in adult male C57BL/6 mice. Arch Toxicol. 83(9):805–815. doi: 10.1007/s00204-009-0424-0.
- Ehrlich V, Bil W, Vandebriel R, Granum B, Luijten M, Lindeman B, Grandjean P, Kaiser A, Hauzenberger I, Hartmann C, et al. 2023. Consideration of pathways for immunotoxicity of per- and polyfluoroalkyl substances (PFAS). Environ Health. 22(1):19. doi: 10.1186/s12940-022-00958-5.
- Emmett E, Zhang H, Shofer F, Freeman D, Rodway N, Desai C, Shaw L. 2006. Community exposure to perfluorooctanoate: relationships between serum levels and certain health parameters. J Occup Environ Med. 48(8):771–779. doi: 10.1097/01.jom.0000233380.13087.37.
- Evans N, Conley J, Cardon M, Hartig P, Medlock-Kakaley E, Gray L. 2022. In vitro activity of a panel of per- and polyfluoroalkyl substances (PFAS), fatty acids, and pharmaceuticals in peroxisome proliferator-activated receptor (PPAR)-α, PPAR-γ, and estrogen receptor assays. Toxicol Appl Pharmacol. 449:116136. doi: 10.1016/j.taap.2022.116136.
- Fair P, Romano T, Schaefer A, Reif J, Bossart G, Houde M, Muir D, Adams J, Rice C, Hulsey T, et al. 2013. Associations between perfluoroalkyl compounds and immune and clinical chemistry parameters in highly-exposed bottlenose dolphins (Tursiops truncatus). Environ Toxicol Chem. 32(4):736–746. doi: 10.1002/etc.2122.
- Fang X, Gao G, Xue H, Zhang X, Wang H. 2012a. In vitro and in vivo studies of the toxic effects of perfluorononanoic acid on rat hepatocytes and Kupffer cells. Environ Toxicol Pharmacol. 34(2):484–494. doi: 10.1016/j.etap.2012.06.011.
- Fang X, Zhang L, Feng Y, Zhao Y, Dai J. 2008. Immunotoxic effects of perfluorononanoic acid on BALB/c mice. Toxicol Sci. 105(2):312–321. doi: 10.1093/toxsci/kfn127.
- Fang X, Zou S, Zhao Y, Cui R, Zhang W, Hu J, Dai J. 2012b. Kupffer cells suppress perfluoro-nonanoic acid-induced hepatic PPAR-α expression by releasing cytokines. Arch Toxicol. 86(10):1515–1525. doi: 10.1007/s00204-012-0877-4.
- Fenton S, Ducatman A, Boobis A, DeWitt J, Lau C, Ng C, Smith J, Roberts S. 2021. Per- and polyfluoroalkyl substance toxicity and human health review: current state of knowledge and strategies for informing future research. Environ Toxicol Chem. 40(3):606–630. doi: 10.1002/etc.4890.
- Fernandez R, Sarma V, Younkin E, Hirschl R, Ward P, Younger J. 2001. Exposure to perflubron is associated with decreased Syk phosphorylation in human neutrophils. J Appl Physiol (1985). 91(5):1941–1947. doi: 10.1152/jappl.2001.91.5.1941.
- Frawley R, Smith M, Cesta M, Hayes-Bouknight S, Blystone C, Kissling G, Harris S, Germolec D. 2018. Immunotoxic and hepatotoxic effects of perfluoro-n-decanoic acid (PFDA) on female Harlan Sprague-Dawley rats and B6C3F1/N mice when administered by oral gavage for 28 days. J Immunotoxicol. 15(1):41–52. doi: 10.1080/1547691X.2018.1445145.
- Ge J, Wang C, Nie X, Yang J, Lu H, Song X, Su K, Li T, Han J, Zhang Y, et al. 2016. ROS-mediated apoptosis of HAPI microglia through p53 signaling following PFOS exposure. Environ Toxicol Pharmacol. 46:9–16. doi: 10.1016/j.etap.2016.06.025.
- Glüge J, Scheringer M, Cousins I, DeWitt J, Goldenman G, Herzke D, Lohmann R, Ng C, Trier X, Wang Z. 2020. Overview of uses of per- and polyfluoroalkyl substances (PFAS). Environ Sci Process Impacts. 22(12):2345–2373. doi: 10.1039/d0em00291g.
- Goodrich J, Calkins M, Caban-Martinez A, Stueckle T, Grant C, Calafat A, Nematollahi A, Jung A, Graber J, Jenkins T, et al. 2021. Per- and polyfluoroalkyl substances, epigenetic age and DNA methylation: a cross-sectional study of firefighters. Epigenomics. 13(20):1619–1636. doi: 10.2217/epi-2021-0225.
- Guillette T, Jackson T, Guillette M, McCord J, Belcher S. 2022. Blood concentrations of per- and polyfluoroalkyl substances are associated with autoimmune-like effects in American alligators from Wilmington, North Carolina. Front Toxicol. 4:1010185. doi: 10.3389/ftox.2022.1010185.
- Guillette T, McCord J, Guillette M, Polera M, Rachels K, Morgeson C, Kotlarz N, Knappe D, Reading B, Strynar M, et al. 2020. Elevated levels of per- and polyfluoroalkyl substances in Cape Fear River striped bass (Morone saxatilis) are associated with biomarkers of altered immune and liver function. Environ Int. 136:105358. doi: 10.1016/j.envint.2019.105358.
- Guo H, Zhang H, Sheng N, Wang J, Chen J, Dai J. 2021. Perfluorooctanoic acid (PFOA) exposure induces splenic atrophy via over-activation of macrophages in male mice. J Hazard Mater. 407:124862. doi: 10.1016/j.jhazmat.2020.124862.
- Guo J, Wu P, Cao J, Luo Y, Chen J, Wang G, Guo W, Wang T, He X. 2019. PFOS disturbed immunomodulatory functions via NF-κB signaling in liver of zebrafish (Danio rerio). Fish Shellfish Immunol. 91:87–98. doi: 10.1016/j.fsi.2019.05.018.
- Han J, Gu W, Barrett H, Yang D, Tang S, Sun J, Liu J, Krause H, Houck K, Peng H. 2021. A roadmap to the structure-related metabolism pathways of per- and polyfluoroalkyl substances in the early life stages of zebrafish (Danio rerio). Environ Health Perspect. 129(7):77004. doi: 10.1289/EHP7169.
- Han R, Hu M, Zhong Q, Wan C, Liu L, Li F, Zhang F, Ding W. 2018a. Perfluorooctane sulphonate induces oxidative hepatic damage via mitochondria-dependent and NF-κB/TNFα- mediated pathway. Chemosphere. 191:1056–1064. doi: 10.1016/j.chemosphere.2017.08.070.
- Han R, Zhang F, Wan C, Liu L, Zhong Q, Ding W. 2018b. Effect of perfluorooctane sulphonate-induced Kupffer cell activation on hepatocyte proliferation through the NF-κB/TNFα-/IL-6-dependent pathway. Chemosphere. 200:283–294. doi: 10.1016/j.chemosphere.2018.02.137.
- Hansen E, Huber N, Bustnes J, Herzke D, Bårdsen B, Eulaers I, Johnsen T, Bourgeon S. 2020. A novel use of the leukocyte coping capacity assay to assess the immunomodulatory effects of organohalogenated contaminants in avian wildlife. Environ Int. 142:105861. doi: 10.1016/j.envint.2020.105861.
- Hopkins Z, Sun M, DeWitt J, Knappe D. 2018. Recently-detected drinking water contaminants: genX and other per‐ and polyfluoroalkyl ether acids. J Am Water Works Assoc. 110(7):13–28. doi: 10.1002/awwa.1073.
- Howe K, Clark M, Torroja C, Torrance J, Berthelot C, Muffato M, Collins J, Humphray S, McLaren K, Matthews L, et al. 2013. The zebrafish reference genome sequence and its relationship to the human genome. Nature. 496(7446):498–503. doi: 10.1038/nature12111.
- Hu X, Andrews D, Lindstrom A, Bruton T, Schaider L, Grandjean P, Lohmann R, Carignan C, Blum A, Balan S, et al. 2016. Detection of poly- and perfluoroalkyl substances (PFASs) in U.S. drinking water linked to industrial sites, military fire training areas, and wastewater treatment plants. Environ Sci Technol Lett. 3(10):344–350. doi: 10.1021/acs.estlett.6b00260.
- Huang J, Wang Q, Liu S, Lai H, Tu W. 2022. Comparative chronic toxicities of PFOS and its novel alternatives on the immune system associated with intestinal microbiota dysbiosis in adult zebrafish. J Hazard Mater. 425:127950. doi: 10.1016/j.jhazmat.2021.127950.
- Huang J, Wang Q, Liu S, Zhang M, Liu Y, Sun L, Wu Y, Tu W. 2021. Crosstalk between histological alterations, oxidative stress and immune aberrations of the emerging PFOS alternative OBS in developing zebrafish. Sci Total Environ. 774:145443. doi: 10.1016/j.scitotenv.2021.145443.
- India-Aldana S, Yao M, Midya V, Colicino E, Chatzi L, Chu J, Gennings C, Jones D, Loos R, Setiawan V, et al. 2023. PFAS exposures and the human metabolome: a systematic review of epidemiological studies. Curr Pollut Rep. 9(3):510–568. doi: 10.1007/s40726-023-00269-4.
- Jiang J, Tu H, Li P. 2022. Lipid metabolism and neutrophil function. Cell Immunol. 377:104546. doi: 10.1016/j.cellimm.2022.104546.
- Jin R, Hao J, Yi Y, Sauter E, Li B. 2021. Regulation of macrophage functions by FABP-mediated inflammatory and metabolic pathways. Biochim Biophys Acta. 1866(8):158964. doi: 10.1016/j.bbalip.2021.158964.
- Keil D, Mehlmann T, Butterworth L, Peden-Adams M. 2008. Gestational exposure to perfluorooctane sulfonate suppresses immune function in B6C3F1 mice. Toxicol Sci. 103(1):77–85. doi: 10.1093/toxsci/kfn015.
- Keiter S, Baumann L, Färber H, Holbech H, Skutlarek D, Engwall M, Braunbeck T. 2012. Long-term effects of a binary mixture of perfluorooctane sulfonate (PFOS) and bisphenol A (BPA) in zebrafish (Danio rerio). Aquat Toxicol. 118-119:116–129. doi: 10.1016/j.aquatox.2012.04.003.
- Khazaee M, Christie E, Cheng W, Michalsen M, Field J, Ng C. 2021. Perfluoroalkyl acid binding with peroxisome proliferator-activated receptors-α, γ, and δ, and fatty acid binding proteins by equilibrium dialysis with a comparison of methods. Toxics. 9(3):45. doi: 10.3390/toxics9030045.
- Knudsen A, Long M, Pedersen H, Bonefeld-Jørgensen E. 2018. Persistent organic pollutants and hematological markers in Greenlandic pregnant women: ACCEPT sub-study. Int J Circumpolar Health. 77(1):1456303. doi: 10.1080/22423982.2018.1456303.
- Kong B, Wang X, He B, Wei L, Zhu J, Jin Y, Fu Z. 2019. 8:2 fluorotelomer alcohol inhibited proliferation and disturbed expression of pro-inflammatory cytokines and antigen-presenting genes in murine macrophages. Chemosphere. 219:1052–1060. doi: 10.1016/j.chemosphere.2018.12.091.
- Lee H, You D, Taylor-Just A, Linder K, Atkins H, Ralph L, de la Cruz G, Bonner J. 2022. Pulmonary exposure of mice to ammonium perfluoro(2-methyl-3-oxahexanoate) (GenX) suppresses the innate immune response to carbon black nanoparticles and stimulates lung cell proliferation. Inhal Toxicol. 34(9–10):244–259. doi: 10.1080/08958378.2022.2086651.
- Lee J, Kim S. 2018. Correlation between mast cell-mediated allergic inflammation and length of perfluorinated compounds. J Toxicol Environ Health A. 81(9):302–313. doi: 10.1080/15287394.2018.1440188.
- Lee J, Lee S, Baek M, Lee B, Lee H, Kwon T, Park P, Shin T, Khang D, Kim S. 2017. Association between perfluorooctanoic acid exposure and degranulation of mast cells in allergic inflammation. J Appl Toxicol. 37(5):554–562. doi: 10.1002/jat.3389.
- Lin H, Wu H, Liu F, Yang H, Shen L, Chen J, Zhang X, Zhong Y, Zhang H, Liu Z. 2022. Assessing hepatotoxicity of PFOA, PFOS, and 6:2 Cl-PFESA in black-spotted frogs (Rana nigromaculata) and elucidating potential association with gut microbiota. Environ Pollut. 312:120029. doi: 10.1016/j.envpol.2022.120029.
- Lindstrom A, Strynar M, Libelo E. 2011. Polyfluorinated compounds: past, present, and future. Environ Sci Technol. 45(19):7954–7961. doi: 10.1021/es2011622.
- Liu C, Wang Q, Liang K, Liu J, Zhou B, Zhang X, Liu H, Giesy J, Yu H. 2013. Effects of tris(1,3-dichloro-2-propyl) phosphate and triphenyl phosphate on receptor-associated mRNA expression in zebrafish embryos/larvae. Aquat Toxicol. 128-129:147–157. doi: 10.1016/j.aquatox.2012.12.010.
- Liu S, Lai H, Wang Q, Martínez R, Zhang M, Liu Y, Huang J, Deng M, Tu W. 2021. Immunotoxicity of F53B, an alternative to PFOS, on zebrafish (Danio rerio) at different early life stages. Sci Total Environ. 790:148165. doi: 10.1016/j.scitotenv.2021.148165.
- Liu Z, Lin H, Zheng Y, Feng Y, Shi C, Zhu R, Shen X, Han Y, Zhang H, Zhong Y. 2023. Perfluorooctanoic acid and perfluorooctanesulfonic acid induce immunotoxicity through the NF-κB pathway in black-spotted frog (Rana nigromaculata). Chemosphere. 313:137622. doi: 10.1016/j.chemosphere.2022.137622.
- Lopez-Espinosa M, Carrizosa C, Luster M, Margolick J, Costa O, Leonardi G, Fletcher T. 2021. Perfluoroalkyl substances and immune cell counts in adults from Mid-Ohio Valley (USA). Environ Int. 156:106599. doi: 10.1016/j.envint.2021.106599.
- Lv Z, Wu W, Ge S, Jia R, Lin T, Yuan Y, Kuang H, Yang B, Wu L, Wei J, et al. 2018. Naringin protects against perfluorooctane sulfonate-induced liver injury by modulating NRF2 and NF-κB in mice. Int Immunopharmacol. 65:140–147. doi: 10.1016/j.intimp.2018.09.019.
- Martínez R, Navarro-Martín L, Luccarelli C, Codina A, Raldúa D, Barata C, Tauler R, Piña B. 2019. Unravelling mechanisms of PFOS toxicity by combining morphological and transcriptomic analyses in zebrafish embryos. Sci Total Environ. 674:462–471. doi: 10.1016/j.scitotenv.2019.04.200.
- Masi M, Maddalon A, Iulini M, Linciano P, Galbiati V, Marinovich M, Racchi M, Corsini E, Buoso E. 2022. Effects of endocrine disrupting chemicals on the expression of RACK1 and LPS-induced THP-1 cell activation. Toxicology. 480:153321. doi: 10.1016/j.tox.2022.153321.
- McMillian M, Nie A, Parker J, Leone A, Kemmerer M, Bryant S, Herlich J, Yieh L, Bittner A, Liu X, et al. 2004. Inverse gene expression patterns for macrophage activating hepatotoxicants and peroxisome proliferators in rat liver. Biochem Pharmacol. 67(11):2141–2165. doi: 10.1016/j.bcp.2004.01.029.
- Miyano Y, Tsukuda S, Sakimoto I, Takeuchi R, Shimura S, Takahashi N, Kusayanagi T, Takakusagi Y, Okado M, Matsumoto Y, et al. 2012. Exploration of the binding proteins of perfluorooctane sulfonate by a T7 phage display screen. Bioorg Med Chem. 20(13):3985–3990. doi: 10.1016/j.bmc.2012.05.016.
- Najjar A, Punt A, Wambaugh J, Paini A, Ellison C, Fragki S, Bianchi E, Zhang F, Westerhout J, Mueller D, et al. 2022. Towards best use and regulatory acceptance of generic physiologically-based kinetic (PBK) models for in vitro-to-in vivo extrapolation (IVIVE) in chemical risk assessment. Arch Toxicol. 96(12):3407–3419. doi: 10.1007/s00204-022-03356-5.
- National Toxicology Program (NTP). 2016. NTP monograph: Immunotoxicity Associated with Exposure to Perfluorooctanoic Acid or Perfluorooctane Sulfonate. Washington (DC): U.S. Department of Health and Human Services.
- National Toxicology Program (NTP). 2019. NTP Technical Report on Toxicity Studies of Perfluoroalkyl Sulfonates (Perfluorobutane Sulfonic Acid, Perfluorohexane Sulfonate Potassium Salt, and Perfluorooctane Sulfonic Acid) Administered by Gavage to Sprague-Dawley (Hsd: Sprague-Dawley, SD) Rats (Revised 2022). Washington (DC): U.S. Department of Health and Human Services.
- Nian M, Zhou W, Feng Y, Wang Y, Chen Q, Zhang J. 2022. Emerging and legacy PFAS and cytokine homeostasis in women of childbearing age. Sci Rep. 12(1):6517. doi: 10.1038/s41598-022-10501-8.
- Organization for Economic Co-operation and Development (OECD). 2018. Environment Directorate Joint Meeting of the Chemicals Committee and the Working Party on Chemicals, Pesticides and Biotechnology. In: Toward a new comprehensive global database of per- and polyfluoroalkyl substances (PFASs): Summary report on updating the OECD 2007 list of per- and polyfluoroalkyl substances (PFASs). Series on Risk Management No. 39. Paris: The Inter-Organization Program for Sound Management of Chemicals (IOMC).
- Oulhote Y, Shamim Z, Kielsen K, Weihe P, Grandjean P, Ryder L, Heilmann C. 2017. Childrens’ white blood cell counts in relation to developmental exposures to methylmercury and persistent organic pollutants. Reprod Toxicol. 68:207–214. doi: 10.1016/j.reprotox.2016.08.001.
- Park S, Sim K, Shrestha P, Yang J, Lee Y. 2021. Perfluorooctane sulfonate and bisphenol A induce a similar level of mast cell activation via a common signaling pathway, Fyn-Lyn-Syk activation. Food Chem Toxicol. 156:112478. doi: 10.1016/j.fct.2021.112478.
- Passante E, Frankish N. 2009. The RBL-2H3 cell line: its provenance and suitability as a model for the mast cell. Inflamm Res. 58(11):737–745. doi: 10.1007/s00011-009-0074-y.
- Pecquet A, Maier A, Kasper S, Sumanas S, Yadav J. 2020. Exposure to perfluorooctanoic acid (PFOA) decreases neutrophil migration response to injury in zebrafish embryos. BMC Res Notes. 13(1):408. doi: 10.1186/s13104-020-05255-3.
- Peden-Adams M, Keller J, Eudaly J, Berger J, Gilkeson G, Keil D. 2008. Suppression of humoral immunity in mice following exposure to perfluorooctane sulfonate. Toxicol Sci. 104(1):144–154. doi: 10.1093/toxsci/kfn059.
- Peden-Adams M, Stuckey J, Gaworecki K, Berger-Ritchie J, Bryant K, Jodice P, Scott T, Ferrario J, Guan B, Vigo C, et al. 2009. Developmental toxicity in white leghorn chickens following in ovo exposure to perfluorooctane sulfonate (PFOS). Reprod Toxicol. 27(3-4):307–318. doi: 10.1016/j.reprotox.2008.10.009.
- Pennings J, Jennen D, Nygaard U, Namork E, Haug L, van Loveren H, Granum B. 2016. Cord blood gene expression supports that prenatal exposure to perfluoroalkyl substances causes depressed immune functionality in early childhood. J Immunotoxicol. 13(2):173–180. doi: 10.3109/1547691X.2015.1029147.
- Phelps D, Fletcher A, Rodriguez-Nunez I, Balik-Meisner M, Tokarz D, Reif D, Germolec D, Yoder J. 2020. In vivo assessment of respiratory burst inhibition by xenobiotic exposure using larval zebrafish. J Immunotoxicol. 17(1):94–104. doi: 10.1080/1547691X.2020.1748772.
- Phelps D, Palekar A, Conley H, Ferrero G, Driggers J, Linder K, Kullman S, Reif D, Sheats M, DeWitt J, et al. 2023. Legacy and emerging per- and polyfluoroalkyl substances suppress the neutrophil respiratory burst. J Immunotoxicol. 20:2176953.
- Phelps D. 2022. Investigating the neutrophil respiratory burst as a target of xenobiotics [Doctoral dissertation]. North Carolina State University. https://repository.lib.ncsu.edu/server/api/core/bitstreams/f369be63-3f04-41d3-b73b-07098c8fb2d1/content
- Pierozan P, Jerneren F, Karlsson O. 2018. Perfluorooctanoic acid (PFOA) exposure promotes proliferation, migration and invasion potential in human breast epithelial cells. Arch Toxicol. 92(5):1729–1739. doi: 10.1007/s00204-018-2181-4.
- Pierozan P, Karlsson O. 2018. PFOS induces proliferation, cell-cycle progression, and malignant phenotype in human breast epithelial cells. Arch Toxicol. 92(2):705–716. doi: 10.1007/s00204-017-2077-8.
- Pierpont T, Limper C, Elmore J, Redko A, Anannya O, Imbiakha B, Villanueva A, Anronikov S, Bondah N, Chang S, et al. 2023. Effects of PFOS and cyclophosphamide exposure on immune homeostasis in mice. Immunobiology. 228(3):152356. doi: 10.1016/j.imbio.2023.152356.
- Planchart A, Mattingly C, Allen D, Ceger P, Casey W, Hinton D, Kanungo J, Kullman S, Tal T, Bondesson M, et al. 2016. Advancing toxicology research using in vivo high-throughput toxicology with small fish models. ALTEX. 33(4):435–452. doi: 10.14573/altex.1601281.
- Poothong S, Papadopoulou E, Padilla-Sánchez J, Thomsen C, Haug L. 2020. Multiple pathways of human exposure to poly- and perfluoroalkyl substances (PFASs): from external exposure to human blood. Environ Int. 134:105244. doi: 10.1016/j.envint.2019.105244.
- Qazi M, Abedi M, Nelson B, DePierre J, Abedi-Valugerdi M. 2010a. Dietary exposure to perfluorooctanoate or perfluorooctane sulfonate induces hypertrophy in centrilobular hepatocytes and alters the hepatic immune status in mice. Toxicol Lett. 219(1):1–7. doi: 10.1016/j.intimp.2010.08.009.
- Qazi M, Bogdanska J, Butenhoff J, Nelson B, DePierre J, Abedi-Valugerdi M. 2009. High-dose, short-term exposure of mice to perfluorooctanesulfonate (PFOS) or perfluorooctanoate (PFOA) affects number of circulating neutrophils differently, but enhances the inflammatory responses of macrophages to lipopolysaccharide (LPS) in a similar fashion. Toxicology. 262(3):207–214. doi: 10.1016/j.tox.2009.06.010.
- Qazi M, Nelson B, Depierre J, Abedi-Valugerdi M. 2010b. 28-Day dietary exposure of mice to a low total dose (7 mg/kg) of perfluorooctanesulfonate (PFOS) alters neither cellular compositions of the thymus and spleen nor humoral immune responses: does route of administration play a pivotal role in PFOS-induced immunotoxicity? Toxicology. 267(1-3):132–139. doi: 10.1016/j.tox.2009.10.035.
- Qazi M, Nelson B, DePierre J, Abedi-Valugerdi M. 2012. High-dose dietary exposure of mice to perfluorooctanoate or perfluorooctane sulfonate exerts toxic effects on myeloid and B-lymphoid cells in the bone marrow and these effects are partially dependent on reduced food consumption. Food Chem Toxicol. 50(9):2955–2963. doi: 10.1016/j.fct.2012.06.023.
- Qin Y, Gu T, Ling J, Luo J, Zhao J, Hu B, Hua L, Wan C, Jiang S. 2022. PFOS facilitates liver inflammation and steatosis: An involvement of NLRP3 inflammasome-mediated hepatocyte pyroptosis. J Appl Toxicol. 42(5):806–817. doi: 10.1002/jat.4258.
- Racanelli V, Rehermann B. 2006. The liver as immunological organ. Hepatology. 43(2 Suppl 1):S54–S62. doi: 10.1002/hep.21060.
- Racchi M, Buoso E, Ronfani M, Serafini M, Galasso M, Lanni C, Corsini E. 2017. Role of hormones in regulation of RACK1 expression as signaling checkpoint in immunosenescence. IJMS. 18(7):1453. doi: 10.3390/ijms18071453.
- Rericha Y, Simonich M, Truong L, Tanguay R. 2023. Review of zebrafish as a model to investigate per- and polyfluoroalkyl substance toxicity. Toxicol Sci. 194(2):138–152. doi: 10.1093/toxsci/kfad051.
- Rockwell C, Turley A, Cheng X, Fields P, Klaassen C. 2013. Acute immunotoxic effects of perfluorononanoic acid (PFNA) in C57Bl/6 Mice. Clin Exp Pharmacol. 4(Suppl 4):S4–S002. doi: 10.4172/2161-1459.S4-002.
- Rockwell C, Turley A, Cheng X, Fields P, Klaassen C. 2017. Persistent alterations in immune cell populations and function from a single dose of perfluorononanoic acid (PFNA) in C57Bl/6 mice. Food Chem Toxicol. 100:24–33. doi: 10.1016/j.fct.2016.12.004.
- Rodríguez-Jorquera I, Colli-Dula R, Kroll K, Jayasinghe B, Parachu Marco M, Silva-Sanchez C, Toor G, Denslow N. 2019. Blood transcriptomics analysis of fish exposed to perfluoro-alkyl substances: Assessment of a non-lethal sampling technique for advancing aquatic toxicology research. Environ Sci Technol. 53(3):1441–1452. doi: 10.1021/acs.est.8b03603.
- Rowan-Carroll A, Reardon A, Leingartner K, Gagné R, Williams A, Meier M, Kuo B, Bourdon-Lacombe J, Moffat I, Carrier R, et al. 2021. High-throughput transcriptomic analysis of human primary hepatocyte spheroids exposed to per- and polyfluoroalkyl substances as a platform for relative potency characterization. Toxicol Sci. 181(2):199–214. doi: 10.1093/toxsci/kfab039.
- Shane H, Baur R, Lukomska E, Weatherly L, Anderson S. 2020. Immunotoxicity and allergenic potential induced by topical application of perfluorooctanoic acid (PFOA) in a murine model. Food Chem Toxicol. 136:111114. doi: 10.1016/j.fct.2020.111114.
- Sheng N, Zhou X, Zheng F, Pan Y, Guo X, Guo Y, Sun Y, Dai J. 2017. Comparative hepatotoxicity of 6:2 fluorotelomer carboxylic acid and 6:2 fluorotelomer sulfonic acid, two fluorinated alternatives to long-chain perfluoroalkyl acids, on adult male mice. Arch Toxicol. 91(8):2909–2919. doi: 10.1007/s00204-016-1917-2.
- Shi G, Xie Y, Guo Y, Dai J. 2018. 6:2 fluorotelomer sulfonamide alkylbetaine (6:2 FTAB), a novel perfluorooctane sulfonate alternative, induced developmental toxicity in zebrafish embryos. Aquat Toxicol. 195:24–32. doi: 10.1016/j.aquatox.2017.12.002.
- Singh T, Lee S, Kim H, Choi J, Kim S. 2012. Perfluorooctanoic acid induces mast cell-mediated allergic inflammation by the release of histamine and inflammatory mediators. Toxicol Lett. 210(1):64–70. doi: 10.1016/j.toxlet.2012.01.014.
- Søderstrøm S, Lille-Langøy R, Yadetie F, Rauch M, Milinski A, Dejaegere A, Stote R, Goksøyr A, Karlsen O. 2022. Agonistic and potentiating effects of perfluoroalkyl substances (PFAS) on the Atlantic cod (Gadus morhua) peroxisome proliferator-activated receptors (PPARs). Environ Int. 163:107203. doi: 10.1016/j.envint.2022.107203.
- Sun X, Xie Y, Zhang X, Song J, Wu Y. 2023. Estimation of per- and polyfluorinated alkyl substance induction equivalency factors for humpback dolphins by transactivation potencies of peroxisome proliferator-activated receptors. Environ Sci Technol. 57(9):3713–3721. doi: 10.1021/acs.est.2c05044.
- Surace L, di Santo J. 2022. Local and systemic features of ILC immunometabolism. Curr Opin Hematol. 29(4):209–217. doi: 10.1097/MOH.0000000000000722.
- Tang L, Qiu W, Zhang S, Wang J, Yang X, Xu B, Magnuson J, Xu E, Wu M, Zheng C. 2023. Poly- and perfluoroalkyl substances induce immunotoxicity via the TLR Pathway in zebrafish: links to carbon chain length. Environ Sci Technol. 57(15):6139–6149. doi: 10.1021/acs.est.2c09716.
- Taylor K, Woodlief T, Ahmed A, Hu Q, Duncker P, DeWitt J. 2023. Quantifying the impact of PFOA exposure on B-cell development and antibody production. Toxicol Sci. 194(1):101–108. doi: 10.1093/toxsci/kfad043.
- Tian J, Hong Y, Li Z, Yang Z, Lei B, Liu J, Cai Z. 2021. Immunometabolism-modulation and immunotoxicity evaluation of perfluorooctanoic acid in macrophage. Ecotoxicol Environ Saf. 215:112128. doi: 10.1016/j.ecoenv.2021.112128.
- Toobian D, Ghosh P, Katkar G. 2021. Parsing the role of PPARs in macrophage processes. Front Immunol. 12:783780. doi: 10.3389/fimmu.2021.783780.
- Torres L, Redko A, Limper C, Imbiakha B, Chang S, August A. 2021. Effect of perfluorooctane-sulfonic acid (PFOS) on immune cell development and function in mice. Immunol Lett. 233:31–41. doi: 10.1016/j.imlet.2021.03.006.
- Traver D, Herbomel P, Patton E, Murphey R, Yoder J, Litman G, Catic A, Amemiya C, Zon L, Trede N. 2003. The zebrafish as a model organism to study development of the immune system. Adv Immunol. 81:253–330.
- Traver D, Yoder J. 2020. Chapter 19 – Immunology. In: Cartner S, Eisen J, Farmer S, Guillemin K, Kent M, Sanders G, editors. The Zebrafish in Biomedical Research. New York: Academic Press; p. 191–216.
- USEPA (United States Environmental Protection Agency). 2019. EPA’s per- and polyfluoroalkyl substances (PFAS) action plan. U.S. Environmental Protection Agency. https://www.epa.gov/sites/default/files/2019-02/documents/pfas_action_plan_021319_508compliant_1.pdf
- USEPA. 2022. INTERIM Drinking Water Health Advisory: perfluorooctane Sulfonic Acid (PFOS) CASRN 1763-23-1. Washington, DC: U.S. Environmental Protection Agency.
- USEPA. 2023a. Navigation panel to PFAS structure lists: CompTox Chemicals Dashboard v2.2.1. U.S. Environmental Protection Agency. https://comptox.epa.gov/dashboard/chemical-lists/PFASSTRUCT (Accessed November 2023)
- USEPA. 2023b. Fact Sheet: 2010/2015 PFOA Stewardship Program. U.S. Environmental Protection Agency. https://www.epa.gov/assessing-and-managing-chemicals-under-tsca/fact-sheet-20102015-pfoa-stewardship-program (Accessed October 2023)
- Vallabhapurapu S, Karin M. 2009. Regulation and function of NF-κB transcription factors in the immune system. Annu Rev Immunol. 27(1):693–733. doi: 10.1146/annurev.immunol.021908.132641.
- Vetvicka V, Vetvickova J. 2013. Reversal of perfluorooctanesulfonate-induced immunotoxicity by a glucan-resveratrol-Vitamin C combination. Orient Pharm Exp Med. 13(1):77–84. doi: 10.1007/s13596-013-0105-7.
- Virmani R, Fink L, Gunter K, English D. 1984. Effect of perfluorochemical blood substitutes on human neutrophil function. Transfusion. 24(4):343–347. doi: 10.1046/j.1537-2995.1984.24484275579.x.
- Virmani R, Warren D, Rees R, Fink L, English D. 1983. Effects of perfluorochemical on phagocytic function of leukocytes. Transfusion. 23(6):512–515. doi: 10.1046/j.1537-2995.1983.23684074274.x.
- Wan C, Gu T, Ling J, Qin Y, Luo J, Sun L, Hua L, Zhao J, Jiang S. 2022. Perfluorooctane sulfonate aggravates CCl4-induced hepatic fibrosis via HMGB1/TLR4/Smad signaling. Environ Toxicol. 37(5):983–994. doi: 10.1002/tox.23458.
- Wang C, Nie X, Zhang Y, Li T, Mao J, Liu X, Gu Y, Shi J, Xiao J, Wan C, et al. 2015. Reactive oxygen species mediate nitric oxide production through ERK/JNK MAPK signaling in HAPI microglia after PFOS exposure. Toxicol Appl Pharmacol. 288(2):143–151. doi: 10.1016/j.taap.2015.06.012.
- Wang D, Tan Z, Yang J, Li L, Li H, Zhang H, Liu H, Liu Y, Wang L, Li Q, et al. 2023. Perfluorooctane sulfonate promotes atherosclerosis by modulating M1 polarization of macrophages through the NF-κB pathway. Ecotoxicol Environ Saf. 249:114384. doi: 10.1016/j.ecoenv.2022.114384.
- Wang L-Q, Liu T, Yang S, Sun L, Zhao Z-Y, Li L-Y, She Y-C, Zheng Y-Y, Ye X-Y, Bao Q, et al. 2021. Perfluoroalkyl substance pollutants activate the innate immune system through the AIM2 inflammasome. Nat Commun. 12(1):2915. doi: 10.1038/s41467-021-23201-0.
- Wang Q, Huang J, Liu S, Wang C, Jin Y, Lai H, Tu W. 2022. Aberrant hepatic lipid metabolism associated with gut microbiota dysbiosis triggers hepatotoxicity of novel PFOS alternatives in adult zebrafish. Environ Int. 166:107351. doi: 10.1016/j.envint.2022.107351.
- Wang T, Zhao X, Liu T, Zhang J, Qiu J, Li M, Weng R. 2023. Transcriptional investigation of the toxic mechanisms of perfluorooctane sulfonate in rats based on an RNA-Seq approach. Chemosphere. 329:138629. doi: 10.1016/j.chemosphere.2023.138629.
- Wang X, Zhou C, He B, Kong B, Wei L, Wang R, Lin J, Shao Y, Zhu J, Jin Y, et al. 2019. 8:2 Fluorotelomer alcohol causes G1 cell cycle arrest and blocks granulocytic differentiation in HL-60 cells. Environ Toxicol. 34(5):666–673. doi: 10.1002/tox.22733.
- Wang Z, Buser A, Cousins I, Demattio S, Drost W, Johansson O, Ohno K, Patlewicz G, Richard A, Walker G, et al. 2021. A new OECD definition for per- and polyfluoroalkyl substances. Environ Sci Technol. 55(23):15575–15578. doi: 10.1021/acs.est.1c06896.
- Wang Z, DeWitt J, Higgins C, Cousins I. 2017. A never-ending story of per- and polyfluoroalkyl substances (PFASs)? Environ Sci Technol. 51(5):2508–2518. doi: 10.1021/acs.est.6b04806.
- Weatherly L, Shane H, Lukomska E, Baur R, Anderson S. 2021. Systemic toxicity induced by topical application of heptafluorobutyric acid (PFBA) in a murine model. Food Chem Toxicol. 156:112528. doi: 10.1016/j.fct.2021.112528.
- Williams A, Grulke C, Edwards J, McEachran A, Mansouri K, Baker N, Patlewicz G, Shah I, Wambaugh J, Judson R, et al. 2017. The CompTox chemistry dashboard: A community data resource for environmental chemistry. J Cheminform. 9(1):61. doi: 10.1186/s13321-017-0247-6.
- Woodlief T, Vance S, Hu Q, DeWitt J. 2021. Immunotoxicity of per- and polyfluoroalkyl substances: insights into short-chain PFAS Exposure. Toxics. 11(8):100. doi: 10.3390/toxics11080656.
- Wu X, Liang M, Yang Z, Su M, Yang B. 2017. Effect of acute exposure to PFOA on mouse liver cells in vivo and in vitro. Environ Sci Pollut Res Int. 24(31):24201–24206. doi: 10.1007/s11356-017-0072-5.
- Xie X, Zhou J, Hu L, Shu R, Zhang M, Xiong Z, Wu F, Fu Z. 2021. Exposure to hexafluoro-propylene oxide dimer acid (HFPO-DA) disturbs the gut barrier function and gut microbiota in mice. Environ Pollut. 290:117934. doi: 10.1016/j.envpol.2021.117934.
- Xu B, Chen L, Zhan Y, Marquez K, Zhuo L, Qi S, Zhu J, He Y, Chen X, Zhang H, et al. 2022. Biological functions and regulatory mechanisms of fatty acid binding protein-5 in various diseases. Front Cell Devel Biol. 10:857919.
- Xu L, Chen Y, Zhang Q, Chen L, Zhang K, Li J, Liu J, Wang Q, Xie X. 2022. Gestational exposure to GenX induces hepatic alterations by the gut-liver axis in maternal mice: A similar mechanism as PFOA. Sci Total Environ. 820:153281. doi: 10.1016/j.scitotenv.2022.153281.
- Yamaki K, Yoshino S. 2010. Enhancement of FcεRI-mediated degranulation response in the rat basophilic leukemia cell line RBL2H3 by the fluoro-surfactants perfluorooctanoic acid and perfluorooctane sulfonate. Environ Toxicol Pharmacol. 29(2):183–189. doi: 10.1016/j.etap.2009.12.009.
- Yan J, Horng T. 2020. Lipid metabolism in regulation of macrophage functions. Trends Cell Biol. 30(12):979–989. doi: 10.1016/j.tcb.2020.09.006.
- Yang H, Lai H, Huang J, Sun L, Mennigen J, Wang Q, Liu Y, Jin Y, Tu W. 2020. Polystyrene microplastics decrease F-53B bioaccumulation but induce inflammatory stress in larval zebrafish. Chemosphere. 255:127040. doi: 10.1016/j.chemosphere.2020.127040.
- Yang J, Wang C, Nie X, Shi S, Xiao J, Ma X, Dong X, Zhang Y, Han J, Li T, et al. 2015. Perfluorooctane sulfonate mediates microglial activation and secretion of TNFα through Ca2+-dependent PKC-NF-κB signaling. Int Immunopharmacol. 28(1):52–60. doi: 10.1016/j.intimp.2015.05.019.
- Yu J, Cheng W, Jia M, Chen L, Gu C, Ren H-Q, Wu B. 2022. Toxicity of perfluorooctanoic acid on zebrafish early embryonic development determined by single-cell RNA sequencing. J Hazard Mater. 427:127888. doi: 10.1016/j.jhazmat.2021.127888.
- Zhang H, Fang W, Wang D, Gao N, Ding Y, Chen C. 2014. The role of interleukin family in perfluorooctanoic acid (PFOA)-induced immunotoxicity. J Hazard Mater. 280:552–560. doi: 10.1016/j.jhazmat.2014.08.043.
- Zhang H, Shen L, Fang W, Zhang X, Zhong Y. 2021. Perfluorooctanoic acid-induced immunotoxicity via NF-κB pathway in zebrafish (Danio rerio) kidney. Fish Shellfish Immunol. 113:9–19. doi: 10.1016/j.fsi.2021.03.004.
- Zhang J, Ren Z, Chen M. 2023. Immunotoxicity and transcriptome analyses of zebrafish (Danio rerio) embryos exposed to 6:2 FTSA. Toxics. 11(5):459. doi: 10.3390/toxics11050459.
- Zhang L, Louie A, Rigutto G, Guo H, Zhao Y, Ahn S, Dahlberg S, Sholinbeck M, Smith M. 2023. A systematic evidence map of chronic inflammation and immunosuppression related to per- and polyfluoroalkyl substance (PFAS) exposure. Environ Res. 220:115188. doi: 10.1016/j.envres.2022.115188.
- Zhang L, Sun W, Chen H, Zhang Z, Cai W. 2020. Transcriptomic changes in liver of juvenile Cynoglossus semilaevis following perfluorooctane sulfonate exposure. Enviro Toxic and Chemistry. 39(3):556–564. doi: 10.1002/etc.4633.
- Zhang Q, Dong X, Lu J, Song J, Wang Y. 2021. Chemoproteomic approach toward probing the interactomes of perfluoroalkyl substances. Anal Chem. 93(27):9634–9639. doi: 10.1021/acs.analchem.1c01948.
- Zhao Y, Jin H, Qu J, Zhang S, Hu S, Xue J, Zhao M. 2022. The influences of perfluoroalkyl substances on the rheumatoid arthritis clinic. BMC Immunol. 23(1):10. doi: 10.1186/s12865-022-00483-7.
- Zheng L, Dong G, Jin Y, He Q. 2009. Immunotoxic changes associated with a 7-day oral exposure to perfluorooctanesulfonate (PFOS) in adult male C57Bl/6 mice. Arch Toxicol. 83(7):679–689. doi: 10.1007/s00204-008-0361-3.
- Zhong Y, Shen L, Ye X, Zhou D, He Y, Zhang H. 2020. Mechanism of immunosuppression in zebrafish (Danio rerio) spleen induced by environmentally-relevant concentrations of perfluorooctanoic acid. Chemosphere. 249:126200. doi: 10.1016/j.chemosphere.2020.126200.
- Zhu J, Qian W, Wang Y, Gao R, Wang J, Xiao H. 2015. Involvement of mitogen-activated protein kinase and NF-κB signaling pathways in perfluorooctane sulfonic acid-induced inflammatory reaction in BV2 microglial cells. J Appl Toxicol. 35(12):1539–1549. doi: 10.1002/jat.3119.