ABSTRACT
The construction of bioactive scaffolds with a suitable microenvironment for tissue regeneration provides a great promise for improving clinical treatment of osteochondral and full-thickness articular cartilage defects. Hydrogels of polysaccharides, such as alginate, agarose, chitosan, cellulose, hyaluronic acid, and dextran, biomimic the structure of the extracellular matrix (ECM), are exceptionally biocompatible scaffold material for tissue regeneration. The application of polysaccharide hydrogels combined with 3-dimensional (3D) printing technology can precisely distribute cell-loaded biomaterials, construct complex 3D living tissues with optimal structure and mechanical properties for osteochondral and cartilage repair. This review highlights recent advances in the development of polysaccharide-based hydrogel materials for promoting bone and cartilage tissue repair. We also highlight recent advances in 3D bioprinting polysaccharide-based hydrogel materials in cartilage regeneration. The cell type and the development of 3D bioprinting technology were described. In addition, the formation of polysaccharide – protein-based is also discussed. We outline the future trends of 3D printing including machine learning, near-infrared photopolymerization, 4D printing, and a combination of self-assembly and live-cell 3D printing-based methods.
1. Introduction
Articular cartilage and osteochondral tissue are important components of human skeletal system. Osteochondral and cartilage defects resulting from trauma, degenerative disease, congenital defect, tumor and infection (osteomyelitis) is a worldwide health problem, which brings heavy economic burden to both society and patients [Citation1,Citation2]. Although the osteochondral has a strong ability of self-repair, when the osteochondral defect exceeds its self-healing ability, it is necessary to take appropriate implants to replace the defect structure in order to restore the integrity of osteochondral interface structure and function [Citation3]. As for cartilage, the proliferation ability of chondrocytes is very weak, basically has no ability of self-replication and proliferation, thus it cannot repair the cartilage defect after injury. More than 900 million bone and cartilage reconstruction operations are performed worldwide each year to deal with all these bone and cartilage defects [Citation4]. In addition, strategies for repairing bone and cartilage defects, including orthopedic surgery and drug therapy cannot successfully perform bone and cartilage reconstruction [Citation2].
At present, the traditional transplantation materials for osteochondral and cartilage are autogenously bone, allogeneic bone and artificial bone, in which the source of autogenously bone is limited and will cause secondary injury of the donor site or the potential infection. Artificial bone has some shortcomings, such as structural size is difficult to match and immune rejection. In this context, repair of cartilage and osteochondral interface defects using a biomimetic scaffold of great value. Osteochondral and cartilage engineering refers to transplanting seed cells cultured in vitro into scaffold materials, and then implanting the materials into bone defects, complemented by cell growth factor to stimulate cell proliferation and differentiation to repair cartilage defects [Citation5]. The development of scaffold materials must therefore be biocompatibility and biodegradable materials. At present, polymer scaffolds, especially hydrogels, have shown great potential in the field of tissue repair because of their three-dimensional reticular structure are similar to human tissue, with high swelling and good biocompatibility. Natural polysaccharide polymers have a wide range of raw materials, safe and non-toxic, and the chemical structure is easy to modify. The hydrogel based on natural polysaccharides is is perfect for osteochondral and cartilage repair [Citation6,Citation7].
An interesting method for preparing scaffolds is to use hydrogels as biomaterials by 3D bio-printing or rapid prototyping (RP) technology. The comprehensive potential of hydrogel and 3D biological printing technology allows the rapid preparation of bionic artificial bone tissue with shape and microstructure matching with defects, which has a good effect in repairing bone defects and can solve the problem of poor matching of traditional implants.
Recently, bioprinting involves the processing of functional biological structures combined with living cells, is increasingly used in articular cartilage and osteochondral tissue engineering [Citation8]. In this review, we outline the latest research trends on polysaccharide-based hydrogel types, cell types and growth factor for effective cartilage formation and osteogenesis. We present our views on the latest development of next-generation matrix with improved physical and biological functions for osteochondral/cartilage tissue engineering. The advanced development of polysaccharide-based hydrogels in biological manufacturing technologies is emphasized, which is used to produce hydrogel-based osteochondral and cartilage structures with complex components and microstructures to imitate their natural counterparts. On this basis, the future development and research direction are prospected, in order to provide reference for the clinical transformation of cartilage tissue engineering regeneration and cartilage repair.
2. Polysaccharide-based hydrogel
Polysaccharides extracted from animals and plants are the preferred basic materials for the development of stents and implants [Citation9,Citation10]. There are various kinds of polysaccharides, and the properties of different kinds of polysaccharides are very different due to the differences of functional groups and polymer chain length. Polysaccharide-based hydrogels are fabricated by cross-linking polysaccharide derivatives with various functional molecules. These hydrogels usually exhibit physical and chemical properties, which are very different from those of macromolecular components in terms of mechanical strength, limited elongation or reproducibility and poor toughness [Citation11]. Through chemical modification of polysaccharides, excellent mechanical properties, physiological signals and minimal tissue reaction can be obtained. In recent years, researchers have prepared a variety of polysaccharide-based hydrogels using sodium alginate, agarose, chitosan, cellulose, glucan and hyaluronic acid, and obtaining positive results. The structure and performance of the above mentioned natural polysaccharide hydrogel materials are summarized in and .
Figure 1. Represent chemical structure of polysaccharide-based hydrogels. agarose is consisting of β-(1–4) (3pc6)-dehydrated-L-galactose (left) and α-(1–3)-D-galactose (right). Hyaluronic acid (HA) is composed of alternating residues of β-D-(1–3) glucuronic acid (left) and β-D-(1–4)-N-acetylglucosamine (right). Alginate is composed of a linear copolymer of β- (1–4)-linked l-guluronic acid units and α-L-guluronic acid residues.
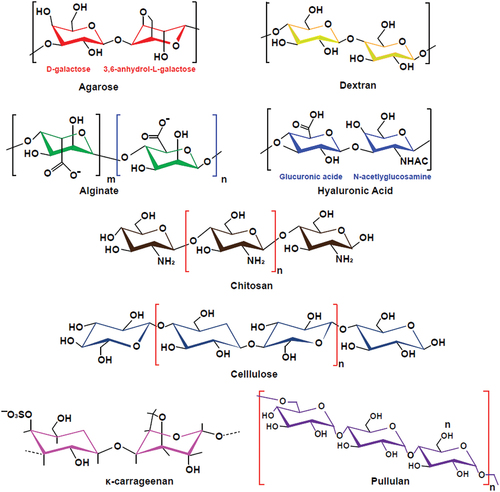
Table 1. Summary of different polysaccharide-based hydrogel as bioinks for osteochondral and cartilage tissue engineering.
2.1. Alginate-based hydrogel
Alginate is a polysaccharide assembly of β-D-manuronic acid and α-L-gulonic acid residues linked by β-(1–4). It exists naturally in the cell wall of algae and in the capsules of nitrogen-fixing bacteria and Pseudomonas aeruginosa, so it can be easy obtained from brown algae and bacterial algae [Citation33,Citation34]. In addition, biosynthesis can fabricate alginate with a clearer chemical structure than alginate extracted from algae, which was considered to be a potential way to produce this polysaccharide [Citation35,Citation36]. Alginate has been widely used in biomedical fields such as wound dressing, drug delivery, biodegradation and tissue engineering because of its excellent biocompatibility, biodegradability and gel forming ability. Yan et al. used alginate as matrix to obtain injectable and biodegradable composite injectable hydrogels by in-situ release of calcium ions and double cross-linking of hydroxyapatite (HAP) and gelatin microspheres (GMs), which can effectively promote the activity of osteoblasts [Citation37]. Alginate can be converted into ideal bioinks for 3D printing by adding divalent cations such as Ca2+ [Citation38]. Stem cell-based alginate 3D bio-printing structures have been used to construct different tissue engineering tissues, including bone, cartilage, heart and blood vessels [Citation39,Citation40]. However, there are two main drawbacks that hinder this future development: one is the loss of shape fidelity and integrity due to weak mechanical properties after printing, and the other is that the printing process will damage the vitality of stem cells and affect their adhesion and proliferation. To solve these problems, different biomaterials are mixed with alginate to form alginate composites to improve properties and meet the requirements of tissue engineering. In order to improve printability and structural stability, Goeun Choe et al added graphene oxide as bioinks for their potential of alginate hydrogel, indicating that the addition of graphene oxide can promte printing and structure properties, which can induce the proliferation and osteogenic differentiation of Mesenchymal stem/stromal cells (MSCs). The results show that the optimal ratio between alginate and graphene oxide is 3% and 0.5 mg ml-1 [Citation39]. Similarly, the addition of graphene oxide to the sodium alginate hydrogel not only emphasized the shape fidelity and resolution of 3D scaffolds, but also triggers cartilage differentiation of human adipose-derived MSCs (hAD-MSCs) [Citation41]. In order to form 3D bone-like scaffold to support osteogenic differentiation and bone-like tissue formation. Zhang et al formed 3D bioprinted cell-laden scaffolds with different mechanical moduli by using different alginate concentrations and cell density. This soft scaffold has higher cell proliferation and differentiation capacity, stronger ability to promote mineralized bone-like tissue formation () [Citation42]. Additional studies was investigate the modify sodium alginate by supplementing it with decellularized extracellular matrix (dECM) extracted from porcine bone tissue. The printability and viability as well as osteogenic differentiation of the hAD-MSCs encapsulated in this scaffold was significant improved. However, due to the large molecular weight of alginate lead it’s difficult to degrade, and the lack of binding sites to react with amino acids and proteins as well as weak mechanical strength, the application of pure alginate in osteochondral and cartilage tissue engineering is limited. In order to further enhance the properties of alginate, it may be another option to introduce more active groups or side chain molecules to modify sodium alginate [Citation43]. Wei et al. prepared a novel self-repairing injectable hydrogel based on biocompatible polysaccharides by using the dynamic reaction of N-carboxyethyl chitosan (CEC) with adipic acid and the aldehyde structure of oxidized sodium alginate [Citation44]. The coexistence of a variety of dynamic covalent bonds in the system gives the hydrogel excellent mechanical properties and self-repairing ability. In addition, the hydrogel has enhanced biocompatibility and cell release ability, thus has a broad application prospect. Balakrishnan et al. completed the self-crosslinking of oxidized alginate and gelatin in the presence of borax, and prepared a rapid gel oxidized alginate-based injectable hydrogel [Citation45]. The results demonstrate that the proliferative activity and migration ability of chondrocytes encapsulated in the hydrogel greatly enhanced. In addition, double cross-linked alginate microgels that composed of oxidized and methacrylic acid alginate (OMA), which are directly assembled into 3D structures and cryopreserved for long-term storage, while stem cells can remain equivalent to newly treated stem cells after recovery, which provides a new solution for 3D bioprinting [Citation46].
Figure 2. Bioink preparation and 3D bioprint assembly with different alginate concentrations and it’s effects on bone mineralization and osteoblast maturation. Adapted from the study from ref [Citation42] with permission of copyright © 2023 elsevier B.V., open access.
![Figure 2. Bioink preparation and 3D bioprint assembly with different alginate concentrations and it’s effects on bone mineralization and osteoblast maturation. Adapted from the study from ref [Citation42] with permission of copyright © 2023 elsevier B.V., open access.](/cms/asset/071fc577-6d59-4fd9-be7c-65bea9cc197b/tdmp_a_2284482_f0002_oc.jpg)
2.2. Agarose hydrogel
Agarose is a natural linear polysaccharide exists in red algae and server as one of the main components of Agar. It consists of alternating β-D-galactose and 3pyrylgalactose units. Because of its biocompatibility, controllable self-gel, water solubility and adaptable mechanical properties, it has been widely used in cartilage formation and bone regeneration [Citation47,Citation48]. In addition, the porous structure, adjustable mechanical strength and hardness of agarose-based hydrogels help adjust the 3D scaffolds of cell culture, which can support cell adhesion, proliferation and activity, making agarose a promising cell-loaded 3D printing hydrogel.
Lopez Marcial et al reported that alginate-based bio-inks were successfully used for extrusion bio-printing of high shape fidelity structures of engineered complex cartilage tissues without additional cross-linking steps [Citation49]. In addition, they reported that the addition of sodium alginate to the agarose gel improved shear thinning, yield strength and print shape fidelity compared with agarose gel alone. The optimize printing performance, cell viability and sGAG yield were obtained by using 5% agarose-sodium alginate-based biological ink, and the results showed agarose and sodium alginate hydrogel have excellent cell viability and matrix production during the 28-day culture period. Compared with pure sodium alginate hydrogel, it has been demonstrated that mixing type I collagen (COL I) and agarose into sodium alginate hydrogel can effectively enhance the mechanical strength of the hydrogel, whereas COL I can also promote cell adhesion, proliferation and cartilage-specific gene expression [Citation50]. The results of Marius et al also showed that mixed hydrogels with a concentration of 0.5% agarose and 0.2% type I collagen had better hardness and printing accuracy, cell diffusion and adhesion [Citation51]. In order to refine the bioinks ratio and the limited size of the printing structure, Daniela et al encapsulated hMSC and MG-63 cells in agarose hydrogels and immersed them with high-density fluorocarbon compounds to create stem cell-loaded scaffolds of different shapes and sizes, which maintained high cell viability after 21 days of culture [Citation52]. In order to further enhance cell activity, silk fibroin/agarose mixture has been utilized as an alternative biomaterial for bone and cartilage tissue [Citation53,Citation54]. Similarly results have shown that human elastic cartilage-derived chondrocytes (HECDC) encapsulated with biodegradable nanostructured fibrin-agarose hydrogels can employe for biodegradable and bioactive tissue engineering [Citation55].
2.3. Chitosan hydrogels
Chitosan is a polymer composed of D-glucosamine and N-acetyl glucosamine, which is extracted from chitin deacetylation in shrimp, crab and coral. Chitosan has a very similar chemical structure to glycosaminoglycan in cartilage matrix. Chitosan has been extensively used in tissue engineering because of its superior properties, including biological compatibility, biodegradability, bioadsorption, inherent antimicrobial feature. Previously studies have shown that chitosan-based scaffolds can increase the proliferation of chondrocyte [Citation56]. For this purpose, Latifi et al imitated the structure of collagen fibers in human soft tissue and prepared chitosan-based biomimetic hydrogels with collagen fiber semi-interpenetrating network. They fabricated chitosan-based hydrogels modified with ethylenediamine tetraacetic acid (EDTA) and showed viscoelasticity in extrusion-based 3D bioprinting. They also revealed the proliferative activity and expression level of cartilage markers were significant enhanced. This kind of biomimetic chitosan-based hydrogel can not only maintain high cell survival rates, metabolic activity and migration ability, but also shown outstanding mechanical properties, thus its comprehensive characterizes can meet the basic practical requirements of tissue engineering. In addition, as chitosan being only natural cationic polysaccharide showed antibacterial properties [Citation57]. A kind of hydrogel material with antibacterial, antioxidant and electrical activity can be prepared by using the unique antibacterial properties of chitosan.
2.4. Hyaluronic acid hydrogel
The hyaluronic acid (HA), a major non-sulfated anionic hydrophilic glycosaminoglycan (GAG) that exists in human articular cartilage and provides lubrication. Hyaluronic is a linear polysaccharide composed of disaccharide units of glucuronic acid and N-acetyl glucosamine. HA interacts with cartilage surfaces through surface receptors such as CD44 and RHAMM, and induces chondrogenesis by promoting the expression of chondrogenesis-related genes (aggrecan, Sox9 and type II collagen) and depositing cartilage-specific ECM components [Citation58]. Excellent characteristics, such as high viscoelasticity, biocompatibility, non-immunogenicity and promoting cartilage differentiation, which making HA a suitable scaffold for cell packaging in bone and cartilage tissue engineering. Although HA has good biological features, it lacks the mechanical and viscoelastic properties required for 3D bio-printing, thus often needs to be modified to improve these limitations. Therefore, synthetic polymers are usually grafted onto HA to provide the necessary stability or combined with natural polysaccharides to form copolymer hydrogels for cartilage tissue engineering. These include chitosan-hyaluronic acid hydrogel, chitosan-hyaluronic acid biomimetic matrix, hyaluronic acid-agarose hydrogel and fibrin-hyaluronic acid hydrogel [Citation59,Citation60]. HA- polylactic acid (PLA) co-printing composite hydrogel can increase the expression of cartilage gene markers and specific matrix deposition, thus promoting tissue regeneration [Citation30]. Antich et al have successfully developed hyaluronic acid-based bioink containing alginate to obtain biological ink with appropriate printability, gel ability, hardness and degradability, in order to use 3D bioprinting to manufacture structures. In addition, 3D bioprinting this scafflod can promote cartilage formation in vitro, showing its potential in cartilage tissue engineering [Citation30].
2.5. Cellulose-based hydrogels
Cellulose is a linear natural polymer connected by glucose, which widely exists in plants, bacteria and other organisms. It is the most widely distributed natural polysaccharide polymer material in nature [Citation61]. It has excellent properties such as natural low toxicity, easy processing, biodegradability, regeneration and so on. Therefore, cellulose has been attracted much attention in the field of tissue engineering. Due to the existence of a large number of hydroxyl groups in the cellulose molecular chain, it is easy to form intermolecular hydrogen bonds, so that cellulose has excellent chemical stability and mechanical properties, which can meet the biomechanical requirements of human tissue. Therefore, it is very suitable to be used as a scaffold material in cartilage tissue engineering. Yang et al. used soluble cellulose derivatives to prepare cellulose-based injectable hydrogels with high self-repair ability and dual environmental response [Citation62]. Zhao et al. prepared hydrogels with semi-interpenetrating network structure using hydroxyethyl cellulose, lignosulfonate and other raw materials [Citation63]. They first used chemical crosslinking to polymerize acrylic acid and lignosulfonate, and then used a large number of hydroxyl groups in hydroxyethyl cellulose to carry out intermolecular hydrogen bond cross-linking, and finally formed a semi-interpenetrating network of hydrogel. This kind of hydrogel has outstanding mechanical properties and can meet the requirements of mechanical properties of load-bearing tissue of organisms.
2.6. Carrageenan-based hydrogels
Carrageenan is a sulfated polysaccharide extracted from red algae, which contains repeated disaccharide units, namely 4-β-D-galactopyranose (G-unit) and 4-α-D-galactopyranose (D-unit) or 4-linked 3-dehydrated galactose (DA unit). According to the number and position of sulfate groups in the repeated galactose unit, it can be divided into three main families-kappa (κ), iota (κ) and lambda (λ). Among them, κ-carrageenan (k-CRG) has unique properties, including thermal response, easy gelation, plasticity, similarity to glycosaminoglycan (GAG) and good injectability under physiological conditions. Some researchers have encapsulated stem cells in κ-CRG hydrogel under mild conditions, which showed ionic gel mechanism. Studies have shown that carrageenan-based hydrogels have good properties when used to encapsulate cells and transforming growth factor-β1 (TGF-β1) [Citation64]. It is reported that injectable κ-CRG hydrogel coated with human ASCs and TGF-β1 for cartilage regeneration can enhance cell viability and proliferation, increase chondrocyte differentiation and expression, and stimulate the production of cartilage proteoglycan and other ECM components [Citation65]. Carrageenan is also compounded with other polymers such as sodium alginate and chitosan to prepare hydrogel beads and fibers. It shows good processability in different formulations and can be used for tissue regeneration and cell transport [Citation66]. Recently, a bioprinted copolymer hydrogel composed of carrageenan and alginate was coated with MSCs, showing excellent structural strength and biological activity [Citation67]. κ-carrageenan hydrogels are temperature-sensitive and reversible volume transitions under thermal stimulation, so they are suitable for temperature-controllable delivery systems [Citation68].
2.7. Polysaccharide-protein hydrogel
However, the modification of polysaccharides is often time-consuming and requires a lot of chemical reactions. In order to improve the physiological signal and mechanical strength of the scaffold, cross-linking protein-polysaccharide has been developed in various engineering fields. This combination, termed proteoglycans, represents an attractive strategy for scaffold formation in tissue engineering because it mimics the structure of ECM and guides cell growth, proliferation, and phenotypic preservation [Citation69]. In addition, protein sugars are more stable and bioactive than their parent polymers. Therefore, the combination of hybrid polysaccharide hydrogel and protein provides a new perspective for the development of suitable scaffolds and their application as tissue engineering bioink in 3D bioprinting. shows a schematic diagram of the preparation and use of protein sugars, including their sources and the preparation of hydrogels for 3D printing in tissue engineering. In addition, polysaccharides are interesting biomaterials because they can combine to form composite polysaccharide hydrogels, called polysaccharide mixtures, such as alginate-nanocellulose, alginate-methylcellulose (MC), HA- agarose, etc. illustrates the characterization of natural and synthetic biomaterials-based bioinks for cartilage tissue engineering applications.
3. Applications of 3D-printing polysaccharide hydrogels in cartilage tissue engineering
3D printing technology can print artificial cartilage structures with appropriate mechanical properties, shape and size. In general, hydrogel based 3D printing can be divided into three main methods: laser-based, extrusion-based and droplet-based systems. During processing, the hydrogel is transferred from the liquid storage tank to the injection system and then extruded to the collection platform in a controlled manner. Extrusion and inkjet based bio-rinters deposit materials through layer-by-layer, while laser-assisted systems are based on photopolymerization of predeposited materials in specific predefined modes [Citation70]. Using 3D bio-printing technology to construct scaffolds requires a specific set of bioink properties to create a suitable 3D bio-printed structure to replace damaged bone and cartilage tissue.In the past few years, the application of using 3D printed engineering scaffolds for tissue repair has increased. Although 3D bioprinted scaffolds can make of a variety of materials, biodegradation behaviors are still the gold standard. Polysaccharide-based hydrogel has the incomparable biocompatibility and biodegradability over synthetic hydrogel, so it is very suitable to be used as a bio-ink for 3D bioprinting [Citation71]. In addition, it can also be functionalized into hydrogel composites so that it can improve the properties tailored to specific applications. In vitro and in vivo studies have shown that polysaccharide hydrogel provides a very ideal 3D environment for cartilage tissue regeneration. The mechanical properties of scaffolds used in cartilage tissue engineering (CTE) are critical because they are similar to those of articular cartilage and simulate conditions in the natural environment [Citation72]. In general, the mechanical properties of artificial structures should be align to the desired tissue: for soft tissue, a strength of 0.4 to 350 Mpa is necessary, while for hard tissue regeneration, a strength of 10–1,500 MPa is required [Citation73]. In contrast to synthetic hydrogels, natural polysaccharide-based injectable hydrogels are inadequate in mechanical strength and controllability. At present, the compression Young’s modulus of natural polysaccharide-based stents used in CTE is between 10 and 250 kPa, corresponding to the relatively low reported range of natural knee cartilage [Citation22]. Therefore, it cannot meet the standards of the use of load-bearing parts such as cartilage tissue. Aiming at the toughening strategy of hydrogel materials, Fu et al successfully constructed a differential double network structure of molecular chain entanglement and interpenetration (double network, DN) by using calcium ion crosslinked alginate network and vinyl modified phosphor (YAG: Ce-VTES) crosslinked polyacrylamide (PAAm) network, which significantly improved the mechanical properties of hydrogel [Citation74]. The alginate/polyacrylamide double network hydrogel shows exceptional extensibility and toughness, with elongation at break as high as 600% and compressive strength as high as 3.6 MPa.
In order to prepare polysaccharide-based high strength injectable hydrogels, You et al combined quaternized cellulose with cationic cellulose nanocrystals to prepare nano-composite hydrogels, which successfully improved the mechanical properties of hydrogels by two orders of magnitude. In vitro and animal studies showed this hydrogel had excellent biocompatibility [Citation75]. Pérez-Madrigal et al. selected two kinds of natural polysaccharide-based polymers-hyaluronic acid and alginic acid, and successfully prepared injectable hydrogels with excellent mechanical strength and sufficient stiffness by using thiol-yne click chemistry reaction [Citation76]. Due to the existence of double polysaccharide network, this kind of hydrogel has good biocompatibility and long-term mechanical strength stability, so it has great potential to become a 3D scaffold for tissue engineering to support and promote cartilage tissue regeneration. To improve the cell proliferation, print adaptability and produce suitable cartilage extracellular matrix, Olate Moya et al, photocrosslinking modified alginate was biologically combined with chondroitin sulfate and gelatin [Citation77]. All togethor, these polysaccharides imitate the ECM composition of natural articular hyaline cartilage and have been widely used in 3D printing of articular hyaline cartilage. However, when the new tissue match with natural cartilage, it is very important to promote the regeneration of cartilage defects. Thus, an appropriate cell type for 3D printing is very important for the regeneration of functional cartilage. And these materials should provide a suitable microenvironment for cell differentiation and maturation, and promote the production of collagen and glycosaminoglycan in the graft. Chondrocytes are only resident cells in articular cartilage and play a vital role in the production and maintenance of cartilage matrix. However, due to the limited self-renewal ability and number of autologous chondrocytes, the regeneration of large cartilage defects needs stem cells especially mesenchymal stem cells (MSCs) to improve the ability to repair damaged cartilage tissue [Citation78]. Integrating MSCs into 3D printing scaffolds can guide these cells to differentiate into chondrocytes and support the formation of similar natural articular cartilage [Citation79,Citation80]. Similarly, inducing pluripotent stem cells (IPSC) also hold eligible potential for chondrogenesis. Hence, Nakamura et al. integrated iPSC into 3D printing scaffolds and shown effectively differentiated into cartilage-like structure [Citation81]. In addition, researchers not only take into account the above properties in the construction of scaffold materials, but also introduce extracellular matrix and other growth factors to simulate the physiological microenvironment of the original tissue, thus can induce seed cells to fabricate hyaline cartilage.
4. Applications of 3D-printing polysaccharide hydrogels in osteochondral tissue engineering
Osteochondral tissue consists of two distinct characteristic tissues: cartilage and subchondral bone, involving articular cartilage, calcified cartilage and subchondral bone, which have different structural, mechanical, biochemical and metabolic properties. Typically, the changes of its structure or composition usually lead to the destruction of whole joint integrity and the ultimate loss of function. At present, the main clinical treatment of osteochondral defects is osteochondral autotransplantation. In this method, the bone blocks taken from the less weight-bearing areas of the femur are used as bone plugs to repair osteochondral defects. But the treatment also has some drawbacks, such as increased incidence of donor sites and expensive surgeries. The use of 3D printing technology to produce physical or mechanical gradients, as well as the pattern of stimulating growth factors, may help to improve the functional recovery of subchondral bone tissue. For example, the cartilage layer is made of hydrogels or polymer scaffolds with different porosity. For the selection of bone layer materials, a biodegradable thermoplastic polymer is often exploited. For the interface between cartilage layer and bone layer, covalent chemical bond coupling and adhesive bonding are usually used to fix the two layers. Therefore, it may be a promising strategy to create a scaffold with gradient porosity to simulate the holes in each layer of bone and cartilage. Scaffolds with vertical porosity and pore diameter gradient were fabricated by extruding 3D printing system, and it was proved that scaffolds with diameters of 90-120um contributed to cartilage formation of MSCs, while larger diameters with about 300 um always promote osteogenesis [Citation82]. MSCs have the capacity of multi-directional differentiation, including chondrocytes, osteocytes and osteoblasts, so it holds great prospective for osteochondral tissue regeneration [Citation78]. Through the recruitment of endogenous MSCs, the delivery of prochondral factor Kartogenin (KGN) in the upper layer promotes cartilage differentiation, while the introduction of pro-osteogenesis aptamers in the lower layer promotes osteogenic differentiation, showing a significant acceleration in the repair of osteochondral defects in the rat model [Citation83]. In addition to recruiting MSCs, xenogeneic MSCs can also produce double-layer bone and cartilage results under the action of inducing factors. MSCs were implanted into 3D printed multi-layer structures containing a variety of extracellular matrix biomaterials for heterogeneous osteochondral regeneration. Hyaluronic acid and transforming growth factor-β3 were combined to promote the formation of cartilage area, while collagen combined with bone morphogenetic protein-2 to form bone region [Citation84].
More recently, a complete double-layer silk fibroin scaffold was constructed, which was composed of a dense and smooth biomimetic cartilage layer and a porous layer loaded with BMP-2, then filled and sealed with Sil-MA hydrogel loaded with TGF-β 3 (TGF- β 3/Sil-MA) for bone and cartilage repair [Citation85]. The porous network containing BMP-2 is beneficial to the transport of nutrients, provides excellent mechanical properties for the newly formed tissue, and can stimulate the osteogenic differentiation of BMSCs.TGF-β3/Sil-MA provides a bridge between the cartilage layer of the scaffold and the surrounding cartilage, guiding the new cartilage to grow to the surrounding natural cartilage and replacing the degraded cartilage layer in the early stage of knee joint repair. This composite material can repair bone and cartilage through endogenous cells and is a potential scaffold for osteochondral repair () [Citation85]. In conclusion, the construction of a highly specialized bilayer scaffolds to simulate the formation of osteochondral and subchondral bone units provides a broad prospect for integrated reconstruction, so it is worthy of further preclinical evaluation.
Figure 4. (a) schematic of the synthesis process of Sil-MA hydrogel. (b) osteochondral defect repair in rabbits with integral bilayer silk scaffold were evaluated in the regenerated area and by safranin-O/fast-green staining. (c)the ICRS scores and micro CT were used to evaluate the BMD (g/cm3), BV/TV (%), and th (μm) values of bone remodeling. Reproduced with permission from ref [Citation85]. Copyright © 2023 elsevier B.V.
![Figure 4. (a) schematic of the synthesis process of Sil-MA hydrogel. (b) osteochondral defect repair in rabbits with integral bilayer silk scaffold were evaluated in the regenerated area and by safranin-O/fast-green staining. (c)the ICRS scores and micro CT were used to evaluate the BMD (g/cm3), BV/TV (%), and th (μm) values of bone remodeling. Reproduced with permission from ref [Citation85]. Copyright © 2023 elsevier B.V.](/cms/asset/47545045-44b3-4acd-95e5-bbd32a895694/tdmp_a_2284482_f0004_oc.jpg)
5. Conclusions and perspectives
With the deepening of social aging and the progress of sports trauma, bone and cartilage injury is a very common clinical disease. Although a variety of treatments have been clinically initiated, such as microfractures, autologous osteochondral grafts, allogeneic cartilage grafts and autologous chondrocyte grafts, there are still certain drawbacks and limitations. Tissue engineering techniques implanted in the body in a minimally invasive manner, which can effectively reduce patient pain and the risk of surgical joint replacement. Due to its high biocompatibility, biodegradability and structural modifiability, the application of polysaccharide-based injectable hydrogels in tissue engineering has gained widespread attention, and a large number of research results have been support this direction.
In addition, engineered exosomes or exosome analogs can also be added as bioinks to polysaccharide-based hydrogels, which are beneficial for enhancing the repair of cartilage tissue [Citation86–94] At the same time, there are still some problems to be solved in the application of polysaccharide-based hydrogels in the field of tissue engineering: (1) realizing many functions of natural tissue is an important goal of tissue engineering materials. At present, the single-component polysaccharide hydrogel still cannot have the complete functions of natural tissue in many aspects, such as biological response, biomechanics, and material transport and so on, so it is limited in practical application. Researchers try to use other chemical synthetic materials and polysaccharide-based hydrogels to make bioactive materials, which expands new ideas in this field, and is expected to promote the development of medical functions of polysaccharide-based injectable hydrogels. (2) the research on the functional mechanism of bone and joint natural tissue is still insufficient, so it is difficult to determine and imitate the core elements of functional realization, which fundamentally limits the development of biological function of bone and cartilage implant materials. In the future, the preparation of polysaccharide-based hydrogel can be combined with the study of joint histology. Based on the principles of biology, physics and even chemistry, the structure and physicochemical properties of biomimetic materials are designed to simulate high strength, elasticity and damping function. In order to expand its clinical application in bone and cartilage tissue engineering, the 3D biological printing technology of hydrogel has attracted much attention in the field of bone and cartilage tissue engineering. However, there are technical challenges, including the selection of appropriate biomaterials, cell types, and bioactive molecules for the manufacture of biological ink to promote cell growth and differentiation after transplantation. The main problem of 3D printing of living cell hydrogel is to optimize printing parameters in order to achieve excellent microenvironment to promote cell viability and phenotype. The latest progress shows that scaffold materials can be externally stimulated by external stimuli such as temperature, pH, light, and deformable structures under electrophoresis called dynamic 3D structures or 4D printing [Citation95]. The application of 4D printing technology to prepare scaffolds is one of the development directions in the future. This technique can improve the accuracy and resolution of scaffolds, because 4D printing can not only form scaffolds directly by feeling external stimuli, but also control the time of multi-scale structural deformation in vivo bone and cartilage repair after implantation. Shrink the 3D print bracket after processing. The spontaneous deformation of 4D printing fascinates many researchers.
You et al uses 4D printing technology to fabricate a multi-response bilayer deformable film consisting of a shape memory polymer (SMP) layer and a hydrogel layer. In vivo experiments showed that compared with the reference membrane with static microstructure, the 4D deformable membrane showed more than 30% improvement in new bone formation [Citation96]. More importantly, 4D membranes can conformal and wrap the bone defect model in a non-invasive way, and this strategy can be extended to the repair of complex tissue defects. If necessary, the controlled release of biodegradability and biochemical clues can be integrated into the scaffold. With the latest developments in switching power supplies, more complex topology operations such as reversible or multi-step sequential switches can also be combined. The use of 4D printing strategy provides a multi-functional platform for accurate and personalized treatment.
Although tremendous progress has been made in this area, it remains a challenge to effectively translate research into clinical practice. The ultimate goal is to translate a promising therapeutic strategy for the regeneration and repair of osteochondral and full-thickness articular cartilage damage in patients.
Authors contributions
Conceptualization, Y.J.L.; writing – original draft preparation, H.J.H., and L.J.Y.; funding acquisition, Y.L.; constructive discussions, Z.J.H, J.Y.X., J.X., and W.Y.; All authors have read and agreed to the submitted version of the manuscript.
Disclosure statement
No potential conflict of interest was reported by the author(s).
Additional information
Funding
References
- Kon E, Delcogliano M, Filardo G, et al. Novel nano-composite multilayered biomaterial for osteochondral regeneration: a pilot clinical trial. Am J Sports Med. 2011;39(6):1180–1190. doi: 10.1177/0363546510392711
- Gomoll AH, Madry H, Knutsen G, et al. The subchondral bone in articular cartilage repair: current problems in the surgical management. Knee Surg Sports Traumatol Arthrosc. 2010;18(4):434–447. doi: 10.1007/s00167-010-1072-x
- Huey DJ, Hu JC, Athanasiou KA. Unlike bone, cartilage regeneration remains elusive. Science. 2012;338(6109):917–921. doi: 10.1126/science.1222454
- Akter F, Ibanez J. Chapter 8 - bone and cartilage tissue engineering. In: Akter F, editor. Tissue engineering made easy. Academic Press; 2016. pp. 77–97. doi: 10.1016/B978-0-12-805361-4.00008-4.
- Ando W, Tateishi K, Hart DA, et al. Cartilage repair using an in vitro generated scaffold-free tissue-engineered construct derived from porcine synovial mesenchymal stem cells. Biomaterials. 2007;28(36):5462–5470. doi: 10.1016/j.biomaterials.2007.08.030
- Lemoine M, Casey SM, O’Byrne JM, et al. The development of natural polymer scaffold-based therapeutics for osteochondral repair. Biochem Soc Trans. 2020;48(4):1433–1445. doi: 10.1042/BST20190938
- Wei W, Ma Y, Yao X, et al. Advanced hydrogels for the repair of cartilage defects and regeneration. Bioact Mater. 2021;6(4):998–1011. doi: 10.1016/j.bioactmat.2020.09.030
- Yilmaz B, Al Rashid A, Mou YA, et al. Bioprinting: a review of processes, materials and applications. Bioprinting. 2021;23:e00148. doi: 10.1016/j.bprint.2021.e00148
- Xie JH, Jin ML, Morris GA, et al. Advances on bioactive polysaccharides from medicinal plants. Crit Rev Food Sci Nutr. 2016;56(Suppl 1):S60–84. doi: 10.1080/10408398.2015.1069255
- Shi L. Bioactivities, isolation and purification methods of polysaccharides from natural products: a review. Int j biol macromol. 2016;92:37–48. doi: 10.1016/j.ijbiomac.2016.06.100
- Zhu T, Mao J, Cheng Y, et al. Recent progress of polysaccharide-based hydrogel interfaces for wound healing and tissue engineering. Adv Mater Interfaces. 2019;6(17):1900761. doi: 10.1002/admi.201900761
- Duarte Campos DF, Blaeser A, Weber M, et al. Three-dimensional printing of stem cell-laden hydrogels submerged in a hydrophobic high-density fluid. Biofabrication. 2013;5(1):015003. doi: 10.1088/1758-5082/5/1/015003
- Sadeghianmaryan A, Naghieh S, Alizadeh Sardroud H, et al. Extrusion-based printing of chitosan scaffolds and their in vitro characterization for cartilage tissue engineering. Int j biol macromol. 2020;164:3179–3192. doi: 10.1016/j.ijbiomac.2020.08.180
- Ye K, Felimban R, Traianedes K, et al. Chondrogenesis of infrapatellar fat pad derived adipose stem cells in 3D printed chitosan scaffold. PLoS One. 2014;9(6):e99410. doi: 10.1371/journal.pone.0099410
- He Y, Derakhshanfar S, Zhong W, et al. Characterization and application of carboxymethyl chitosan-based bioink in cartilage tissue engineering. J Nanomater. 2020;2020:2057097. doi: 10.1155/2020/2057097
- Pescosolido L, Schuurman W, Malda J, et al. Hyaluronic acid and dextran-based semi-IPN hydrogels as biomaterials for bioprinting. Biomacromolecules. 2011;12(5):1831–1838. doi: 10.1021/bm200178w
- Daly AC, Critchley SE, Rencsok EM, et al. A comparison of different bioinks for 3D bioprinting of fibrocartilage and hyaline cartilage. Biofabrication. 2016;8(4):045002. doi: 10.1088/1758-5090/8/4/045002
- Levato R, Webb WR, Otto IA, et al. The bio in the ink: cartilage regeneration with bioprintable hydrogels and articular cartilage-derived progenitor cells. Acta Biomaterialia. 2017;61:41–53. doi: 10.1016/j.actbio.2017.08.005
- Kesti M, Müller M, Becher J, et al. A versatile bioink for three-dimensional printing of cellular scaffolds based on thermally and photo-triggered tandem gelation. Acta Biomaterialia. 2015;11:162–172. doi: 10.1016/j.actbio.2014.09.033
- Park JY, Choi JC, Shim JH, et al. A comparative study on collagen type I and hyaluronic acid dependent cell behavior for osteochondral tissue bioprinting. Biofabrication. 2014;6(3):035004. doi: 10.1088/1758-5082/6/3/035004
- Schuurman W, Levett PA, Pot MW, et al. Gelatin-methacrylamide hydrogels as potential biomaterials for fabrication of tissue-engineered cartilage constructs. Macromol biosci. 2013;13(5):551–561. doi: 10.1002/mabi.201200471
- López-Marcial GR, Zeng AY, Osuna C, et al. O’Connell GD: agarose-based hydrogels as suitable bioprinting materials for tissue engineering. ACS Biomater Sci Eng. 2018;4(10):3610–3616. doi: 10.1021/acsbiomaterials.8b00903
- Duarte Campos DF, Blaeser A, Korsten A, et al. The stiffness and structure of three-dimensional printed hydrogels direct the differentiation of mesenchymal stromal cells toward adipogenic and osteogenic lineages. Tissue Eng Part A. 2015;21(3–4):740–756. doi: 10.1089/ten.tea.2014.0231
- Henrionnet C, Pourchet L, Neybecker P, et al. Combining innovative bioink and low cell density for the production of 3D-Bioprinted cartilage substitutes: a pilot study. Stem Cells Int. 2020;2020:2487072. doi: 10.1155/2020/2487072
- Park S-H, Song T, Bae TS, et al. Synergistic effects of alginate coating method on cartilage tissue engineering using fibrin/ha composite gel. Int J Precis Eng Manuf. 2012;13(11):2067–2074. doi: 10.1007/s12541-012-0272-3
- Müller M, Öztürk E, Arlov Ø, et al. Alginate Sulfate-Nanocellulose Bioinks for Cartilage Bioprinting Applications. Ann Biomed Eng. 2017;45(1):210–223. doi: 10.1007/s10439-016-1704-5
- Markstedt K, Mantas A, Tournier I, et al. 3D bioprinting human chondrocytes with Nanocellulose-Alginate Bioink for cartilage tissue engineering applications. Biomacromolecules. 2015;16(5):1489–1496. doi: 10.1021/acs.biomac.5b00188
- Huang J, Huang Z, Liang Y, et al. 3D printed gelatin/hydroxyapatite scaffolds for stem cell chondrogenic differentiation and articular cartilage repair. Biomater Sci. 2021;9(7):2620–2630. doi: 10.1039/D0BM02103B
- Fan Y, Shi T, Yue X, et al. 3D Composite Cell Printing Gelatin/Sodium Alginate/n-HAP Bioscaffold. J Phys. 2019;1213(4):042020. doi: 10.1088/1742-6596/1213/4/042020
- Antich C, de Vicente J, Jiménez G, et al. Bio-inspired hydrogel composed of hyaluronic acid and alginate as a potential bioink for 3D bioprinting of articular cartilage engineering constructs. Acta Biomaterialia. 2020;106:114–123. doi: 10.1016/j.actbio.2020.01.046
- Hodder E, Duin S, Kilian D, et al. Investigating the effect of sterilisation methods on the physical properties and cytocompatibility of methyl cellulose used in combination with alginate for 3D-bioplotting of chondrocytes. J Mater Sci. 2019;30(1):10. doi: 10.1007/s10856-018-6211-9
- Nguyen D, Hägg DA, Forsman A, et al. Cartilage tissue engineering by the 3D bioprinting of iPS Cells in a Nanocellulose/alginate bioink. Sci Rep. 2017;7(1):658. doi: 10.1038/s41598-017-00690-y
- Ahmad Raus R, Wan Nawawi WMF, Nasaruddin RR. Alginate and alginate composites for biomedical applications. Asian J Pharm Sci. 2021;16(3):280–306. doi: 10.1016/j.ajps.2020.10.001
- Trica B, Delattre C, Gros F, et al. Extraction and characterization of alginate from an edible brown seaweed (cystoseira barbata) harvested in the Romanian black sea. Mar Drugs. 2019;17(7):405. doi: 10.3390/md17070405
- Urtuvia V, Maturana N, Acevedo F, et al. Bacterial alginate production: an overview of its biosynthesis and potential industrial production. World J Microbiol Biotechnol. 2017;33(11):198. doi: 10.1007/s11274-017-2363-x
- Dudun AA, Akoulina EA, Zhuikov VA, et al. Competitive biosynthesis of bacterial alginate using Azotobacter vinelandii 12 for tissue engineering applications. Polymers. 2021;14(1). doi: 10.3390/polym14010131
- Yan J, Miao Y, Tan H, et al. Injectable alginate/hydroxyapatite gel scaffold combined with gelatin microspheres for drug delivery and bone tissue engineering. Mater Sci Eng C. 2016;63:274–284. doi: 10.1016/j.msec.2016.02.071
- Gonzalez-Fernandez T, Tenorio AJ, Campbell KT, et al. Alginate-based bioinks for 3D bioprinting and fabrication of anatomically accurate bone grafts. Tissue Eng Part A. 2021;27(17–18):1168–1181. doi: 10.1089/ten.tea.2020.0305
- Choe G, Oh S, Seok JM, et al. Graphene oxide/alginate composites as novel bioinks for three-dimensional mesenchymal stem cell printing and bone regeneration applications. Nanoscale. 2019;11(48):23275–23285. doi: 10.1039/C9NR07643C
- De Santis MM, Alsafadi HN, Tas S, et al. Extracellular-Matrix-Reinforced Bioinks for 3D Bioprinting Human Tissue. Adv Mater (Deerfield Beach, Fla). 2021;33(3):e2005476. doi: 10.1002/adma.202005476
- Kang ES, Kim H, Han Y, et al. Enhancing osteogenesis of adipose-derived mesenchymal stem cells using gold nanostructure/peptide-nanopatterned graphene oxide. Colloids Surf B Biointerfaces. 2021;204:111807. doi: 10.1016/j.colsurfb.2021.111807
- Zhang J, Wehrle E, Adamek P, et al. Optimization of mechanical stiffness and cell density of 3D bioprinted cell-laden scaffolds improves extracellular matrix mineralization and cellular organization for bone tissue engineering. Acta Biomaterialia. 2020;114:307–322. doi: 10.1016/j.actbio.2020.07.016
- Gomez CG, Rinaudo M, Villar MA. Oxidation of sodium alginate and characterization of the oxidized derivatives. Carbohydr Polym. 2007;67(3):296–304. doi: 10.1016/j.carbpol.2006.05.025
- Wei Z, Yang JH, Liu ZQ, et al. Novel biocompatible polysaccharide-based self-healing hydrogel. Adv Funct Mater. 2015;25(9):1352–1359. doi: 10.1002/adfm.201401502
- Balakrishnan B, Jayakrishnan A. Self-cross-linking biopolymers as injectable in situ forming biodegradable scaffolds. Biomaterials. 2005;26(18):3941–3951. doi: 10.1016/j.biomaterials.2004.10.005
- Distler T, Solisito AA, Schneidereit D, et al. 3D printed oxidized alginate-gelatin bioink provides guidance for C2C12 muscle precursor cell orientation and differentiation via shear stress during bioprinting. Biofabrication. 2020;12(4):045005. doi: 10.1088/1758-5090/ab98e4
- Tabata M, Shimoda T, Sugihara K, et al. Osteoconductive and hemostatic properties of apatite formed on/in agarose gel as a bone-grafting material. J Biomed Mater Res, Part B. 2003;67(2):680–688. doi: 10.1002/jbm.b.10063
- Zarrintaj P, Manouchehri S, Ahmadi Z, et al. Agarose-based biomaterials for tissue engineering. Carbohydr Polym. 2018;187:66–84. doi: 10.1016/j.carbpol.2018.01.060
- López-Marcial GR, Elango K, O’Connell GD. O’Connell GD: addition of collagen type I in agarose created a dose-dependent effect on matrix production in engineered cartilage. Regen Biomater. 2022;9:rbac048. doi: 10.1093/rb/rbac048
- Yang J, Zhang YS, Yue K, et al. Cell-laden hydrogels for osteochondral and cartilage tissue engineering. Acta Biomaterialia. 2017;57:1–25. doi: 10.1016/j.actbio.2017.01.036
- Kreimendahl F, Köpf M, Thiebes AL, et al. Three-dimensional printing and angiogenesis: tailored agarose-type I collagen blends comprise three-dimensional printability and angiogenesis potential for tissue-engineered substitutes. Tissue Eng Part C Methods. 2017;23(10):604–615. doi: 10.1089/ten.tec.2017.0234
- Duarte Campos DF, Drescher W, Rath B, et al. Supporting biomaterials for articular cartilage repair. Cartilage. 2012;3(3):205–221. doi: 10.1177/1947603512444722
- Singh YP, Bhardwaj N, Mandal BB. Potential of agarose/silk fibroin blended hydrogel for in vitro cartilage tissue engineering. ACS Appl Mater Inter. 2016;8(33):21236–21249. doi: 10.1021/acsami.6b08285
- Melke J, Midha S, Ghosh S, et al. Silk fibroin as biomaterial for bone tissue engineering. Acta Biomaterialia. 2016;31:1–16. doi: 10.1016/j.actbio.2015.09.005
- García-Martínez L, Campos F, Godoy-Guzmán C, et al. Encapsulation of human elastic cartilage-derived chondrocytes in nanostructured fibrin-agarose hydrogels. Histochem Cell Biol. 2017;147(1):83–95. doi: 10.1007/s00418-016-1485-9
- Wu H, Wan Y, Cao X, et al. Proliferation of chondrocytes on porous poly(dl-lactide)/chitosan scaffolds. Acta Biomaterialia. 2008;4(1):76–87. doi: 10.1016/j.actbio.2007.06.010
- Pranantyo D, Xu LQ, Kang ET, et al. Chitosan-based peptidopolysaccharides as cationic antimicrobial agents and antibacterial coatings. Biomacromolecules. 2018;19(6):2156–2165. doi: 10.1021/acs.biomac.8b00270
- Bajorath J, Greenfield B, Munro SB, et al. Identification of CD44 residues important for hyaluronan binding and delineation of the binding site. J Biol Chem. 1998;273(1):338–343. doi: 10.1074/jbc.273.1.338
- Mohan N, Mohanan PV, Sabareeswaran A, et al. Chitosan-hyaluronic acid hydrogel for cartilage repair. Int J Biol Macromol. 2017;104(Pt B):1936–1945. doi: 10.1016/j.ijbiomac.2017.03.142
- Rampichová M, Filová E, Varga F, et al. Fibrin/hyaluronic acid composite hydrogels as appropriate scaffolds for in vivo artificial cartilage implantation. ASAIO J. 2010;56(6):563–568. doi: 10.1097/MAT.0b013e3181fcbe24
- Li T, Chen C, Brozena AH, et al. Developing fibrillated cellulose as a sustainable technological material. Nature. 2021;590(7844):47–56. doi: 10.1038/s41586-020-03167-7
- Yang X, Bakaic E, Hoare T, et al. Injectable polysaccharide hydrogels reinforced with cellulose nanocrystals: morphology, rheology, degradation, and cytotoxicity. Biomacromolecules. 2013;14(12):4447–4455. doi: 10.1021/bm401364z
- Zhao J, Zheng K, Nan J, et al. Synthesis and characterization of lignosulfonate-graft-poly (acrylic acid)/hydroxyethyl cellulose semi-interpenetrating hydrogels. React Funct Polym. 2017;115:28–35. doi: 10.1016/j.reactfunctpolym.2017.03.019
- Rocha PM, Santo VE, Gomes ME, et al. Encapsulation of adipose-derived stem cells and transforming growth factor-β1 in carrageenan-based hydrogels for cartilage tissue engineering. J Bioact Compat Polym. 2011;26(5):493–507. doi: 10.1177/0883911511420700
- Dev A, Mohanbhai SJ, Kushwaha AC, et al. κ-carrageenan-C-phycocyanin based smart injectable hydrogels for accelerated wound recovery and real-time monitoring. Acta Biomaterialia. 2020;109:121–131. doi: 10.1016/j.actbio.2020.03.023
- Ali KA, Hassan ME, Elnashar MMM. Development of functionalized carrageenan, chitosan and alginate as polymeric chelating ligands for water softening. Int J Environ Sci Technol. 2017;14(9):2009–2014. doi: 10.1007/s13762-017-1298-y
- Kim UJ, Park J, Li C, et al. Structure and properties of silk hydrogels. Biomacromolecules. 2004;5(3):786–792. doi: 10.1021/bm0345460
- Gholamipour-Shirazi A, Norton IT, Mills T. Dual stimuli-sensitive carrageenan-based formulation for additive manufacturing. Int J Biol macromol. 2021;189:370–379. doi: 10.1016/j.ijbiomac.2021.08.127
- Cassimjee H, Kumar P, Choonara YE, et al. Proteosaccharide combinations for tissue engineering applications. Carbohydr Polym. 2020;235:115932. doi: 10.1016/j.carbpol.2020.115932
- Sabzevari A, Rayat Pisheh H, Ansari M, et al. Progress in bioprinting technology for tissue regeneration. J Artif Organs. 2023;26(4):255–274. doi: 10.1007/s10047-023-01394-z
- Unagolla JM, Jayasuriya AC. Hydrogel-based 3D bioprinting: a comprehensive review on cell-laden hydrogels, bioink formulations, and future perspectives. Appl Mater Today. 2020;18:18. doi: 10.1016/j.apmt.2019.100479
- Hutmacher DW. Scaffolds in tissue engineering bone and cartilage. Biomaterials. 2000;21(24):2529–2543. doi: 10.1016/S0142-9612(00)00121-6
- Hollister SJ. Porous scaffold design for tissue engineering. Nature Mater. 2005;4(7):518–524. doi: 10.1038/nmat1421
- Fu J, In Het Panhuis M. In Het Panhuis M: Hydrogel properties and applications. J Mat Chem B. 2019;7(10):1523–1525. doi: 10.1039/C9TB90023C
- You J, Cao J, Zhao Y, et al. Improved Mechanical Properties and Sustained Release Behavior of Cationic Cellulose Nanocrystals Reinforeced Cationic Cellulose Injectable Hydrogels. Biomacromolecules. 2016;17(9):2839–2848. doi: 10.1021/acs.biomac.6b00646
- Pérez-Madrigal MM, Shaw JE, Arno MC, et al. Robust alginate/hyaluronic acid thiol-yne click-hydrogel scaffolds with superior mechanical performance and stability for load-bearing soft tissue engineering. Biomater Sci. 2020;8(1):405–412. doi: 10.1039/C9BM01494B
- Olate-Moya F, Arens L, Wilhelm M, et al. Chondroinductive alginate-based hydrogels having graphene oxide for 3D printed scaffold fabrication. ACS Appl Mater Inter. 2020;12(4):4343–4357. doi: 10.1021/acsami.9b22062
- Xu X, Xu L, Xia J, et al. Harnessing knee joint resident mesenchymal stem cells in cartilage tissue engineering. Acta Biomaterialia. 2023;168:372–387. doi: 10.1016/j.actbio.2023.07.024
- Huang J, Liu Q, Xia J, et al. Modification of mesenchymal stem cells for cartilage-targeted therapy. J Transl Med. 2022;20(1):515. doi: 10.1186/s12967-022-03726-8
- Jia Z, Liu Q, Liang Y, et al. Repair of articular cartilage defects with intra-articular injection of autologous rabbit synovial fluid-derived mesenchymal stem cells. J Transl Med. 2018;16(1):123. doi: 10.1186/s12967-018-1485-8
- Nakamura A, Murata D, Fujimoto R, et al. Bio-3D printing iPSC-derived human chondrocytes for articular cartilage regeneration. Biofabrication. 2021;13(4):044103. doi: 10.1088/1758-5090/ac1c99
- Karageorgiou V, Kaplan D. Porosity of 3D biomaterial scaffolds and osteogenesis. Biomaterials. 2005;26(27):5474–5491. doi: 10.1016/j.biomaterials.2005.02.002
- Hu X, Wang Y, Tan Y, et al. A difunctional regeneration scaffold for knee repair based on aptamer-directed cell recruitment. Adv Mater. 2017;29(15): doi: 10.1002/adma.201605235
- Shim JH, Jang KM, Hahn SK, et al. Three-dimensional bioprinting of multilayered constructs containing human mesenchymal stromal cells for osteochondral tissue regeneration in the rabbit knee joint. Biofabrication. 2016;8(1):014102. doi: 10.1088/1758-5090/8/1/014102
- Wu X, Zhou M, Jiang F, et al. Marginal sealing around integral bilayer scaffolds for repairing osteochondral defects based on photocurable silk hydrogels. Bioact Mater. 2021;6(11):3976–3986. doi: 10.1016/j.bioactmat.2021.04.005
- Xu X, Xu L, Wen C, et al. Programming assembly of biomimetic exosomes: an emerging theranostic nanomedicine platform. Mater Today Bio. 2023;22:100760. doi: 10.1016/j.mtbio.2023.100760
- Liang Y, Xu X, Li X, et al. Chondrocyte-targeted MicroRNA delivery by engineered exosomes toward a cell-free osteoarthritis therapy. ACS Appl Mater Inter. 2020;12(33):36938–36947. doi: 10.1021/acsami.0c10458
- Liang Y, Duan L, Lu J, et al. Engineering exosomes for targeted drug delivery. Theranostics. 2021;11(7):3183–3195. doi: 10.7150/thno.52570
- Duan L, Xu L, Xu X, et al. Exosome-mediated delivery of gene vectors for gene therapy. Nanoscale. 2021;13(3):1387–1397. doi: 10.1039/D0NR07622H
- Liu Q, Li D, Pan X, et al. Targeted therapy using engineered extracellular vesicles: principles and strategies for membrane modification. J Nanobiotechnol. 2023;21(1):334. doi: 10.1186/s12951-023-02081-0
- Iqbal Z, Rehman K, Xia J, et al. Biomaterial-assisted targeted and controlled delivery of CRISPR/Cas9 for precise gene editing. Biomater Sci. 2023;11(11):3762–3783. doi: 10.1039/D2BM01636B
- Liang Y, Iqbal Z, Wang J, et al. Cell-derived extracellular vesicles for CRISPR/Cas9 delivery: engineering strategies for cargo packaging and loading. Biomater Sci. 2022;10(15):4095–4106. doi: 10.1039/D2BM00480A
- Huang J, Xiong J, Yang L, et al. Cell-free exosome-laden scaffolds for tissue repair. Nanoscale. 2021;13(19):8740–8750. doi: 10.1039/D1NR01314A
- Xu L, Xu X, Liang Y, et al. Osteoclast-targeted delivery of anti-miRNA oligonucleotides by red blood cell extracellular vesicles. J Control Release. 2023;358:259–272. doi: 10.1016/j.jconrel.2023.04.043
- Anandhapadman A, Venkateswaran A, Jayaraman H, et al. Advances in 3D printing of composite scaffolds for the repairment of bone tissue associated defects. Biotechnol Prog. 2022;38(3):e3234. doi: 10.1002/btpr.3234
- You D, Chen G, Liu C, et al. 4D printing of multi-responsive membrane for accelerated in vivo bone healing via remote regulation of stem cell fate. Adv Funct Mater. 2021;31(40):2103920. doi: 10.1002/adfm.202103920