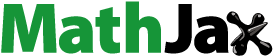
ABSTRACT
The stratification of the atmospheric surface layer (ASL) plays an important role in regulating the water vapor and heat exchange across the lake–air interface. Based on one year of data measured by the eddy covariance technique over Erhai Lake in 2015, the ASL stability () was divided into six ranges, including unstable (
), weakly unstable (
), near-neutral1 (
), near-neutral2 (
), weakly stable (
), and stable (
). The characteristics of ASL stability conditions and factors controlling the latent (
) and sensible heat (
) fluxes under different stability conditions were analyzed in this study. The stability conditions of Erhai Lake have noticeably seasonal and diurnal variation, with the near-neutral and (weakly) stable stratification usually occurring before July, with frequencies of 51.7% and 23.3%, respectively, but most of the (weakly) unstable stratification was observed after July, with a frequency of 59.8%. Large evaporation occurred even in stable atmospheric conditions, due to the coupled effects of the relatively larger lake–air vapor pressure difference and wind speed. The relative controls of
and
by different atmospheric variables are largely dependent on the stability conditions. In stable and unstable ranges,
is closely correlated with the vapor pressure difference, whereas in weakly unstable to weakly stable ranges, it is primarily controlled by wind speed.
is related to wind speed and the lake–air temperature difference under stable conditions, but shows no obvious relationship under unstable conditions.
Graphical abstract
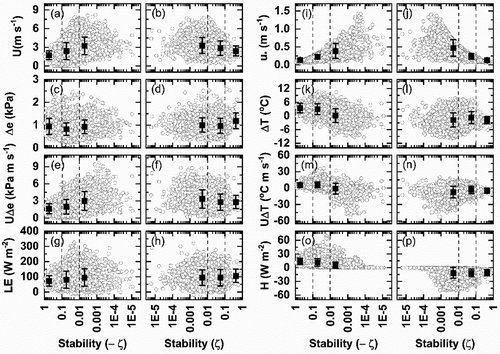
摘要
本文利用由涡动相关法观测得到的2015年高原开放淡水湖泊 (洱海) 的湍流通量资料, 分析了湖面近地层大气稳定度特征以及不同稳定度条件下潜热和感热通量的主要控制因子。湖面近地层大气稳定度具有显著的日变化和季节变化特征。不同大气变量对潜热和感热通量的相对影响程度受到大气稳定度的调控。在稳定和不稳定范围内, 潜热通量与湖-气间的水汽压差密切相关, 而在弱不稳定到弱稳定范围内, 潜热通量主要受风速控制。在稳定条件下, 风速和湖-气温差对感热通量具有显著影响, 而在不稳定条件下对感热通量的影响不显著。
1. Introduction
Lakes and reservoirs cover more than 4% of the global land surface area, and even more in the Northern Hemisphere (Downing et al. Citation2006), and play an important role in affecting the local and regional climate through turbulent exchange of heat and water vapor between the water and the overlying atmosphere (Rouse et al. Citation2005; Long et al. Citation2007). In recent times, the surface fluxes of latent () and sensible (
) heat and their controlling factors have attracted more attention on inland water bodies owing to the development of direct measurements by the eddy covariance (EC) technique (Blanken et al. Citation2000; Liu et al. Citation2009; Lee et al. Citation2014). However, the dynamics and drivers of surface exchange processes at the water–air interface vary on temporal and spatial scales, due to the difference in lake morphology, geographic condition, and weather and climatic background (Du et al. Citation2018a; Woolway et al. Citation2018). More understanding on the dynamics and physical control factors of turbulent exchange processes between the lake and the atmosphere under climate change is much needed, which will help improve the parameterization of lake–atmosphere interaction in numerical models (Dutra et al. Citation2010).
Many researchers have pointed out that wind speed and the vertical gradient of temperature and humidity are critical factors for diurnal variations of and
at the water–air interface (Liu et al. Citation2009; Nordbo et al. Citation2011; Shao et al. Citation2015). A positive temperature gradient (lake surface temperature exceeds air temperature) is typically related to unstable stratification in the atmospheric surface layer (ASL), which can promote heat and water vapor transfer from the lake surface to the atmosphere (Verburg and Antenucci Citation2010). The stability condition of the ASL is closely related to the thermodynamic processes between the lake surface and the atmosphere, and thereby affects the surface energy exchange (Woolway et al. Citation2017). These drivers mentioned above can be parameterized by the bulk transfer relation, which is commonly used in numerical models for predicting
and
.
The different properties of the overlying air mass under different ASL stratification play an important role in the water–air turbulent energy exchange process. The contribution of the entrainment process induced by downward cold and dry air to heat and water vapor fluxes is enhanced as the atmospheric condition changes from unstable to neutral (Gao et al. Citation2018). High wind speeds contribute to an increase in and
at the air–sea interface under unstable but very-close-to-neutral stratification, due to the downdrafts of cold and dry air caused by detached eddies (Andersson et al. Citation2019). Similar results have also been observed in lake studies insofar as large
and
tend to be concentrated in weakly unstable to neutral stratification (Li et al. Citation2015; Yusup and Liu Citation2016). However, increased lake evaporation has been observed even under stable atmospheric conditions due to the mixing of downward warm and dry air, although turbulent mixing was depressed in this situation (Blanken, Rouse, and Schertzer Citation2003). As the stability represents the mixed contributions of mechanical and thermal convection to turbulence in the ASL, the role of other atmospheric variables (wind speed, vapor pressure difference, and temperature difference) in controlling the water vapor and heat exchange across the lake–atmosphere interface under different stability conditions needs further analysis.
Since the stability condition of lakes is dependent on lake size, latitude, and weather patterns (Woolway et al. Citation2017), the effects of stability on and
need to be investigated at site scale to provide a more comprehensive understanding of the parameterization of turbulent exchange over lake surfaces in numerical models. Erhai Lake is a highland open freshwater lake (25.46°N, 100.10°E) located at the southeastern margin of the Tibetan Plateau, with an altitude of 1978 m. Due to the low latitude and the associated small temperature difference between the lake surface and the atmosphere, the stability condition of Erhai Lake may be different from those lakes in mid- to high-latitude areas. The variability and driving force of surface energy exchange under different ASL stratification in low-latitude highland lakes remains unclear. On the basis of one year of EC measurement data at Erhai Lake in 2015, this study aims to (1) discuss the characteristics of ASL stratification over Erhai Lake; (2) analyze the variations of
and
with ASL stability; and (3) investigate the factors controlling
and
under different ASL stability conditions.
2. Measurements and data processing
2.1 Site description and instruments
The measurement site was located on the west side of Erhai Lake (). The lake lies in a narrow valley stretching from north to south, with the Cangshan Mountains on the west and Yu’an Mountains to the east. It covers an area of 256.5 km2, with a south-to-north length of about 40.5 km and an east-to-west length of about 3–9 km. The average and maximum water depth are 10 m and 20.7 m, respectively. The western shore of Erhai Lake is surrounded by villages and cropland. This region has a subtropical plateau monsoon climate, with a warm–wet season from May to October and a cold–dry season from November to April of the next year. The average annual temperature and precipitation in this region are 15.06°C and 1055 mm, respectively. The lake water is ice-free throughout the year. There are 117 rivers and streams flowing into the lake, but only one natural outlet (Xi’er River). The lake water is usually mixed most of the time, with no obvious thermal stratification (Feng et al. Citation2015). The EC system was installed on a concrete measurement platform approximately 70 m away from the western lake shore. The EC instruments were mounted at 2 m above the platform, including a three-dimensional sonic anemometer (CSAT3, Campbell Scientific) and an open-path infrared gas analyzer (IRGA, LI-7500, LI-COR). The sampling frequency of the EC instruments was 10 Hz. Sensors measured radiation, air temperature, relative humidity, wind speed/direction, and the water temperature profile every minute, recorded as 30-min-averaged values. More information about the measurement site and instruments can be found in our previous study (Liu et al. Citation2015).
2.2 Data processing and quality control
One year of EC measurement data at Erhai Lake in 2015 were analyzed in this study. The raw high-frequency (10 Hz) data were processed into 30-min mean fluxes using the EddyPro software, V.6.1 (LI-COR, Inc. 2015). Latent and sensible heat fluxes were calculated from 30-min mean covariances between the scalars and the vertical wind speed component. Since detailed information on the data post-processing procedures used in this study have already been documented in our previous study (Liu et al. Citation2015), only the quality control procedures are introduced here. The 0-1-2 flag system suggested by Foken et al. (Citation2004) was applied to control the quality of the 30-min fluxes. The data points with flag = 2 were removed in this study. According to the footprint analysis described in Du et al. (Citation2018a), the 90% cumulative footprint distances in the western direction are 177 m and 230 m under unstable and stable conditions, respectively. As the measurement site was close to the western shore of the lake (with a distance of 70 m), the fluxes from the western direction (225° – 315°) were also removed to eliminate the influence of the surrounding land areas.
The ASL stability () is quantified by a dimensionless parameter
, where
is the measurement height and
is the Monin–Obukhov length calculated as
Here, is the frictional velocity (m s−1);
is the von Karman constant (0.4),
is gravitational acceleration (9.8 m s−2),
is the potential temperature (K), and
is the vertical kinematic heat flux (K m s−1). The
and
can be measured directly by the EC system. Other meteorological variables, including wind speed (
), temperature difference (
,
, ◦C), and vapor pressure difference (
,
, kPa) were also analyzed.
and
are the water surface temperature and air temperature, respectively.
is derived from longwave radiation.
and
are the saturated vapor pressure at
and the vapor pressure at
, respectively.
In order to investigate the driving factors of and
under different stability conditions, we divided the stability (
) into six stability ranges. Data points with
and
were removed because there were not enough data (fewer than 20) in these two stability ranges. After the filtering processes mentioned above, about 62.5% of
and 69.8% of
were available for further analysis. The six stability ranges were set as follows: unstable (
, n1 = 1044, n2 = 1078), weakly unstable (
, n1 = 4059, n2 = 4591), near-neutral1 (
, n1 = 1642, n2 = 2068), near-neutral2 (
, n1 = 2177, n2 = 2446), weakly stable (
, n1 = 1864, n2 = 1875), and stable (
, n1 = 168, n2 = 179), where n1 and n2 are the numbers of valid data points for
and
, respectively.
3. Results and discussion
3.1 Characteristics of ASL stability conditions
The ASL stability conditions over Erhai Lake have obvious seasonal and diurnal variations. In terms of seasonal variation ()), weakly stable () and stable (
) stratification usually occurred before July, with a total frequency of 23.3%, but rarely occurred after July (9.8%). Conversely, most of the weakly unstable (
) and unstable (
) stratification occurred after July, with a total frequency of 59.8%, while it was less common from March to June, especially for unstable ranges, with a frequency of < 4%. Such seasonal variations of ASL stability over Erhai Lake are mainly caused by the variation of
, where positive
(water surface temperature
exceeds air temperature
) is usually related to unstable atmospheric conditions. The
was mostly negative during January to June and became positive after June (not shown), which contributed to the occurrence of (weakly) stable stratification during January to June and (weakly) unstable stratification after June. The near-neutral (
) stratification mostly appeared during January to June, with a frequency of 51.7%. A high frequency of near-neutral stratification is related to the large cloud amount in this area and the relatively small temperature difference between the lake surface and the atmosphere (Du et al. Citation2018b). The diurnal variations of stability over Erhai Lake show two different patterns by month. As shown in ), ASL stratifications were stable in the afternoon during 1300 BJT (Beijing Time, one hour sssearlier than local time) to 1600 BJT and near-neutral during the rest of the day from March to June. The stratifications became unstable in daytime and near-neutral at nighttime after July, and the strongest unstable condition occurred during morning hours to midday. Such diurnal variation of stable stratification in late-afternoon and strong unstable stratification in the early morning was also found in a temperate reservoir, but seasonal variation of the ASL stability was not observed (Liu, Zhang, and Dowler Citation2012). In a tropical great lake (Lake Tanganyika) and a large high-altitude lake (Lake Ngoring), a persistent unstable ASL existed during the observation period (Li et al. Citation2016; Verburg and Antenucci Citation2010). This persistent unstable ASL was also found in a small lake on the Tibetan Plateau (Wang et al. Citation2017).
3.2 Variations of latent and sensible heat fluxes with stability conditions
shows the variations of ,
, and meteorological variables with atmospheric stability conditions. Under unstable conditions,
and frictional velocity (
) increased from the unstable (
) to near-neutral1 (
) range, leading to an increase in
and
with the weakening instability, although
decreased at the same time. The increase in
can be mainly attributed to the enhanced wind speed and mechanical turbulent mixing (described by
) when the stratification shifts towards near-neutral. Thus,
and stability seem to have a minor influence on the variation of
under unstable conditions. Such an increasing trend of
with weakening instability under unstable conditions has also been observed in other lakes of different size and latitude (Li et al. Citation2015; Wang et al. Citation2017; Yusup and Liu Citation2016). The maximum
usually occurred under weakly unstable to near-neutral stratification, rather than under strong unstable stratification. Under stable conditions,
dropped from the near-neutral2 (
) to stable (
) range; and conversely,
started to increase, causing a slight decrease in
but a slight increase in
. Large evaporation still occurred under stable stratification. Meanwhile, the average value of
was observed to be greater under stable conditions (
, 94.8 W m−2) than under unstable conditions (
, 82.4 W m−2), as was also the case for
and
(stable: 3.1 m s−1, 1.1 kPa; unstable: 2.5 m s−1, 0.8 kPa). The combination of a relatively larger lake–air vapor pressure difference and wind speed resulted in large evaporation under stable atmospheric conditions. A similar result was also found in a small boreal lake in Sweden (Heikinheimo et al. Citation1999).
Figure 3. Variations of /
and other meteorological variables (
,
,
/
, and
/
) with stability conditions. The black squares and error bars are the average values and the corresponding standard deviations in each stability range, respectively.

The variation of with stability conditions was closely related to
.
decreased with weakening instability under unstable conditions, due to the depressed thermal convection by a decline in
. When the atmospheric condition turned stable,
became negative such that energy was transferred from the atmosphere to the lake surface. Under stable conditions, decreased
and
contributed to the slightly reduced heat transfer as the ASL changed from near-neutral2 (
) to stable (
). The mean values of
,
, and
were 10.8 (
11.4) W m−2, 2.5 (3.1) m s−1, and 2.4 (
1.3) °C under unstable (stable) conditions. The heat exchange over Erhai Lake seems to be smaller than for low-altitude lakes in North America, such as Ross Barnett Reservoir (Liu, Zhang, and Dowler Citation2012), Lake Superior (Blanken et al. Citation2011), and Great Slave Lake (Blanken, Rouse, and Schertzer Citation2003). This might be related to the high frequency of near-neutral conditions caused by the large low-cloud amount in the plateau region and thereby relatively small temperature difference. Low cloud has a cooling effect on the surface energy budget in the southeastern plateau region (Cai, Xu, and Sun Citation2012) and might lead to smaller
over Erhai Lake.
3.3 Driving factors of LE and H under different stability conditions
The coefficient of determination (R2, P 0.05) between the 30-min
/
and the meteorological variables (
,
/
, and
) was calculated to illustrate the driving factors of
and
under different stability ranges (). The correlation between
and
ranged from 0.38 to 0.69 under unstable conditions and from 0.21 to 0.50 under stable conditions. The
had a relatively stronger influence on
under unstable (
) and stable (
) conditions when
was large and wind speed was small, which supports the importance of a large
magnitude on the water vapor exchange across the lake–air interface (Zhang and Liu Citation2014).
had a larger correlation with
than
from weakly unstable (
) to weakly stable (
) conditions. The correlation between
and
was largest under all the stability ranges, with mean R2 values of 0.65 under unstable conditions and 0.56 under stable conditions. However, the correlation coefficients between
and
were not obviously enhanced compared to the correlation between
and
under near-neutral conditions (
), indicating a strong impact of
in near-neutral stratification associated with high wind speed and strong mechanical turbulent mixing.
Table 1. Coefficients of determination (R, p
0.05) for the relationships of
with
,
, and
, and
with
,
, and
, under six stability ranges.
was correlated to
only under stable (
) conditions, with R2 = 0.37, while its R2 values were smaller than 0.1 in other stability ranges.
had a stronger relationship with
under stable conditions, with R2 varying from 0.23 to 0.40, but no significant relationship with
under unstable conditions (R2 < 0.2).
had the largest correlation with
under all the stability ranges. The correlations between
and meteorological variables (
,
, and
) were obviously stronger under stable conditions than under unstable conditions, reflecting the stability conditions having a significant influence on controlling the relative contributions of
,
, and
to transfer heat at the lake–air interface. The mean R2 value between
and
was 0.60 under all stability ranges, while that between
and
was 0.30. The smaller correlation between
and
suggests that the atmospheric stability condition has a stronger influence on
than
in terms of the bulk transfer relationship.
The relative impacts of meteorological variables on heat and water vapor transfer across the lake–air interface were regulated by the ASL stability conditions. Yusup and Liu (Citation2016) studied a large temperate reservoir and found that had a stronger influence on
(
) than
(
) under unstable conditions, while
(
) was the major driver of
(
) under stable conditions. For a small lake in the Tibetan Plateau,
and
were more strongly correlated with
and
than
under unstable conditions, while under weakly unstable to neutral conditions
had a stronger impact on
and
(Wang et al. Citation2017). In Lake Ngoring, the largest freshwater lake on the Tibetan Plateau,
and
made a greater contribution than
to the
and
under unstable conditions, but vice versa under near-neutral and weakly stable conditions (Li et al. Citation2015). The results for Erhai Lake are similar to the results of the two different lakes on the Tibetan Plateau mentioned above, which can be summarized as
having a major influence on
under unstable (
) and stable (
) conditions, while
contributes more to
from the weakly unstable to weakly stable range. The impacts of
and
on
are only significant under stable conditions. The different roles of these atmospheric variables might be attributable to the different lake morphology, geography, and weather conditions, which determine the stability conditions and atmospheric forcing over lake surfaces.
4. Conclusions
Based on one year of data measured by the EC technique over Erhai Lake in 2015, the characteristics and drivers of and
fluxes under different stability conditions were analyzed in this study. Large evaporation still occurred under stable conditions, attributable to the relatively larger wind speed and vapor pressure difference between the lake and the atmosphere. The effect of
on
was more significant than
from the weakly unstable to weakly stable range, whereas under unstable and stable conditions
made a large contribution to
. The impacts of
and
on
were only notable under stable conditions. These results for Erhai Lake have important implications for a better understanding of the parameterization of turbulent fluxes in low-latitude highland lakes, but might not be appropriate for other inland lakes because the stability condition, as well as the water vapor and heat exchange at the water–air interface and its driving factors, will be site-specific and atmospheric-forcing-specific.
Acknowledgments
We appreciate the staff at the Yunnan Provincial Institute of Meteorology and Dali National Climatic Observatory for their help in the maintenance of the measurement site and instruments.
Disclosure statement
No potential conflict of interest was reported by the authors.
Additional information
Funding
References
- Andersson, A., A. Sjöblom, E. Sahlée, E. Falck, and A. Rutgersson. 2019. “Enhanced Air–Sea Exchange of Heat and Carbon Dioxide over a High Arctic Fjord during Unstable Very-Close-to-Neutral Conditions.” Boundary-Layer Meteorology 170: 471–488. doi:10.1007/s10546-018-0408-9.
- Blanken, P. D., W. R. Rouse, A. D. Culf, C. Spence, L. D. Boudreau, J. N. Jasper, B. Kochtubajda, W. M. Schertzer, P. Marsh, and D. Verseghy. 2000. “Eddy Covariance Measurements of Evaporation from Great Slave Lake, Northwest Territories, Canada.” Water Resources Research 36 (4): 1069–1077. doi:10.1029/1999WR900338.
- Blanken, P. D., W. R. Rouse, and W. M. Schertzer. 2003. “Enhancement of Evaporation from a Large Northern Lake by the Entrainment of Warm, Dry Air.” Journal of Hydrometeorology 4 (4): 680–693. doi:10.1175/1525-7541(2003)004<0680:EOEFAL>2.0.CO;2.
- Blanken, P. D., C. Spence, N. Hedstrom, and J. D. Lenters. 2011. “Evaporation from Lake Superior: 1. Physical Controls and Processes.” Journal of Great Lakes Research 37 (4): 707–716. doi:10.1016/j.jglr.2011.08.009.
- Cai, W. Y., X. D. Xu, and J. H. Sun. 2012. “An Investigation into the Surface Energy Balance on the Southeast Edge of the Tibetan Plateau and the Cloud’s Impact.” Acta meteorologica Sinica 70 (4): 837–846. [In Chinese]. doi:10.11676/qxxb2012.069.
- Downing, J. A., Y. T. Prairie, J. J. Cole, C. M. Duarte, L. J. Tranvik, R. G. Striegl, W. H. McDowell, et al. 2006. “The Global Abundance and Size Distribution of Lakes, Ponds, and Impoundments.” Limnology and Oceanography 51 (5): 2388–2397. doi:10.4319/lo.2006.51.5.2388.
- Du, Q., H. Z. Liu, Y. Liu, L. Wang, L. J. Xu, J. H. Sun, and A. L. Xu. 2018a. “Factors Controlling Evaporation and the CO2 Flux over an Open Water Lake in Southwest of China on Multiple Temporal Scales.” International Journal of Climatology 38 (13): 4723–4739. doi:10.1002/joc.5692.
- Du, Q., H. Z. Liu, L. J. Xu, Y. Liu, and L. Wang. 2018b. “The Monsoon Effect on Energy and Carbon Exchange Processes over a Highland Lake in the Southwest of China.” Atmospheric Chemistry and Physics 18 (20): 15087–15104. doi:10.5194/acp-18-15087-2018.
- Dutra, E., V. M. Stepanenko, G. Balsamo, P. Viterbo, P. M. A. Miranda, D. Mironov, and C. Schar. 2010. “An Offline Study of the Impact of Lakes on the Performance of the ECMWF Surface Scheme.” Boreal Environment Research 15 (2): 100–112.
- Feng, J. W., H. Z. Liu, J. H. Sun, and L. Wang. 2015. “The Surface Energy Budget and Interannual Variation of the Annual Total Evaporation over a Highland Lake in Southwest China.” Theoretical and Applied Climatology 126 (1–2): 303–312. doi:10.1007/s00704-015-1585-9.
- Foken, T., M. Göockede, M. Mauder, L. Mahrt, B. Amiro, and W. Munger. 2004. “Post-field Data Quality Control.” In Handbook of Micrometeorology: A Guide for Surface Flux Measurement and Analysis, edited by Lee, X., W. Massman, and B. Law, 181–208. Dordrecht: Kluwer Academic Publishers.
- Gao, Z., H. Liu, D. Li, G. G. Katul, and P. D. Blanken. 2018. “Enhanced Temperature-Humidity Similarity Caused by Entrainment Processes with Increased Wind Shear.” Journal of Geophysical Research: Atmospheres 123 (8): 4110–4121. doi:10.1029/2017JD028195.
- Heikinheimo, M., M. Kangas, T. Tourula, A. Venäläinen, and S. Tattari. 1999. “Momentum and Heat Fluxes over Lakes Tämnaren and Råksjö Determined by the Bulk-aerodynamic and Eddy-correlation Methods.” Agricultural and Forest Meteorology 98–9: 521–534. doi:10.1016/S0168-1923(99)00121-5.
- Lee, X., S. Liu, W. Xiao, W. Wang, Z. Gao, C. Cao, C. Hu, et al. 2014. “THE TAIHU EDDY FLUX NETWORK: An Observational Program on Energy, Water, and Greenhouse Gas Fluxes of a Large Freshwater Lake.” Bulletin of the American Meteorological Society 95 (10): 1583–1594. doi:10.1175/BAMS-D-13-00136.1.
- Li, Z., S. Lyu, Y. Ao, L. Wen, L. Zhao, and S. Wang. 2015. “Long-term Energy Flux and Radiation Balance Observations over Lake Ngoring, Tibetan Plateau.” Atmospheric Research 155: 13–25. doi:10.1016/j.atmosres.2014.11.019.
- Li, Z., S. Lyu, L. Zhao, L. Wen, Y. Ao, and S. Wang. 2016. “Turbulent Transfer Coefficient and Roughness Length in a High-altitude Lake, Tibetan Plateau.” Theoretical and Applied Climatology 124 (3–4): 723–735. doi:10.1007/s00704-015-1440-z.
- Liu, H., Q. Zhang, and G. Dowler. 2012. “Environmental Controls on the Surface Energy Budget over a Large Southern Inland Water in the United States: An Analysis of One-Year Eddy Covariance Flux Data.” Journal of Hydrometeorology 13 (6): 1893–1910. doi:10.1175/JHM-D-12-020.1.
- Liu, H., Y. Zhang, S. Liu, H. Jiang, L. Sheng, and Q. L. Williams. 2009. “Eddy Covariance Measurements of Surface Energy Budget and Evaporation in a Cool Season over Southern Open Water in Mississippi.” Journal of Geophysical Research: Atmospheres 114: 83–84. doi:10.1029/2008JD010891.
- Liu, H. Z., J. W. Feng, J. H. Sun, L. Wang, and A. L. Xu. 2015. “Eddy Covariance Measurements of Water Vapor and CO2 Fluxes above the Erhai Lake.” Science China: Earth Sciences 58 (3): 317–328. doi:10.1007/s11430-014-4828-1.
- Long, Z., W. Perrie, J. Gyakum, D. Caya, and R. Laprise. 2007. “Northern Lake Impacts on Local Seasonal Climate.” Journal of Hydrometeorology 8 (4): 881–896. doi:10.1175/JHM591.1.
- Nordbo, A., S. Launiainen, I. Mammarella, M. Leppäranta, J. Huotari, A. Ojala, and T. Vesala. 2011. “Long-term Energy Flux Measurements and Energy Balance over a Small Boreal Lake Using Eddy Covariance Technique.” Journal of Geophysical Research 116: D02119. doi:10.1029/2010JD014542.
- Rouse, W. R., C. J. Oswald, J. Binyamin, C. R. Spence, W. M. Schertzer, P. D. Blanken, N. Bussieres, and C. R. Duguay. 2005. “The Role of Northern Lakes in a Regional Energy Balance.” Journal of Hydrometeorology 6 (3): 291–305. doi:10.1175/JHM421.1.
- Shao, C., J. Chen, C. A. Stepien, H. Chu, Z. Ouyang, T. B. Bridgeman, K. P. Czajkowski, R. H. Becker, and R. John. 2015. “Diurnal to Annual Changes in Latent, Sensible Heat, and CO2 Fluxes over A Laurentian Great Lake: A Case Study in Western Lake Erie.” Journal of Geophysical Research: Biogeosciences 120 (8): 1587–1604. doi:10.1002/2015JG003025.
- Verburg, P., and J. P. Antenucci. 2010. “Persistent Unstable Atmospheric Boundary Layer Enhances Sensible and Latent Heat Loss in a Tropical Great Lake: Lake Tanganyika.” Journal of Geophysical Research: Atmospheres 115: D11109. doi:10.1029/2009JD012839.
- Wang, B., Y. Ma, W. Ma, and Z. Su. 2017. “Physical Controls on Half-hourly, Daily, and Monthly Turbulent Flux and Energy Budget over a High-altitude Small Lake on the Tibetan Plateau.” Journal of Geophysical Research: Atmospheres 122 (4): 2289–2303. doi:10.1002/2016JD026109.
- Woolway, R. I., P. Verburg, J. D. Lenters, C. J. Merchant, D. P. Hamilton, J. Brookes, E. de Eyto, et al. 2018. “Geographic and Temporal Variations in Turbulent Heat Loss from Lakes: A Global Analysis across 45 Lakes.” Limnology and Oceanography 63 (6): 2436–2449. doi:10.1002/lno.10950.
- Woolway, R. I., P. Verburg, C. J. Merchant, J. D. Lenters, D. P. Hamilton, J. Brookes, S. Kelly, et al. 2017. “Latitude and Lake Size are Important Predictors of Over-lake Atmospheric Stability.” Geophysical Research Letters 44 (17): 8875–8883. doi:10.1002/2017GL073941.
- Yusup, Y., and H. Liu. 2016. “Effects of Atmospheric Surface Layer Stability on Turbulent Fluxes of Heat and Water Vapor across the Water–Atmosphere Interface.” Journal of Hydrometeorology 17 (11): 2835–2851. doi:10.1175/JHM-D-16-0042.1.
- Zhang, Q., and H. Liu. 2014. “Seasonal Changes in Physical Processes Controlling Evaporation over Inland Water.” Journal of Geophysical Research: Atmospheres 119: 9779–9792. doi:10.1002/2014JD021797.