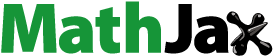
ABSTRACT
In this study, using construction activities as an example, a proposal is made for the time-dependent prediction of the thermal sensation and the performance loss using a cooling vest, under transient conditions. The approach presented here can, mutatis mutandis, be used for any work activity and the use of a cooling vest, whether or not in a building.
Introduction
Climate change, resulting in global warming, is one of the biggest problems of our time. Rising outdoor temperatures will continue in the twenty-first century, with a greater frequency and duration of heat waves. Some regions of the world are more affected than others. Europe is one of the regions of the world where rising global temperatures will adversely affect public health; especially that of the labour force, resulting in a decline in labour productivity. It will be clear that in many situations air conditioning is not an option because it does not provide sufficient cooling or it is a too expensive investment; for example in the situation of construction work. In such a situation, personal cooling systems, such as cooling vests, can be an efficient and financially attractive solution to the problem of discomfort and heat stress. The use of personal cooling systems, in the form of cooling vests, is not only intended to reduce the heat load, in order to prevent disruption of the thermoregulation system of the body, but also to improve work performance. In most studies in the literature, the influence of a cooling vest on the human performance is often limited to the time-dependent measurement and/or mathematical prediction of the skin temperature, the core temperature and skin wetness in order to evaluate heat strain; often when exercising. A time-dependent mathematical prediction of the thermal sensation as well as the performance loss in certain professional situations are not taken into account. This study focuses in particular on the latter. In this paper a proposal is made, using an example with construction work. The question is ‘In what order of magnitude does the comfort and performance improvement lie with the use of a cooling vest for construction workers?’ To answer this question, a calculation study was carried out on the basis of four validated mathematical models, namely a cooling vest model, a thermophysiological human model, a dynamic thermal sensation (DTS) model and a human performance loss model, linked to each other. It was examined whether cooling vests can be objectively compared with each other with this approach and whether an optimal choice can be made for a specific type of work and working environment. The starting points, the calculation results, as well as the findings, are set out in this paper.
Cooling power and cooling capacity cooling vest
As yet there is no standard or guideline that states how the cooling power, the cooling capacity and the maximum cooling time of a cooling vest should be determined. In practice, it is therefore difficult to objectively compare cooling vests and to make an optimal choice for a specific type of work and working environment.
However, each cooling vest can be characterized on the basis of the maximum cooling power (Pmax; in W/m2), the cooling capacity (Auc; in Wh/m2) and the time (tc; in minutes) after which the cooling power is negligible; namely 20 W/m2. The cooling capacity of a cooling vest is then approx. In total, 10% of the amount of heat produced per m2-body surface, with a metabolism of 58.2 W/m2 (a sitting activity; the surface of the trunk is approx. 30% of the total body surface). Ergo below this value can be assumed that a cooling vest provides the body insufficient cooling () (Ciuha, Valencic, and Mekjavic Citation2020).
The simulation with a cooling vest, in this study, is carried out in the same way as Dhiman describes in his thesis (Dhiman Citation1974), i.e. by allowing the cooling of the vest to benefit the trunk of the body, to know:
Herein is: HFskin,trunk is the rate of heat flow into or from segment [kcal/h]; Qskin,trunk is the total metabolic heat production segment[kcal/h]; BCskin,trunk is the convective heat transfer between central blood and segment [kcal/h]; Eskin,trunk is the total evaporative heat segment [kcal /h]; Drytrunk is the dry heat loss segment [kcal/h]; Qcooling vest is the cooling output of the cooling vest [kcal/h]. However, the difference between this study and the study of Dhiman is that the cooling output of the vest does not have a constant power, as assumed by Dhiman, but is time-dependent according to the model in
Cooling vests
In principle, cooling vests can be classified as follows (Ciuha, Valencic, and Mekjavic Citation2020):
Active cooling vests (Pmax = 44–60 W/m2, tC = 151– ∞ min., Auc = 118–331 Wh/m2)
Air cooling vests (e.g. Teijin)
Liquid-perfused cooling vests (e.g. Battery Heated Clothing)
Passive cooling vests (Pmax = 6–92 W/m2, tC = 21–210 min., Auc = 7–164 Wh/m2)
Evaporative cooling vests (e.g. Inuteq International B.V.)
Vests with pcm and gel inserts (e.g. StaCool Industries)
Hybrid cooling vests (Pmax = 57–75 W/m2, tC = 194–226 min., Auc = 127–146 Wh/m2) (e.g. Inuteq International B.V.)
Ad 1 a. Air cooling vests
Air-cooled vests provide a constant flow of (cooled) ambient air or (cooled) compressed air into the micro space between the trunk and vest, promoting heat dissipation through evaporation of sweat and convection around the trunk of the body. As a result, the cooling efficiency depends, among other things, on the moisture of the skin and the air that is drawn in. Actively cooled vests allow only limited freedom of movement when connected to a device that supplies air. There is no such limitation with fans in a cooling vest that are powered by a battery.
Ad 1 b. Liquid-perfused cooling vests
In a liquid-cooled vest, a cooled liquid, usually water, circulates through small tubes, close to the skin, through the fabric of the vest. The liquid is pumped from a container, which may or may not be contained in the vest.
Ad 2 a. Evaporative cooling vests
With this type of cooling vest, the outside is moistened. The body is then cooled by natural evaporation. The cooling vests perform best in an environment with low humidity. The air flow around the body determines the degree of cooling. It is therefore important that no other clothing is worn over the cooling vest.
Ad 2 b. Vests with PCM and gel inserts
In this type of vest there is room for exchangeable cooling elements, consisting of a so-called phase change material or a gel. The elements extract heat from the body through phase change (melting) and conduction. This type of vest is suitable in warm and humid environments and to be worn under protective clothing; for example in melting, welding or extinguishing work.
Ad 3. Hybrid cooling vests
Hybrid cooling vests combine two or more of the cooling concepts described above.
Thermophysiological human model of Stolwijk
For the calculation study, use was made of the mathematical thermophysiological human model of Stolwijk, developed on behalf of NASA (National Aeronautics and Space Administration). The Stolwijk model consists of six segments (namely the head, the trunk, the arms, the hands, the legs and the feet), each with four layers (namely the core, the muscles, the fat layer and the skin layer). The head is represented as a sphere. The other segments are represented as cylinders. Each cylinder consists of the aforementioned four layers. An extra element is the blood, which transfers the heat via convection from and to the layers and from and to the segments themselves. For the parts that are in contact with the environment, the mathematical relationships apply for heat loss via radiation, convection and evaporation as well as the influence of the clothing. For each component dynamic energy balances are drawn up, the metabolism is calculated and the blood flow is determined. The cardiac output, heat production and heat losses through evaporation are obtained by summing them over the segments. The skin blood flow and average skin temperature are calculated by summing the skin blood flow and the skin temperature of each segment, weighted by skin area. Likewise, the average body temperature is obtained from the average of all segment temperatures with their specific heat capacity as a weighting factor. The total heat storage for the whole body is obtained by summing the individual storage per segment. The model can be used to simulate thermophysiology, thermal perception and performance loss under transient conditions. For detailed information about the model, please refer to the literature (Roelofsen Citation2016). The cooling power of a cooling vest, as shown graphically in , is built into the Stolwijk model, in a similar way as Dhiman (Citation1974) has described in his thesis.
ASHRAE seven-point scale
To quantify the thermal sensation, the seven-point psycho-physical ASHRAE scale is used:
Hot: 3
Warm: 2
Slightly warm: 1
Neutral: 0
Slightly cool:−1
Cool:−2
Cold:−3
In general, a thermal sensation of −0.5 to 0.5 is used for the comfort zone, in accordance with category II in NEN-EN-15251 (Citation2007).
Dynamic thermal sensation
In the Stolwijk model, an equation is included to predict the thermal sensation under dynamic conditions, the so-called DTS, originally developed by Fiala (Citation1998), based on the simulated core temperature and the mean skin temperature. The equation for predicting the thermal sensation is based on a large number of independent experiments. Using a multivariate analysis, it was found that the mean skin temperature, the core temperature and the rate at which the mean skin temperature changes are the parameters affecting the thermal sensation under dynamic conditions. The thermal sensation was assessed on the basis of the ASHRAE seven-point scale. The DTS is calculated as follows:
DTS = 3*tanh(fsk + ø+ψ) [-]
Where:
fsk = a1*ΔTsk,m [-]; for ΔTsk,m > 0
fsk = a2*ΔTsk,m [-]; for ΔTsk,m < 0
ΔTsk,m = (Tmean skin – 34.4) [K]
ø = b1*exp(b2/(ΔThy + 0.4) + b3/(ΔTsk,m – 4)) [-]; ø = 0 when ΔThy + 0.4 ≤ 0 or ΔTsk,m – 4 ≥ 0
ΔThy = (Thypothalamic – 37.0) [K]
ψ = (τ- + τ+) / (1 + ø) [-]
τ- = c1*dTsk,m/dt [-]; for dTsk,m/dt < 0
τ+ = c2*(dTsk,m/dt)max.exp(–c3*Δt) [-]; for dTsk,m/dt > 0
Δt = t – t0 [h]
t0 = time of occurrence of highest rate dTsk,m/dt [h]
a1 to c3: regression coefficients obtained through multivariate analysis.
In the studies of Fiala two versions of the DTS were published (Fiala Citation1998; Fiala, Lomas, and Stohrer Citation2003). Both versions are included in the Stolwijk model used here. For more information, please refer to (Roelofsen Citation2016).
Performance loss as a function of the thermal sensation
Mohamed and Korb (Citation2002) found relationships for the performance loss of light, medium-heavy and heavy construction work as a function of the thermal sensation, based on 200 datasets of research conducted by several other scientists. The relationships derived by them were later tested in practice and slightly modified (Mohamed and Korb Citation2003), namely:
Plight = 99.91 – 0.796*PMV – 1.843*PMV2
Pmedium = 99.81 – 1.3*PMV – 2.27*PMV2
Pheavy = 83.952 + 15.09*PMV – 4.76*PMV2
Herein is:
Plight = the performance loss of light construction work (Metabolism < 130 W/m2) [%]
Pmedium = the performance loss of medium-heavy work (130 ≤ Metabolism ≤ 190 W/m2) [%]
Pheavy = the performance loss of heavy work (190 < Metabolism ≤ 350 W/m2) [%]
PMV = predicted mean vote, according to NEN-EN-ISO-Citation7730 (Citation2005) [-].
The PMV model, although developed for the assessment of the thermal sensation under steady-state conditions, provides predictions for transient scenarios with overall good accuracy and relative to other thermal sensation models (Koelblen et al. Citation2018). Experiments of Fiala showed that the predicted DTS and the PMV were in agreement (Fiala Citation1998), so in this study, the DTS is used instead of the PMV.
Research of Gao et al.
A major determinant of cooling vest effectiveness would be the drop in trunk skin temperature that correlates significantly with the improved sensation and comfort. This trunk skin temperature drop is simulated by the used thermophysiological human model. The effectiveness of the vest stems from the local cooling of the most influential segment on comfort and sensation (trunk has the highest heat development in the body and has a large surface area). In addition, a dynamic human model is used to simulate the overall comfort in a uniform environment, based on, among other things, the average skin temperature. The extent to which this could influence the results has been verified through research conducted by Gao et al. (Citation2012) and Gao, Kuklane, and Holmer (Citation2010), because these studies were a good fit with the manikin research of Ciuha, Valencic, and Mekjavic (Citation2020) and the overall thermal sensation as a function of time was considered. This is not really the case in other studies in the literature, which have searched for a mathematical model for a cooling vest (Jansen and Teunissen Citation2022; Itani et al. Citation2016; Mokhtari Yazdi, Sheikhzadeh, & Borhani, Citation2014).
In the study of Gao et al. (Citation2012), cooling vests with PCM were measured on a thermal manikin before studies on human subjects. Eight male subjects participated in the study in a climatic chamber (Tair = 34_C, RH = 60% and Vair = 0.4 m/s). The cooling power was firstly measured on the thermal manikin Tore with 17 individually controlled (heating and measuring) zones. Constant manikin surface temperature (34°C) and constant power (20 W/m2) control modes were used respectively to measure the cooling power of the PCMs and the cooling effect on skin temperatures. Subjects rectal temperature, skin temperatures, thermal sensation, thermal comfort and skin wetness without (first 30 min) and with the cooling vest (last 60 min) were measured when the subjects carried out simulated office work at Tair = 34°C, RH = 60%, Vair = 0.4 m/s.
For the simulation of the study of Gao et al. (Citation2012), according to the approach described above, the measured average trunk heat loss in time is approximate by a Gauss curve (see ). The other principles are:
Metabolic rate: 60.9 W/m²
Tair = Tmrt = 34°C
Vair = 0.4 m/s
RH = 60%
Intrinsic clo-value = 0.24 (without cooling vest) (Gao Citation2022)
Intrinsic clo-value = 0.33 (with cooling vest) (Gao Citation2022)
PCM21 cooling vest (Auc = 43.6 Wh/m², tc = 99 min, Pmax = 30 W/m2).
The measured and calculated trunk skin temperature has been displayed in .
The measured and calculated overall thermal sensation has been displayed in .
In this case, Fiala’s first version of the DTS equation (Fiala Citation1998) appears to best approach the experimental results, for the time being.
Variant calculations
To get an impression of the comfort and performance improvement through the use of a cooling vest for construction workers, two variant calculations were performed using the aforementioned human model and cooling vest model. The following principles have been used for this:
Activity
Light activity: 110 W/m2 (e.g. wallpapering and painting)
Medium-heavy activity: 170 W/m2 (e.g. tiling and bricklaying)
Clothing
Intrinsic clo value: 0.24 clo (without cooling vest)
Intrinsic clo value: 0.33 clo (with cooling vest)
A PCM21 cooling vest (Pmax = 30 W/m2, tc = 99 min, Auc = 43.6 Wh/m2) (Gao et al. Citation2012).
Environmental condition
Air temperature: 34°C
Mean radiant temperature: 34°C
Relative humidity: 30%
Air velocity: 0.4 m/s.
The scenario considered concerns an hour of light/medium-heavy activity, without cooling vest and an hour of light/medium-heavy activity, with cooling vest.
The calculation results are shown graphically in and . It can clearly be seen to what extent the overall thermal sensation and the loss of performance, as a result of the thermal load, are influenced by the cooling of the cooling vest chosen here.
Conclusion and advice
Based on the foregoing consideration, the following is concluded and recommended:
Using measurements of the cooling power and cooling capacity of cooling vests in combination with a mathematical thermophysiological model, equivalent to the Stolwijk model or more advanced, allow reasonably accurate thermal simulations of the human body’s response to a wide range of environmental conditions.
In this study, using construction activities as an example, a proposal is made for the time-dependent prediction of the overall thermal sensation and the performance loss using a cooling vest, under transient conditions.
It should be clear that, in principle, the approach can be used for any work activity and the use of a cooling vest, whether or not in a building.
On the basis of the proposal, it is possible to objectively compare cooling vests and to make an optimal choice for a specific type of work and working environment.
Each cooling vest can be characterized on the basis of the maximum cooling power (Pmax; in W/m2), the cooling capacity (Auc; in Wh/m2) and the time (tc; in min) after which the cooling power is negligible ().
In order to objectively compare cooling vests, a (preferably International and/or European) standard/guideline must be compiled to determine the cooling power and the cooling capacity of cooling vests.
Using a cooling vest can have a significant beneficial effect on the thermal sensation and the loss of performance, depending on the thermal load on the body ( and ).
In situations where air conditioning is not an option, the use of cooling vests is an efficient and financially attractive solution to the heat stress of construction workers, as
the investment is small.
it increases the allowable exposure time under warm conditions.
it improves physical and cognitive performance.
it, after exertion and strain, promotes comfort and recovery and reduces fatigue.
It is recommended to implement the use of cooling vests in the construction process, so that employees can use them if necessary or desired.
Disclosure statement
No potential conflict of interest was reported by the author(s).
Additional information
Notes on contributors
C. P. G. Roelofsen
C. P. G. Roelofsen is a research fellow Healthy Urban Living & Working at the faculty of Industrial Design Engineering. His current research interests involve indoor environmental quality, wellbeing and human performance engineering.
K. M. B. Jansen
K. M. B. Jansen is professor of Emerging Materials at the faculty of Industrial Design Engineering. His current interests involve electronic textiles, smart materials, shape morphing materials and electroluminescence.
References
- Ciuha, U., T. Valencic, and I. B. Mekjavic. 2020. “Cooling Efficiency of Vests with Different Cooling Concepts Over 8-Hour Trials.” Ergonomics 64: 1–14.
- Dhiman, B. S. 1974. “Simulation of a Human Thermoregulatory System with Dry Ice Cooling.” M.Sc. thesis, Kansas State University, Industrial Engineering, Manhattan.
- Fiala, D. 1998. “Dynamic Simulation of Human Transfer and Thermal Comfort.” Ph.D thesis, Montfort University Leicester/Fh Stuttgart-Hochschule fur Technik, Institute of Energy and Sustainable Development/Jospeh-von-Egle Institut fur angewandte Forschung, Leicester.
- Fiala, D., K. Lomas, and M. Stohrer. 2003. “First Principles Modelling of Thermal Sensation Responses in Steady State and Transient Boundary Conditions.” ASHRAE Transactions 109 (1): 179–186.
- Gao, C. 2022, October 24. Personal message.
- Gao, C., K. Kuklane, and I. Holmer. 2010. “Cooling Vests with Phase Change Material Packs: The Effects of Temperature Gradient, Mass and Covering Area.” Ergonomics 53 (5): 716–723.
- Gao, C., K. Kuklane, F. Wang, and I. Holmer. 2012. “Personal Cooling with Phase Change Materials to Improve Thermal Comfort from a Heat Wave Perspective.” Indoor Air 22: 523–530.
- Itani, M., D. Ouahrani, N. Ghaddar, K. Ghali, and W. Chakroun. 2016. “The Effect of PCM Placement on Torso Cooling Vest for an Active Human in hot Environment.” Building and Environment 107: 29–42.
- Jansen, K., and L. Teunissen. 2022. “Analytical Model for Thermoregulation of the Human Body in Contact with a Phase Change Material (PCM) Cooling Vest.” Thermo 2: 232–250.
- Koelblen, B., A. Psikuta, A. Bogdan, S. Annaheim, and R. M. Rossi. 2018. “Thermal Sensation Models: Validation and Sensitivity Towards Thermo-Physiological Parameters.” Building and Environment 130: 12.
- Mohamed, S., and S. Korb. 2002. “Thermal Environment Effects on Construction Workers’ Productivity.” Work Study 51 (6): 297–302.
- Mohamed, S., and S. Korb. 2003. “Thermal Environment Effects on Construction Workers’ Productivity: Some Evidence from Thailand.” Building and Environment 38: 339–345.
- Mokhtari Yazdi, M., Sheikhzadeh, M., & Borhani, S. (2014). Modeling the Heat Transfer in a PCM Cooling Vest. The Journal of The Textile Institute, 1003-1012.
- NEN-EN-15251. 2007. Indoor Environmental Input Parameters for Design and Assessment of Energy Performance of Buildings Addressing Indoor air Quality, Thermal Environment, Lighting and Acoustics. Delft: NNI.
- NEN-EN-ISO-7730. 2005. Ergonomics of the Thermal Environment - Analytical Determination and Interpretation of Thermal Comfort Using Calculation of the PMV and PPD Indices and Local Thermal Comfort Criteria (ISO 7730:2005.IDT). Delft: Nederlands Normalisatie Instituut.
- Roelofsen, P. 2016. “Modelling Relationships Between a Comfortable Indoor Environment, Perception and Performance Change.” Ph.D thesis, December 14. Accessed October 21, 2021. www.repository.tudelft.nl. doi:10.4233/uuid:e7778a5c-3013-40ed-9567-bceaffc57ab9