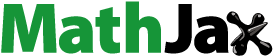
Abstract
This study examined the effects of different high-heeled footwear heights on lower extremity medial tibiofemoral cartilage mechanics and longitudinal failure probability, using musculoskeletal simulation and probabilistic failure modelling approaches. The current investigation examined 24 participants, walking in four different footwear (high heel, medium heel, low heel, and trainer). Walking kinematics were collected using an 8-camera motion capture system and kinetics via an embedded force plate. Medial tibiofemoral loading was explored using musculoskeletal simulation and cartilage probability via probabilistic modelling. Distance travelled per day was inputted into the model as 6.0 km per day. This was modelled as participants completing all 6.0 km in the trainer condition whereas in the heeled footwear, this was modelled as participants completing 3.0, 2.4, 1.8, 1.2 and 0.6 km in the heels, and the remaining distance undertaken in the trainers. Cartilage forces and strains were significantly greater in the high (force = 3.39BW & strain = 0.26), medium (force = 2.98BW & strain = 0.22) and low heels (force = 2.91BW & strain = 0.22) compared to the trainer (force = 2.52BW & strain = 0.19). Cartilage failure probability was significantly greater in the high (3.0 km = 21.26%, 2.4 km = 18.45%, 1.8 km = 18.15%, 1.2 km = 17.90% & 0.6 km = 17.52%), medium (3.0 km = 9.97%, 2.4 km = 9.86%, 1.8 km = 9.76%, 1.2 km = 9.66% & 0.6 km = 9.55%) and low heels (3.0 km = 9.13%) compared to the trainer (1.89%), and in the high heel condition decreased linearly alongside reductions in modelled walking distance. The findings from the current investigation show that high heels of increasing height have a profoundly negative influence on medial knee cartilage health and strongly advocate that walking distance in heeled footwear and the heel height itself be reduced wherever possible.
Introduction
The knee joint plays an important role in load bearing during walking and other common daily activities (D'Lima et al., Citation2012). However, knee osteoarthritis (OA) is the most common form of lower limb osteoarthritis, a major source of disability worldwide (Heidari, Citation2011) and imposes an extremely high economic burden (Martins et al., Citation2021). Causal factors in the disease’s initiation and progression are complex and contentious, but mechanical load imposed onto the cartilage itself during gait and other everyday activities appears to be an important factor. Therefore, due to factors such as age, excessive body mass or previous injury, the negative effects of excessive knee joint mechanical loads begin to emerge, and the risk of knee disorders such as OA increase (Eitner et al., Citation2017). Knee OA itself represents a degenerative articular disease, caused by erosion and deterioration of the articular cartilage within the knee joint (Chen et al., Citation2017), and those with knee OA experience ongoing pain and stiffness (Alshami, Citation2014). OA at the knee joint has been shown to be present in as many as 10% of individuals over the age of 55 (Losina et al., Citation2013), and importantly over 90% of knee OA cases are observed in the medial tibiofemoral compartment (Wise et al., Citation2012). This is because during traditional activities of daily life over 60% of the total load borne by the tibiofemoral joint passes through the medial compartment of the knee (Farrokhi et al., Citation2013).
Heeled footwear have been utilised for over 400 years and are adopted daily in as many as 69% of females (Linder & Saltzman, Citation1998). Heeled designs therefore persist as one of the fundamental features of female footwear modalities, and 21st century fashion practices continue to promote the sustained utilisation of high-heels (Hong et al., Citation2005). However, despite frequent usage, there are long-held apprehensions concerning the enduring impact of high-heels on women’s musculoskeletal wellbeing (Linder & Saltzman, Citation1998). High-heeled footwear feature a slim base of support, and mechanically position the ankle joint into a plantarflexed position, mediating kinematic and kinetic alterations in lower extremity biomechanics during gait (Cronin, Citation2014; Sinclair et al., Citation2019).
Importantly, previous biomechanical analyses have shown that stance phase knee adduction moments were statistically larger when walking in high-heels (Simonsen et al., Citation2012; Barkema et al. Citation2012; Kerrigan et al., Citation1998). As the knee adduction moment is often adopted as a pseudo measurement of medial tibiofemoral compartment loading, this has led to the proposition that high heels may place wearers at risk from tibiofemoral joint OA. As tibiofemoral joint OA is renowned for its increased prevalence in females (Hame & Alexander, Citation2013), this makes the utilisation of high heels particularly concerning.
However, although extremely important, this proposition cannot be explored using resultant knee joint moments from inverse dynamics to quantify knee joint kinetics during gait. Joint moments represent global indices of joint loading, and therefore are not characteristic of localised joint loading (Herzog et al., Citation2003). In recent years, considerable advances in musculoskeletal simulation modelling have been made (Delp et al., Citation2007), allowing skeletal muscle kinetics to be simulated during movement and utilised to quantify muscle driven indices of lower extremity joint reaction forces (Sinclair et al., Citation2019). Furthermore, although indices of joint kinetics are now feasible through simulation analyses, there remain challenges in exploring the effects of different conditions and joint loading scenarios on the initiation and time course of knee OA. Therefore, computational probabilistic modelling of cartilage mechanics and accumulative damage may be valuable for quantifying the probability of osteoarthritic degeneration probability over a lifetime of cyclic loading (Miller & Krupenevich, Citation2020).
Therefore, the aim of the current investigation was to explore the effects of different heeled footwear heights on medial tibiofemoral cartilage mechanics and lifetime failure probability in relation to non-heeled footwear using a combined musculoskeletal simulation and computational modelling approach. The findings from this investigation will yield new information firstly on the effects of heeled footwear on medial tibiofemoral cartilage mechanics during walking, but also on lifetime failure probability in relation to non-heeled footwear.
This study tests the hypothesis that heeled footwear will increase medial tibiofemoral cartilage loading mechanics and also lifetime failure probability in relation to non-heeled footwear and also that both cartilage loading and probability of cartilage failure will be greatest in the highest heel height conditions.
Methods
Participants
Twenty-four female participants (age 29.89 ± 5.17 years, height 1.66 ± 0.10 m, body mass 64.35 ± 6.36 kg and body mass index 23.49 ± 2.78) volunteered to take part in this study. Using data from our previous work (Sinclair et al., Citation2019) and a mean ± SD difference in peak compressive medial tibiofemoral force of 0.37 ± 0.60 BW between conditions, it was determined that in order to achieve α = 5% and β = 0.80, that 24 participants would be required in this investigation. All participants were free from pathology at the time of data collection and provided written informed consent, in accordance with the principles outlined in the Declaration of Helsinki. The procedure utilised for this investigation was approved, by a university ethical committee (REF 637).
Experimental footwear
The footwear used during this study consisted of traditional footwear (New Balance 1260 v2; ), high heels (10 cm heel; ), medium heels (7 cm heel; ), and low heels (4 cm heel; ) in sizes 3–6 in UK. The heeled footwear were identical with the exception of the heel heights.
Procedure
Participants walked across a 22 m Biomechanics Laboratory, striking an embedded piezoelectric force platform (Kistler Instruments Ltd., Winterthur, Switzerland)—which sampled at 1000 Hz—with their right (dominant) foot. A self-selected walking velocity was adopted to improve ecological validity and allow the true longitudinal effects of heeled footwear on medial tibiofemoral cartilage to be explored, as a fixed velocity in the four different footwear conditions would not be feasible in the real world. Five successful trials were collected in each footwear condition, with a successful trial being one where the foot made full contact with the force platform, and with no evidence of gait modifications due to the experimental conditions. Kinematic and ground reaction force (GRF) data were synchronously collected. Kinematic data were captured at 250 Hz via an 8-camera motion analysis system (Qualisys Medical AB, Gothenburg, Sweden). Dynamic calibration of the motion capture system was performed before each data collection session.
Body segments were modelled in 6 degrees of freedom using the calibrated anatomical systems technique (Cappozzo et al., Citation1995). To define the anatomical frames of the thorax, pelvis, thighs, shanks, and feet, retroreflective markers were placed at the 7th cervical vertebrae (C7), 12th thoracic vertebrae (T12), and xiphoid process landmarks, and also positioned bilaterally onto the acromion process, iliac crest, anterior superior iliac spine (ASIS), posterior super iliac spine (PSIS), medial and lateral malleoli, medial and lateral femoral epicondyles, greater trochanter, calcaneus, and 1st and 5th metatarsals. Intra-rater reliability for the researcher responsible for positioning of the aforementioned anatomical markers has been shown to be excellent (Sinclair et al., Citation2014). The centre’s of the ankle and knee joints were delineated as the midpoints between the malleoli and the femoral epicondyle markers (Graydon et al., Citation2015; Sinclair et al., Citation2015). The hip joint centre was determined using a regression equation that uses the positions of the ASIS markers (Sinclair et al., Citation2014). Carbon-fibre tracking clusters comprising four non-linear retroreflective markers were positioned onto the thigh and shank segments. In addition, the foot segments were tracked via the calcaneus, first metatarsal, and fifth metatarsal, the pelvic segment was tracked using the PSIS and ASIS markers, and the thorax segment was tracked using the T12, C7, and xiphoid markers. Static calibration trials were carried out, allowing for the anatomical markers to be referenced in relation to the tracking markers/clusters. The Z (transverse) axis was oriented vertically from the distal segment end to the proximal segment end. The Y (coronal) axis was oriented in the segment from posterior to anterior. Finally, the X (sagittal) axis orientation was determined using the right-hand rule and was oriented from medial to lateral ().
Processing
Four total data processing analyses were undertaken that used the walking biomechanics data to estimate long-term medial tibiofemoral cartilage failure probability; 1. initial kinematic processing, 2. medial tibiofemoral forces, 3. medial tibiofemoral contact mechanics and 4. medial tibiofemoral cartilage failure probabilistic modelling.
Initial kinematic processing
Dynamic trials were digitised using Qualisys Track Manager (Qualisys Medical AB, Gothenburg, Sweden) in order to identify anatomical and tracking markers, and then exported as C3D files to Visual 3D (C-Motion, Germantown, MD, USA). All data were linearly normalised to 100% of the stance phase, which was delineated as the duration over which 20 N or greater of vertical GRF was applied to the force platform (Sinclair et al., Citation2011). GRF data and marker trajectories were smoothed with cut-off frequencies of 50 Hz and 6 Hz, respectively, using a low-pass Butterworth 4th-order zero-lag filter. Kinetic and kinematics cut-off frequencies were obtained using residual analysis (Sinclair et al., Citation2013). Walking velocity was quantified within Visual 3D, using the linear velocity of the model centre of mass in the anterior direction (Sinclair et al., Citation2021) and stride length was determined by calculating the difference in the anterior position of the foot’s centre of mass at footstrike between initial and subsequent ipsilateral foot contacts (Sinclair et al., Citation2021). Cadence (steps/min) was also extracted by calculating the time between initial and subsequent ipsilateral foot contacts and determining the number of footfalls that would be achieved per minute (Sinclair et al., Citation2022).
Medial tibiofemoral forces
Following this, data during the stance phase were exported from Visual 3D into OpenSim 3.3 software (Simtk.org). A validated musculoskeletal model was used to process the biomechanical data, which were scaled to account for the anthropometrics of each participant. The model with 12 segments, 19 degrees of freedom, and 92 musculotendon actuators (Lerner et al., Citation2015) was used to estimate lower extremity muscle and joint forces. A residual reduction algorithm (Delp et al., Citation2007) was first used to resolve dynamic inconsistency between the kinematics of the measured GRF and the model, and muscle kinetics were then quantified using static optimisation, as described by Steele et al. (Citation2012). As muscle forces are the main determinant of joint compressive forces (Herzog et al., Citation2003), following the static optimisation process the peak normalised compressive medial tibiofemoral joint force (BW) was calculated via the joint reaction analysis function within OpenSim, using the muscle forces generated from the static optimisation process as inputs (Delp et al., Citation2007). Finally, the medial compressive tibiofemoral cumulative load was quantified by dividing the average tibiofemoral contact force by the stride length in accordance with Miller and Krupenevich (Citation2020). Sinclair et al. (Citation2021) importantly showed that vastus intermedius, vastus lateralis and vastus medialis muscle forces at the instance of peak compressive joint force, were the strongest predictors of peak medial tibiofemoral joint loading during walking. Therefore, to determine the mechanisms responsible for any alterations in peak medial tibiofemoral kinetics between footwear conditions, the forces (BW) quantified during static optimisation for the aforementioned muscles were quantified at the instance of peak joint force and extracted for statistical analysis.
Medial tibiofemoral contact mechanics
The aforementioned peak compressive medial tibiofemoral contact forces during the stance phase of walking were utilised as inputs into a model of medial knee contact mechanics which was utilised to determine the peak compressive stress and strain indices in the tibiofemoral cartilage using MATLAB code adapted from Miller and Krupenevich (Citation2020). The medial tibiofemoral contact mechanics model was based on that developed and described by Nuño and Ahmed (Citation2001).
The medial aspect of the femoral condyle was delineated as two convex arcs representing its anterior and posterior components from a sagittal perspective and a single arc from a coronal posterior viewpoint, whereas the tibial plateau was modelled conversely as a concave curve. The radius of the tibial arc in frontal plane was assumed to be 21 mm and the radii of the anterior and posterior aspects of the femoral arc in sagittal plane were 35.0 mm and 18.9 mm respectively. The tibial segment was assumed to be held in fixed position in space but in accordance with the Nuño and Ahmed (Citation2001) model, the femur featured two variable parameters: the height of the knee flexion axis relative to the tibia and the flexion angle itself. The cartilage within the tibiofemoral joint itself was depicted as a series of spring elements upon the tibial plateau, with an unloaded height of 5.0 mm (Liu et al., Citation2010). The modelled cartilage elements themselves were assumed to exhibit a nonlinear elastic stress–strain relationship (Blankevoort et al., Citation1991). Briefly medial tibiofemoral contact stresses (σ) and strains (ε) were calculated based on EquationEquations 1(1)
(1) and Equation2
(2)
(2) .
(1)
(1)
(2)
(2)
Peak compressive tibiofemoral contact stresses (MPa) and strains were calculated as a function of the loaded cartilage modulus at this joint, the magnitude of the compression of the modelled contact elements and the modelled number of contact elements which was considered to be 7326 accounting for a modelled distance between elements of 0.5 mm and the aforementioned tibial and femoral arc radii. The tibiofemoral cartilage moduli differed as a function of the location of the loaded contact elements as some were concealed by the medial meniscus. The moduli included in the model for the femoral cartilage, unconcealed tibial cartilage and concealed tibial cartilage, were 8.6, 4.0 and 10.1 MPa (Shepherd & Seedhom, Citation1999). The medial meniscus modulus was considered to be 1.3 MPa and the meniscus itself was modelled to conceal 46% of the tibial plateau (Danso et al., Citation2015; Bloecker et al., Citation2013). The cartilage and menisci were delineated with a Poisson’s ratio of = 0.45.
The knee flexion angle input into the model was the angle from the initial kinematic processing analysis at which the peak medial tibiofemoral contact force (obtained from the medial tibiofemoral force processing) occurred. The vertical height of the knee flexion axis was initially set so that the cartilage was unloaded and then incrementally decreased until the contact force matched that from the input medial tibiofemoral contact force. With distinct modelled radii for the anterior and posterior aspects of the medial femur, the area of cartilage under load differed as a function of the knee flexion angle (Henderson et al., Citation2011). Translational mechanics of the knee joint were not included into the model as the medial aspect of the femoral condyle typically remains close to the centre of the tibial plateau despite changes in the knee flexion angle (DeFrate et al., Citation2004).
Medial tibiofemoral cartilage failure probabilistic modelling
In accordance with Miller and Krupenevich (Citation2020), cartilage failure at the medial aspect of the tibiofemoral joint was delineated as macroscopic plastic deformation typical in early-stage osteoarthritic degeneration (Weightman et al., Citation1973). As the median age of knee OA diagnosis is 55 years and 9.29% of the US population is diagnosed with symptomatic knee OA by age 60 years (Losina et al., Citation2013), the probability of cartilage failure was calculated over a period of 42 years, from skeletal maturity aged 18 until 60 years of age (Johnson et al., Citation2012). Probability of failure was calculated using probabilistic models of tissue damage and repair (Taylor, Citation1998; Taylor & Kuiper, Citation2001; Taylor et al., Citation2004), with cartilage compressive strain as its equivalent for damage. In our previous work (Sinclair et al., Citation2023) we have undertaken sensitivity analyses to determine the sensitivity of the key cartilage failure determinant, i.e., peak compressive tibiofemoral strain to alterations (within feasible biological/anthropometric ranges) of each modelled parameter separately whilst maintaining the others at their modelled values.
The probability of cartilage failure at the specified time (i.e. 42 years) was expressed as a cumulative function of the modelled cartilage experiencing loading cycles over a specific distance each day with the stride length from the initial kinematic processing using EquationEquation 3(3)
(3) .
(3)
(3)
In EquationEquation 3(3)
(3) the reference stressed cartilage (78.5 mm3), the Weibull exponent (14.3) and the power law exponent (12.9) were all constants in the cartilage failure probability model. Time until failure was and calculated using EquationEquation 4
(4)
(4) .
(4)
(4)
In EquationEquation 4(4)
(4) , the time until failure is representative of the time at which 63.2% of cases would fail when experiencing the modelled number and magnitude of cartilage strains. Distance travelled per day was inputted into the model as 6.0 km per day which was the approximate distance that would have been completed had 7000 steps (the number now considered optimal for health and wellbeing (Paluch et al., Citation2021) been completed per day, based on the stride lengths obtained from the trainer footwear condition. In the trainer condition, this was modelled as participants completing all 6.0 km per day (i.e. with all of the aforementioned inputs being derived from the trainer condition). In each of the heeled footwear, this was modelled as participants completing 3.0, 2.4, 1.8, 1.2 and 0.6 km (i.e. 50, 40, 30, 20 and 10% of total daily distance) in the heels and the remaining distances undertaken in the trainer, as it is unlikely that heeled footwear would be utilised throughout the entire day. The number of daily loading cycles i.e. steps per day in each footwear and distance condition was calculated by dividing the modelled daily distance by the step length (Supplementary material 1). The Weibull coefficient (1.03), power law coefficient (1.0) and power law exponent (12.9) were all constants in the time until failure model. Values for the power law and Weibull parameters were obtained from Miller and Krupenevich (Citation2020), who fit a power law for cycles to failure to the data from Riemenschneider et al. (Citation2019).
EquationEquation 3(3)
(3) represents the probability of failure of cartilage in vitro. As living cartilage possesses some innate ability to partially recover from strain-imposed damage over several years (Nakamura et al., Citation2008), the probability of medial tibiofemoral cartilage repair was therefore modelled using EquationEquation 5
(5)
(5) .
(5)
(5)
In EquationEquation 5(5)
(5) , time until repair (5.0 years) and the cartilage repair exponent (5.2) were constants extracted from Miller and Krupenevich (Citation2020) and the time until repair was modelled as the duration after which repair would be expected in 63.2% of cases of damage.
Repair itself was integrated intoEquation Equation 3(3)
(3) in accordance with Miller and Krupenevich (Citation2020) by deriving a probability density function that determines the instantaneous probability of failure at a given time point in time. This process is outlined in EquationEquation 6
(6)
(6) .
(6)
(6)
The probability density function is then multiplied by the cumulative probability that repair has not occurred yet (i.e. 1 − Probability of repair) and then integrated over time to determine the probability of failure taking into account repair. This process is outlined in EquationEquation 7(7)
(7) .
(7)
(7)
Miller and Krupenevich (Citation2020) proposed that the aforementioned modelling of repair is potentially optimistic for cartilage, owing to the assumption that damage occurring at a time point within the model will be repaired by this time + time to repair, and that ultimately almost all damage will eventually fully repair.
Statistical analyses
For each biomechanical and cartilage failure outcome variable and footwear/distance condition, means and standard deviations (SD) were calculated. To compare biomechanical outcomes across the four footwear conditions, linear mixed effects models with repeated measures were adopted. Furthermore, linear mixed effects models with repeated measures were also utilised to contrast cartilage failure indices between footwear within each distance condition (e.g. footwear differences whilst walking 3 km in heeled footwear) and also between distances within each heeled footwear (e.g. differences between 0.6 and 3.0 km in the low heels). Linear mixed effects models were undertaken using the restricted maximum-likelihood and compound symmetry methods, with footwear/distance condition modelled as a fixed factor and random intercepts by participants (Sinclair et al., Citation2021). All statistical analyses were conducted using SPSS v27 (IBM, SPSS). Statistical significance for all analyses was accepted at the p < 0.05 level, and in the interests of conciseness, only outcomes that presented statistical significance are presented in the results section.
Results
Initial kinematic processing
Walking velocity was significantly slower in the low (p = 0.002), medium (p = 0.004) and high (p = 0.001) heel conditions, in relation to the trainer. Furthermore, walking velocity was also significantly slower in the high heels compared to low (p = 0.002) and medium (p = 0.001) conditions ().
Table 1. Kinematic temporal for each footwear conditions.
Stride length was significantly shorter in the low (p < 0.001), medium (p < 0.001) and high (p < 0.001) heel conditions, compared to the trainer. Furthermore, stride length was significantly shorter in the high heels compared to low (p < 0.001) and medium (p = 0.001) conditions ().
Medial tibiofemoral forces & muscle forces
Peak compressive medial tibiofemoral force was significantly greater in the low (p < 0.001), medium (p = 0.001) and high (p < 0.001) heels compared to the trainer condition. Furthermore, peak compressive medial tibiofemoral force was significantly greater in the high compared to low (p < 0.001) and medium heels (p = 0.001) ().
Table 2. Medial tibiofemoral forces and muscle forces for each footwear condition.
Medial tibiofemoral cumulative load was significantly greater in the low (p < 0.001), medium (p < 0.001) and high (p < 0.001) heels compared to the trainer condition. Furthermore, medial tibiofemoral cumulative load was significantly greater in the high compared to low (p < 0.001) and medium heels (p < 0.001) ().
Vastus intermedius force was significantly greater in the high (p < 0.001) and medium (p = 0.026) conditions compared to trainer, and also in the high heels in relation to medium (p = 0.001) and low (p < 0.001) conditions. Vastus lateralis force was significantly greater in the high (p < 0.001) and medium (p = 0.046) conditions compared to trainer, and also in the high heels in relation to medium (p < 0.001) and low (p < 0.001) conditions. Finally, vastus medialis force was significantly greater in the high (p < 0.001) and medium (p = 0.049) conditions compared to trainer, and also in the high heels in relation to medium (p < 0.001) and low (p < 0.001) conditions ().
Medial tibiofemoral contact mechanics
Peak medial tibiofemoral compressive stress was significantly greater in the low (p < 0.001), medium (p < 0.001) and high (p < 0.001) heels compared to the trainer condition. Furthermore, peak medial compressive stress was significantly greater in the high compared to low (p < 0.001) and medium heels (p < 0.001) ().
Table 3. Medial tibiofemoral contact mechanics for each footwear condition.
Medial peak tibiofemoral compressive strain was significantly greater in the low (p < 0.001), medium (p < 0.001) and high (p < 0.001) heels compared to the trainer condition. Furthermore, peak medial compressive strain was significantly greater in the high compared to low () and medium heels (p < 0.001) ().
Medial tibiofemoral cartilage failure probabilistic modelling
Effects of footwear
When completing 3.0 km daily in the heeled conditions, probability of failure with repair was significantly greater in the low (p = 0.046), medium (p = 0.032) and high (p = 0.003) heels compared to the trainer condition. Furthermore, probability of failure with repair was significantly greater in the high compared to low (p = 0.005) and medium heels (p = 0.006) (; ).
Figure 3. Average medial tibiofemoral cartilage failure time series probabilities in the high heels in each experimental distance condition.

Figure 5. Average medial tibiofemoral cartilage failure time series probabilities in the low heels in each experimental distance condition.

Table 4. Medial tibiofemoral cartilage failure probabilistic parameters for each footwear and distance condition.
When completing 2.4 km daily in the heeled conditions, probability of failure with repair was significantly greater in the medium (p = 0.033) and high (p = 0.004) heels compared to the trainer condition. Furthermore, probability of failure with repair was significantly greater in the high compared to low (p = 0.009) and medium heels (p = 0.033) (; ).
When completing 1.8 km daily in the heeled conditions, probability of failure with repair was significantly greater in the medium (p = 0.032) and high (p = 0.005) heels compared to the trainer condition. Furthermore, probability of failure with repair was significantly greater in the high compared to low (p = 0.009) and medium heels (p = 0.035) (; ).
When completing 1.2 km daily in the heeled conditions, probability of failure with repair was significantly greater in the medium (p = 0.034) and high (p = 0.005) heels compared to the trainer condition. Furthermore, probability of failure with repair was significantly greater in the high compared to low (p = 0.037) and medium heels (p = 0.009) (; ).
When completing 0.6 km daily in the heeled conditions, probability of failure with repair was significantly greater in the medium (p = 0.035) and high (p = 0.006) heels compared to the trainer condition. Furthermore, probability of failure with repair was significantly greater in the high compared to low (p = 0.041) and medium heels (p = 0.009) (; ).
Effects of distance
In the high heels, each of the five distance conditions differed significantly from one another (p = 0.004–0.026) and probability of failure with repair increased linearly alongside increases in total distance walked (; ).
In the medium heels, probability of failure with repair was significantly greater in the 3.0 km compared to 2.4 km (p = 0.038), 1.8 km (p = 0.041), 1.2 km (p = 0.044) and 0.6 km (p = 0.046), in the 2.4 km compared to 1.8 km (p = 0.043), 1.2 km (p = 0.046) and 0.6 km (p = 0.049) and also in the 1.8 km compared to 0.6 km (p = 0.049) (; ).
Discussion
The current study aimed to explore the effects of different heeled footwear heights on medial tibiofemoral cartilage mechanics and lifetime failure probability in relation to non-heeled footwear using a combined musculoskeletal simulation and computational modelling approach. This represents the first investigation to examine the effects of high-heeled footwear using a concurrent approach of the aforementioned techniques and may therefore provide more detailed information regarding the effects of high-heeled footwear on knee OA risk.
In agreement with our hypotheses, the current investigation importantly showed using musculoskeletal simulation and contact mechanical modelling that compressive medial tibiofemoral joint forces, stresses and strains were statistically increased in the heeled conditions in comparison to the trainer and also linearly between heel heights. It is proposed that these observations in relation to joint mechanics were mediated by the corresponding increases in quadriceps muscle kinetics, as Sinclair et al. (Citation2021) showed that vastus intermedius, vastus lateralis and vastus medialis muscle forces were the most prominent predictors of medial tibiofemoral loading. This observation supports those using joint moments and indicates that heeled footwear increases the risk of from the mechanical parameters linked to the aetiology of medial knee OA (Simonsen et al., Citation2012; Barkema et al. Citation2012; Kerrigan et al., Citation1998) compared to walking exclusively in non-heeled footwear.
In addition to the aforementioned observations in relation to medial tibiofemoral forces, stresses and strains per footfall, the current investigation also showed that medial compressive tibiofemoral cumulative load was significantly increased in the heeled footwear. Allied to the increased joint loading indices per footfall in the heeled footwear, it appears that the cumulative joint loads were further exacerbated by the statistically reduced stride lengths in the heeled footwear. This importantly meant that a larger number of footfalls are required to complete the same distance, which has clear implications for lifetime cartilage failure probability.
Importantly, in line with our hypotheses, this study showed using probabilistic modelling that at the greatest modelled daily walking distance (i.e. 3.0 km), medial tibiofemoral cartilage failure probability was statistically increased in all of the experimental heeled conditions in comparison to the trainer. Furthermore, at the remaining distances, cartilage failure probability was shown to be statistically greater in the high and medium heels compared to the trainer. Finally, and again in agreement with our hypothesis, the analyses of the experimental footwear conditions showed that at all of the experimentally modelled walking distances, probability of cartilage failure increased linearly and statistically between heel heights. It is noteworthy that the failure probability indices of the heeled footwear were in some cases as much as tenfold larger in relation to the trainer condition. Taking into account the model parameters (Miller & Krupenevich, Citation2020), such increases were mediated as a function of the greater cartilage strains in the heeled footwear conditions. The distance based probabilistic indices importantly showed in the high and medium heels, that cartilage failure probability, decreased linearly alongside reductions in walking distance. Overall, this investigation strongly supports the long-held notion regarding the effects of high-heels on tibiofemoral cartilage health (Linder & Saltzman, Citation1998), as the utilisation of heeled footwear leads to a far greater probability of medial tibiofemoral OA. Tibiofemoral OA is characterised by debilitating and painful presentation (Heidari, Citation2011) as well as long-term fiscal healthcare implications (Martins et al., Citation2021). This investigation therefore strongly advocates that walking distance in heeled footwear as well as the heel height itself be reduced to the greatest extent possible.
That this study utilised a musculoskeletal simulation-based procedure to quantify both muscle and joint kinetics may serve as a limitation to the current investigation. Whilst musculoskeletal simulation is considered to be superior to inverse dynamics driven indices of joint loading, and the simulation models were scaled to account for each participants anthropometrics; there are numerous mechanical assumptions in the creation of musculoskeletal simulation models. These predominately relate to the constrained degrees of freedom within the lower extremity joints, the absence of modelled soft tissues within the joints themselves as well as a lack of surface EMG to corroborate the static optimisation process. A further potential limitation is that medial tibiofemoral contact strains and cartilage-failure probability indices were quantified via generic scaled model parameters rather than a patient-specific imaging-based approach. This drawback is less pronounced considering the within-subject comparisons utilised in this study; but may have a greater impact on between-subject analyses. Whilst there are logistical advantages to this approach to probabilistic modelling, there remains ambiguity in the specificity of cartilage structure, material properties and mechanical responses to imposed strains. Finally, as the main damage parameter into the cartilage failure model is maximum compressive cartilage strain, it does not therefore account for shear strain or quantify loading and failure at bespoke macroscopic cartilage components. The most effective approach by which to address the research aims outlined in this investigation would be to adopt a longitudinal prospective investigation, exploring the effects of high heels over the modelled time course (i.e. 18–60 years). However, prospective experiments on longitudinal and progressive diseases such as OA are rarely undertaken, owing to logistical and fiscal challenges, and future computational and epidemiological analyses should seek to undertake longitudinal model validation from the standpoint of predicting medial knee OA.
In conclusion, though walking biomechanics in heeled footwear has received previous research attention, there has yet to be an exploration of the longitudinal effects of walking in high heels using a cumulative musculoskeletal simulation and probabilistic modelling approach. The present investigation therefore adds to the current clinical knowledge, by examining the effects of different high-heeled footwear on medial tibiofemoral cartilage failure probability. Importantly, compressive medial tibiofemoral cartilage force, stress and strain increased statistically in the heeled conditions in comparison to the trainer and also linearly between heel heights and similarly that cartilage failure probability was statistically increased in the heeled conditions compared to the trainer and also linearly between heel heights. The findings from the current investigation show that high heels of increasing height have a profoundly negative influence on knee cartilage health and strongly advocate that walking distance in heeled footwear and the heel height itself be reduced wherever possible.
Supplemental Material
Download PDF (145.4 KB)References
- Alshami, A. M. (2014). Knee osteoarthritis related pain: A narrative review of diagnosis and treatment. International Journal of Health Sciences, 8(1), 85–104. https://doi.org/10.12816/0006075
- Barkema, D. D., Derrick, T. R., & Martin, P. E. (2012). Heel height affects lower extremity frontal plane joint moments during walking. Gait & Posture, 35(3), 483–488. https://doi.org/10.1016/j.gaitpost.2011.11.013
- Blankevoort, L., Kuiper, J. H., Huiskes, R., & Grootenboer, H. J. (1991). Articular contact in a three-dimensional model of the knee. Journal of Biomechanics, 24(11), 1019–1031. https://doi.org/10.1016/0021-9290(91)90019-j
- Bloecker, K., Guermazi, A., Wirth, W., Benichou, O., Kwoh, C. K., Hunter, D. J., Englund, M., Resch, H., & Eckstein, F. (2013). Tibial coverage, meniscus position, size and damage in knees discordant for joint space narrowing–data from the Osteoarthritis Initiative. Osteoarthritis and Cartilage, 21(3), 419–427. https://doi.org/10.1016/j.joca.2012.11.015
- Cappozzo, A., Catani, F., Della Croce, U., & Leardini, A. (1995). Position and orientation in space of bones during movement: Anatomical frame definition and determination. Clinical Biomechanics, 10(4), 171–178. https://doi.org/10.1016/0268-0033(95)91394-t
- Chen, D. I., Shen, J., Zhao, W., Wang, T., Han, L., Hamilton, J. L., & Im, H. J. (2017). Osteoarthritis: Toward a comprehensive understanding of pathological mechanism. Bone Research, 5(1), 1–13. https://doi.org/10.1038/boneres.2016.44
- Cronin, N. J. (2014). The effects of high heeled shoes on female gait: A review. Journal of Electromyography and Kinesiology, 24(2), 258–263. https://doi.org/10.1016/j.jelekin.2014.01.004
- D'Lima, D. D., Fregly, B. J., Patil, S., Steklov, N., & Colwell, C. W. (2012). Knee joint forces: Prediction, measurement, and significance. Proceedings of the Institution of Mechanical Engineers. Part H, Journal of Engineering in Medicine, 226(2), 95–102. https://doi.org/10.1177/0954411911433372
- Danso, E. K., Mäkelä, J. T. A., Tanska, P., Mononen, M. E., Honkanen, J. T. J., Jurvelin, J. S., Töyräs, J., Julkunen, P., & Korhonen, R. K. (2015). Characterization of site-specific biomechanical properties of human meniscus—Importance of collagen and fluid on mechanical nonlinearities. Journal of Biomechanics, 48(8), 1499–1507. https://doi.org/10.1016/j.jbiomech.2015.01.048
- DeFrate, L. E., Sun, H., Gill, T. J., Rubash, H. E., & Li, G. (2004). In vivo tibiofemoral contact analysis using 3D MRI-based knee models. Journal of Biomechanics, 37(10), 1499–1504. https://doi.org/10.1016/j.jbiomech.2004.01.012
- Delp, S. L., Anderson, F. C., Arnold, A. S., Loan, P., Habib, A., John, C. T., Guendelman, E., & Thelen, D. G. (2007). OpenSim: Open-source software to create and analyze dynamic simulations of movement. IEEE Transactions on Bio-Medical Engineering, 54(11), 1940–1950. https://doi.org/10.1109/TBME.2007.901024
- Eitner, A., Hofmann, G. O., & Schaible, H. G. (2017). Mechanisms of osteoarthritic pain. Studies in humans and experimental models. Frontiers in Molecular Neuroscience, 10, 349. https://doi.org/10.3389/fnmol.2017.00349
- Farrokhi, S., Voycheck, C. A., Tashman, S., & Fitzgerald, G. K. (2013). A biomechanical perspective on physical therapy management of knee osteoarthritis. The Journal of Orthopaedic and Sports Physical Therapy, 43(9), 600–619. https://doi.org/10.2519/jospt.2013.4121
- Graydon, R. W., Fewtrell, D. J., Atkins, S., & Sinclair, J. K. (2015). The test-retest reliability of different ankle joint center location techniques. Foot and Ankle Online Journal, 1(11), 1–11.
- Hame, S. L., & Alexander, R. A. (2013). Knee osteoarthritis in women. Current Reviews in Musculoskeletal Medicine, 6(2), 182–187. https://doi.org/10.1007/s12178-013-9164-0
- Heidari, B. (2011). Knee osteoarthritis prevalence, risk factors, pathogenesis and features: Part I. Caspian Journal of Internal Medicine, 2(2), 205–212.
- Henderson, C. E., Higginson, J. S., & Barrance, P. J. (2011). Comparison of MRI-based estimates of articular cartilage contact area in the tibiofemoral joint. Journal of Biomechanical Engineering, 133(1), 014502. https://doi.org/10.1115/1.4002938
- Herzog, W., Clark, A., & Wu, J. (2003). Resultant and local loading in models of joint disease. Arthritis and Rheumatism, 49(2), 239–247. https://doi.org/10.1002/art.11004
- Hong, W. H., Lee, Y. H., Chen, H. C., Pei, Y. C., & Wu, C. Y. (2005). Influence of heel height and shoe insert on comfort perception and biomechanical performance of young female adults during walking. Foot & Ankle International, 26(12), 1042–1048. https://doi.org/10.1177/107110070502601208
- Johnson, W., Stovitz, S. D., Choh, A. C., Czerwinski, S. A., Towne, B., & Demerath, E. W. (2012). Patterns of linear growth and skeletal maturation from birth to 18 years of age in overweight young adults. International Journal of Obesity, 36(4), 535–541. https://doi.org/10.1038/ijo.2011.238
- Kerrigan, D. C., Todd, M. K., & Riley, P. O. (1998). Knee osteoarthritis and high-heeled shoes. Lancet, 351(9113), 1399–1401. https://doi.org/10.1016/S0140-6736(97)11281-8
- Lerner, Z. F., DeMers, M. S., Delp, S. L., & Browning, R. C. (2015). How tibiofemoral alignment and contact locations affect predictions of medial and lateral tibiofemoral contact forces. Journal of Biomechanics, 48(4), 644–650. https://doi.org/10.1016/j.jbiomech.2014.12.049
- Linder, M., & Saltzman, C. L. (1998). A history of medical scientists on high heels. International Journal of Health Services: Planning, Administration, Evaluation, 28(2), 201–225. https://doi.org/10.2190/GA2M-FLA2-17FB-V5PE
- Liu, F., Kozanek, M., Hosseini, A., Van de Velde, S. K., Gill, T. J., Rubash, H. E., & Li, G. (2010). In vivo tibiofemoral cartilage deformation during the stance phase of gait. Journal of Biomechanics, 43(4), 658–665. https://doi.org/10.1016/j.jbiomech.2009.10.028
- Losina, E., Weinstein, A. M., Reichmann, W. M., Burbine, S. A., Solomon, D. H., Daigle, M. E., Rome, B. N., Chen, S. P., Hunter, D. J., Suter, L. G., Jordan, J. M., & Katz, J. N. (2013). Lifetime risk and age at diagnosis of symptomatic knee osteoarthritis in the US. Arthritis Care & Research, 65(5), 703–711. https://doi.org/10.1002/acr.21898
- Martins, R., Kotsopoulos, N., Kosaner Kließ, M., Beck, C., Abraham, L., Large, S., Schepman, P., & Connolly, M. P. (2021). Comparing the Fiscal Consequences of Controlled and Uncontrolled Osteoarthritis Pain Applying a UK Public Economic Perspective. Journal of Health Economics and Outcomes Research, 8(1), 127–136. https://doi.org/10.36469/001c.24629
- Miller, R. H., & Krupenevich, R. L. (2020). Medial knee cartilage is unlikely to withstand a lifetime of running without positive adaptation: A theoretical biomechanical model of failure phenomena. PeerJ, 8, e9676. https://doi.org/10.7717/peerj.9676
- Nakamura, N., Horibe, S., Toritsuka, Y., Mitsuoka, T., Natsu-Ume, T., Yoneda, K., Hamada, M., Tanaka, Y., Boorman, R. S., Yoshikawa, H., & Shino, K. (2008). The location-specific healing response of damaged articular cartilage after ACL reconstruction: Short-term follow-up. Knee Surgery, Sports Traumatology, Arthroscopy, 16(9), 843–848. https://doi.org/10.1007/s00167-008-0565-3
- Nuño, N., & Ahmed, A. M. (2001). Sagittal profile of the femoral condyles and its application to femorotibial contact analysis. Journal of Biomechanical Engineering, 123(1), 18–26. https://doi.org/10.1115/1.1339819
- Paluch, A. E., Gabriel, K. P., Fulton, J. E., Lewis, C. E., Schreiner, P. J., Sternfeld, B., Sidney, S., Siddique, J., Whitaker, K. M., & Carnethon, M. R. (2021). Steps per day and all-cause mortality in middle-aged adults in the Coronary Artery Risk Development in Young Adults study. JAMA Network Open, 4(9), e2124516. https://doi.org/10.1001/jamanetworkopen.2021.24516
- Riemenschneider, P. E., Rose, M. D., Giordani, M., & McNary, S. M. (2019). Compressive fatigue and endurance of juvenile bovine articular cartilage explants. Journal of Biomechanics, 95, 109304. https://doi.org/10.1016/j.jbiomech.2019.07.048
- Shepherd, D. E., & Seedhom, B. B. (1999). The’instantaneous’ compressive modulus of human articular cartilage in joints of the lower limb. Rheumatology, 38(2), 124–132. https://doi.org/10.1093/rheumatology/38.2.124
- Simonsen, E. B., Svendsen, M. B., Nørreslet, A., Baldvinsson, H. K., Heilskov-Hansen, T., Larsen, P. K., Alkjær, T., & Henriksen, M. (2012). Walking on high heels changes muscle activity and the dynamics of human walking significantly. Journal of Applied Biomechanics, 28(1), 20–28. https://doi.org/10.1123/jab.28.1.20
- Sinclair, J., Brooks, D., & Butters, B. (2019). Effects of different heel heights on lower extremity joint loading in experienced and in-experienced users: A musculoskeletal simulation analysis. Sport Sciences for Health, 15(1), 237–248. https://doi.org/10.1007/s11332-019-00534-4
- Sinclair, J., Brooks, D., Taylor, P. J., & Liles, N. B. (2021). Effects of running in minimal, maximal and traditional running shoes: A musculoskeletal simulation exploration using statistical parametric mapping and Bayesian analyses. Footwear Science, 13(2), 143–156. https://doi.org/10.1080/19424280.2021.1892834
- Sinclair, J., Edmundson, C. J., & Bentley, I. (2022). The efficacy of repetitions-in-reserve vs. traditional percentage-based resistance training: A 4-week pre-season randomized intervention in elite rugby league players. Sport Sciences for Health, 18(2), 525–535. https://doi.org/10.1007/s11332-021-00837-5
- Sinclair, J., Edmundson, C. J., Brooks, D., & Hobbs, S. J. (2011). Evaluation of kinematic methods of identifying gait Events during running. International Journal of Sports Science and Engineering, 5(3), 188–192.
- Sinclair, J., Hebron, J., & Taylor, P. J. (2014). The influence of tester experience on the reliability of 3D kinematic information during running. Gait & Posture, 40(4), 707–711. https://doi.org/10.1016/j.gaitpost.2014.06.004
- Sinclair, J., Hebron, J., & Taylor, P. J. (2015). The test-retest reliability of knee joint center location techniques. Journal of Applied Biomechanics, 31(2), 117–121. https://doi.org/10.1123/jab.2013-0312
- Sinclair, J., Huang, G., Taylor, P. J., Chockalingam, N., & Fan, Y. (2022). Effects of Running in Minimal and Conventional Footwear on Medial Tibiofemoral Cartilage Failure Probability in Habitual and Non-Habitual Users. Journal of Clinical Medicine, 11(24), 7335. https://doi.org/10.3390/jcm11247335
- Sinclair, J., Ingram, J., Taylor, P. J., & Chockalingam, N. (2019). Acute effects of different orthoses on lower extremity kinetics and kinematics during running: A musculoskeletal simulation analysis. Acta of Bioengineering and Biomechanics, 21(4), 13–25. https://doi.org/10.37190/ABB-01405-2019-02
- Sinclair, J., Lynch, H., Chockalingam, N., & Taylor, P. J. (2023). Effects of Obesity on Medial Tibiofemoral Cartilage Mechanics in Females—An Exploration Using Musculoskeletal Simulation and Probabilistic Cartilage Failure Modelling. Life, 13(2), 270. https://doi.org/10.3390/life13020270
- Sinclair, J., Taylor, P. J., Currigan, G., & Hobbs, S. J. (2014). The test-retest reliability of three different hip joint centre location techniques. Movement & Sport Sciences, 83(1), 31–39. https://doi.org/10.3917/sm.083.0031
- Sinclair, J., Taylor, P. J., & Hobbs, S. J. (2013). Digital filtering of three-dimensional lower extremity kinematics: An assessment. Journal of Human Kinetics, 39, 25–36. https://doi.org/10.2478/hukin-2013-0065
- Steele, K. M., DeMers, M. S., Schwartz, M. H., & Delp, S. L. (2012). Compressive tibiofemoral force during crouch gait. Gait & Posture, 35(4), 556–560. https://doi.org/10.1016/j.gaitpost.2011.11.023
- Taylor, D. (1998). Fatigue of bone and bones: An analysis based on stressed volume. Journal of Orthopaedic Research, 16(2), 163–169. https://doi.org/10.1002/jor.1100160203
- Taylor, D., Casolari, E., & Bignardi, C. (2004). Predicting stress fractures using a probabilistic model of damage, repair and adaptation. Journal of Orthopaedic Research, 22(3), 487–494. https://doi.org/10.1016/j.orthres.2003.08.022
- Taylor, D., & Kuiper, J. H. (2001). The prediction of stress fractures using a ‘stressed volume’ concept. Journal of Orthopaedic Research, 19(5), 919–926. https://doi.org/10.1016/S0736-0266(01)00009-2
- Weightman, B. O., Freeman, M. A. R., & Swanson, S. A. V. (1973). Fatigue of articular cartilage. Nature, 244(5414), 303–304. https://doi.org/10.1038/244303a0
- Wise, B. L., Niu, J., Yang, M., Lane, N. E., Harvey, W., Felson, D. T., Hietpas, J., Nevitt, M., Sharma, L., Torner, J., Lewis, C. E., & Zhang, Y. (2012). Patterns of compartment involvement in tibiofemoral osteoarthritis in men and women and in whites and African Americans. Arthritis Care & Research, 64(6), 847–852. https://doi.org/10.1002/acr.21606