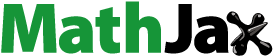
Abstract
The radial flow field structure, which has the advantages of low pressure drop, good water removal and good mass transfer, is an emerging structure for proton exchange membrane fuel cell (PEMFC) flow fields. However, optimization work on the design the structure is scarce for a complex structure that is difficult to manufacture. A comprehensive three-dimensional, non-isothermal and single-phase mathematical model is developed to describe the flow and heat, mass and charge transfer processes in a PEMFC. The transport phenomena and cell performance of radial flow fields with different gradient channels and parallel flow fields are studied and compared using the commercial computational fluid dynamics (CFD) software Fluent®. The distribution of oxygen concentration, pressure drop and temperature with different radial lengths is obtained. The effects of gradient channels, gas supply modes and radial lengths on cell performance are investigated. The results show that radial flow fields could offer more uniform oxygen distributions and lower pressure drops compared with parallel flow fields. Larger gradient channel sizes contribute to larger transfer volumes and higher gas molar concentrations in the catalyst layers and lower pressures in channels. The counter-flow supply mode is superior to the co-flow supply mode because it can enable a higher oxygen velocity and hence a higher current density.
1. Introduction
The proton exchange membrane fuel cell (PEMFC), which has the advantages of low noise, zero emission, and high specific power and current density, cannot only be used in special fields such as aerospace and the military, but also has great market potential in electric vehicles, hybrid locomotives, fuel cell power stations, etc. (Bao et al., Citation2014; Hsieh & Su, Citation2014; Hu et al., Citation2012; Li & Liu, Citation2009). Much research has been focused on the reduction of manufacturing costs and the optimization of PEMFC performance, and great progress has been made in many corresponding fields such as operation conditions optimisation, flow field design, etc. (Akella et al., Citation2018; Li et al., Citation2017; Subramaniam et al., Citation2017).
A superior flow field structure is particularly important in fuel cells for reducing the pressure drop while providing proper and uniformly distributed mass transport in the catalyst layer (CL). Conventional flow fields, such as parallel, interdigitated, metal foam and some bionic flow fields, have been investigated widely and in depth. Although the pressure drop is reduced in parallel flow fields, the provision of reactants and water removal predominately rely on diffusion, and the performance decreases dramatically as the current density increases (Atyabi & Afshari, Citation2019; Lim et al., Citation2020). The advantage of interdigital flow fields is that gases are transported mainly by the convection mechanism, which is faster than the diffusion mechanism (Nguyen, Citation1996). Furthermore, the reactant utilization and water removal rate could be enhanced for faster convection in the interdigital one, and the reaction rate in the CL could be greatly improved (Wang et al., Citation2011). A serpentine flow field has a performance in a PEMFC superior to that of a parallel one. However, it also has a higher pressure drop when the distance from inlet to outlet is long with a single flow path, so the reactant gas distribution also appears non-uniform (Zhang et al., Citation2019). The friction of walls and turning channels would result in a higher pressure drop, which could effectively enhance the removal of accumulated water from the channels (Lakshminarayanan & Karthikeyan, Citation2019). The distribution of the reactant gases is relatively even because of a low pressure drop in metal foam flow fields for its high permeability and foam structure (Huo et al., Citation2021). But the disadvantage of metal foam flow fields is weak convection in corners (Afshari, Citation2020). The bionic flow field is designed to improve water balance and fuel distribution uniformity, and the mechanism comes from the biological properties of nature (Iranzo et al., Citation2020). However, less attention has been paid to this flow field due to high material cost and the complexity of manufacturing (Arvay et al., Citation2013).
In the work of Cano-Andrade et al. (Citation2010), the effect of an innovative radial flow field design for bipolar plates was studied, with four, eight and twelve channels, respectively. It was indicated that this radical model produced a current density about six times larger than that of the conventional serpentine configuration. Subsequent studies showed that a radial flow field had better water removal ability and mass transfer than the traditional models (Friess & Hoorfar, Citation2012). This kind of flow field has been researched more and more for its good characteristics, such as low pressure drop and good mass transfer (Solati et al., Citation2019).
A gradient channel whose width or height is not constant but varies in gradient has also been a popular research subject for flow field structure. Wang et al. (Citation2009) found that sub-rib convection could be enhanced significantly by reducing the channel height gradually. A gradient channel with different width could enhance the velocity, which was instructive for removing water, while the under-rib convection between adjacent channels was also enhanced (Wang et al., Citation2017). It was reported that the flow channels with gradient slope could enhance the gas flow rate and promote the gas to enter the reaction zone (Ahmadi et al., Citation2018). Korkischko et al. (Citation2017) invented a channel with trapezoidal cross section, which was compared with a rectangular flow channel. The results showed that current generation was improved; simultaneously, higher and more uniform current density was obtained. Subsequently, convergent and divergent channels were studied and it was shown that convergent ones had good mass transport, water removal characteristics and uniformity of current density (Chowdhury & Timurkutluk, Citation2018; Timurkutluk & Chowdhury, Citation2018).
In a study of gas supply modes, Kim (Citation2008) found that the co-flow mode could achieve higher current density than the counter-flow mode. However, the opposite conclusion was obtained by Sierra et al. (Citation2011). In addition, the counter-flow mode was better in keeping an even water content distribution to avoid over-drying of the membrane and to increase species permeability for well-distributed reacting in the cell (P. Xu & S.C. Xu, Citation2017; Yuan et al., Citation2021). Furthermore, different cooling patterns were explored (Liu et al., Citation2020). The counter-flow mode, with the cooling water flowing along with cathode gas in the same direction, was proved to be better.
Because of the lower pressure drop and shorter mass transfer distance, a radial flow field with a gradient channel is a strong candidate for the development of fuel cell technology in the near future. In this work, the effect of gradient channels and radial lengths is studied to optimize the structure of radial models. Owing to the geometry of the configuration and to save time and computational resources, a quarter cut of the overall cell is analyzed by computational fluid dynamics (CFD) technology. The meshing method is improved to avoid the generation of triangular grids, which enhances the calculation convergence and accuracy in the simulation procedure. A more uniform oxygen distribution and a lower pressure drop are found in the flow channels of radial flow fields. It is proved that radial models have a significant advantage in transport, comparing with parallel flow fields. Additionally, the varieties of oxygen concentration, pressure drop, temperature, current density and water distribution in the membrane are discussed. The effect of gas supply mode on the flow velocity distribution of hydrogen and oxygen is studied for further optimization of the working conditions of radial models.
2. Model development
2.1. Geometric models
Schematic model diagrams of a parallel flow field and three radial flow fields with an electrode area of 2500 mm2 are shown in Figure . The depth and width of the channels in the parallel flow field are 1 mm. The radial flow fields have the same inlet channel width, i.e. 1.0 mm, and different outlet widths of 1.5, 2.0 and 2.5 mm, respectively, marked R1, R2 and R3.
Figure 1. Schematic diagrams of parallel flow field and radial flow fields with gradient flow channels.

Two gas supply modes, co-flow and counter-flow, are plotted in Figure . For the co-flow mode, both hydrogen and oxygen are supplied from inside to outside, as shown in Figure (a). For the counter-flow mode, hydrogen is fed from inside to outside and oxygen is fed from outside to inside, as shown in Figure (b).
Figure 2. Two methods of gas supply for a radial flow field: (a) co-flow mode; (b) counter-flow mode.

Radial flow fields with the same electrode area, i.e. 2500 mm2, and different radial lengths of 33.9, 36.9, 39.9 and 42.9 mm, respectively, are shown in Figure , marked R4, R5, R6 and R7.
2.2. Control equations
In order to develop a mathematical model for the PEMFC, the following assumptions are made.
The fuel cell works under steady state.
The liquid water produced is neglected.
The fluid is an ideal gas mixture.
The fluid flow is laminar for the low Renolds number.
The weight of the gas is not considered.
Porous media such as the gas diffusion layer (GDL) and the CL are both considered isotropic and uniform.
The general mass conservation equation is written as follows:
(1)
(1) where
is the porosity;
is the gas velocity;
is the gas density; and
is the quality source item.
The conservation of momentum equation is written as follows:
(2)
(2) where
is the dynamic viscosity, p stands for the reaction gas pressure; and
is the source term of momentum.
The energy conservation is written as follows:
(3)
(3) where
is the specific heat capacity of the mixture;
is the effective thermal conductivity; and
is the energy source term.
The species conservation equation is written as follows:
(4)
(4) where
is the species concentration;
is the effective diffusion coefficient; and
is the species source item.
The charge conservation equations for electron and ion transport are described as follows:
(5)
(5)
(6)
(6) where
is the effective electric conductivity in the solid phase;
is the effective ionic conductivity of the membrane phase;
is the solid-phase potential;
is the membrane-phase potential; and
is the volume source term of charge.
2.3. Numerical procedure and simulation parameters
The numerical solutions of the continuity and momentum equations as well as the heat transfer and charge transfer equations are carried out using the commercial software ANSYS®–FLUENT® 2021R1. The coupled velocity equations and pressure equation are solved using semi-implicit method for pressure-linked equations consistent (SIMPLEC) algorithm. The current density is obtained for a given voltage from 1.1 to 0.4 V in steps of 0.1 V.
The thickness of the proton exchange membrane, the CL, the GDL and the current collector are 0.04, 0.01, 0.25 and 1.75 mm, respectively.
Setting the operating and physical parameters directly affects the correctness of the simulation results. Some main simulation parameters are shown in Table for solving the model equations such as mass, momentum, energy, component and charge conservation.
Table 1. Operating cases and physical parameters.
3. Model validation
3.1. Grid reliability verification
The PEMFC with a radial flow field is meshed by a hexahedral grid, and different numbers, i.e. 595,200, 952,320, 1,904,640 and 3,809,280, are applied to verify the reliability of the grid. Their current densities operating at 0.4 V are listed in Figure . If the grid number is equal to or above 1,904,640, the current density change is in the range 0.06–1.02%. Thus, 1,904,640 grids are chosen in this study considering the balance of computation time and accuracy.
3.2. Experimental verification
The simulation results of the parallel design are compared with two recent experimental results (Ruan et al., Citation2016; Wang et al., Citation2020), as illustrated in Figure . The results illustrate that the predicted performance agrees well with the experimental data, which proves the high reliability of the numerical model and simulation results. Verification of the simulation results with radial flow fields is not conducted because experimental data, though rare, can be found in the published literature.
4. Results and discussion
4.1. Effect of gradient channels
Figure shows the polarization performance of PEMFCs with a parallel flow field and three radial flow fields working in the co-flow mode. At low current density, the activation polarization is the main factor of voltage loss, and the flow field structure has less influence on the polarization curves. However, both electrical resistance and mass transfer resistance cause voltage loss at high current density. The main purpose of flow field structure research is to enhance mass transfer and reduce voltage loss. It can be seen in Figure that the peak power density in the parallel case is 0.633 W cm−2. Among the PEMFCs with radial flow fields, the peak value is obtained by R3, i.e. 0.676 W cm−2, followed by R2 and R1. The value of 6.7% is improved by applying a radial flow field with a 2.5 mm outlet flow channel. The performance of R2 and R3 are relatively close, indicating that less effect is found as the size of the gradient channel continues increasing. This result is consistent with what Perng and Wu (Citation2011) obtained. It may be due to higher local current density in the CL because of larger flow channel size.
The molar concentration of oxygen in the cathodic CL is studied for further understanding of the performance of the four flow fields. In Figure , the decrease of gas concentration along the transport direction is caused by continuous consumption of reactants. The average concentrations in the cathodic CL are 1.28, 1.23, 1.43 and 1.66 mol m−3, respectively, as shown in Figures (a)–(d). A larger outlet width results in a higher gas concentration, which promotes the electrochemical reaction and contributes to the improvement of cell performance (Ahmadi et al., Citation2018; Timurkutluk & Chowdhury, Citation2018). The parallel flow field has a low-oxygen region, which indicates high non-uniformity causing bad current distribution and therefore low durability (Zhong et al., Citation2018).
Figure 7 Oxygen molar concentration distribution in the cathodic CL of PEMFCs with four different flow fields at 0.5 V.

The pressure drops in the flow channels are shown in Figure . At the anode, the values in Figures (a)–(d) are 55.2, 4.9, 3.4 and 2.8 Pa, respectively. The values at the cathode in Figures (e)–(h) are 1970.0, 32.9, 24.4 and 19.8 Pa, which are several times those at the anode. Lower pressure drops are obtained with radial flow fields, especially at the cathode, which indicates the superiority of the radial flow fields. Furthermore, the pressure drops in the radial flow fields decrease at both sides as the outlet width increases, which may be due to the larger mass transfer volume in R3 (Fontana et al., Citation2011).
Figure 8. Pressure drop at the anode (a)–(d) and cathode (e)–(h) of PEMFCs with four different flow fields at 0.5 V.

As the outlet size of the flow channel increases, the contact area between the flow channel and the diffusion layer increases. The contact areas of R1, R2 and R3 iare 748, 898 and 1047 cm2, respectively. The higher area of R3 is suitable for the reactants to enter the diffusion layer and react in the CL (Zhang et al., Citation2018).
4.2. Effect of gas supply modes
The effect of flow modes is studied in R3, with an outlet width of 2.5 mm. The polarization curves for co-flow and counter-flow modes are shown in Figure , with peak power densities of 0.633 and 0.707 W cm−2, respectively. It is shown that the performance of radial flow fields can be enhanced by counter-flow modes compared with co-flow ones. The counter-flow mode is also preferred in industry to avoid the mixing of gas reactants in radial flow fields.
The velocity distributions of hydrogen and oxygen under two supply modes at 0.5 V is shown in Figure . The maximum velocity appears in the narrow part of channels, which can be explained by Bernoulli’s equation (Choi & Kim, Citation2014). For a given flow rate, the flow velocity is inversely proportional to the square of the channel width. Under a co-flow mode, the velocities at the anode and cathode are 1.31 and 4.33 m s−1, respectively, while the hydrogen transport direction at the anode is the same for both modes—there is no difference in the velocity of hydrogen between Figures (a) and (c). In the counter-flow mode, the oxygen at the cathode has a velocity of 5.08 m s−1. In this situation, oxygen is forced to enter the GDL while passing through the tapering flow channel, therefore the mass transfer is enhanced (Zehtabiyan-Rezaie et al., Citation2017).
4.3. Effect of radial length
Four radial flow fields with diverse radial lengths are designed and their polarization curves are obtained in counter-flow mode, as shown in Figure . The peak power densities with flow fields of R4, R5, R6 and R7 are 0.948, 0.854, 0.707 and 0.648 W cm−2, respectively. It is shown that a decrease in radial length brings higher cell performance, which is probably related to the larger contact area of the R4 flow field. The contact areas of R4, R5, R6 and R7 are 1367, 1228, 1047 and 900 cm2, respectively.
The effect of radial length on the molar concentration of oxygen in the cathodic CL is shown in Figure . The average concentrations in Figures (a)–(d) are 1.57, 1.56, 1.53 and 1.33 mol m−3, respectively. The value gradually decreases as the length increases. More reactive gases in Figure (a) participate in the electrochemical reaction, which has an enhancing effect on the cell performance.
Figure 12 Oxygen molar concentration distributions in the cathodic CLs of PEMFCs with radial flow fields having different radial lengths under 0.5 V.

The effect of radial length on the pressure drop in the cathodic channels is shown in Figure . The values in Figures (a)–(d) are 14.7, 19.4, 28.8 and 39.6 Pa, respectively. Since the total mass flow is fixed, the flow rate in each channel decreases with the number of channels. More channels with shorter radial lengths can bring lower pressure drops.
Figure 13. Pressure drop distributions in the channels of the cathodes of PEMFCs with radial flow fields having different radial lengths.

In fuel cells, the temperature at the anode side is always lower than that at the cathode side due to the heat generated by electrochemical reaction and inner resistance (Hashemi et al., Citation2012). Therefore, the temperature in the cathodic CL is chosen to study the effect of length. As shown in Figure , the average temperatures are 356.1, 355.7, 355.2 and 355.0 K, respectively. The highest temperature points are concentrated at the oxygen inlet and the outside region of the radial flow field. More red points are depicted in Figure (a), which indicates that a high current density is produced.
Figure 14. Temperature distribution in the cathodic CLs of PEMFCs with radial flow fields having different radial lengths.

The amount of water in the membrane is essential for the cell performance, which is necessary for the transfer of protons through the membrane (Velisala & Srinivasulu, Citation2018). The water distribution in the membrane of fuel cells with radial flow fields of different radial length is shown in Figure . A relatively more even water distribution is found in Figures (a) and (b).
Figure 15 Water content distribution in the membranes of PEMFCs with radial flow fields having different radial lengths.

The current density in the cathodic CL is shown in Figure . The current density reduces along the flow direction from outside to inside owing to the decreasing oxygen concentration along the flow channel. Lower current density can be found at the edge for the lack of reaction gas (Velisala et al., Citation2021). The average current densities of fuel cells with radial flow fields and different radial lengths (Figures (a)–(d)) are 3.2, 2.9, 2.4 and 2.0 A cm−2, respectively. The current density in the cathodic CL also decreases as the radial length increases, which is bound up with the changes of oxygen concentration.
5. Conclusions
A PEMFC model with radial flow fields is developed by CFD technology in order to study mass transport characteristics and polarization performance. The influences of gradient channels, gas supply modes and radial length have been investigated. The main conclusions can be obtained as follows.
Firstly, compared with a parallel flow field, radial flow fields have a significant advantage in transport and can offer more uniform oxygen distributions and lower pressure drops in flow channels.
Secondly, the counter-flow gas supply mode increases the oxygen velocity at the cathode and thus improves cell performance.
In this work, the single-phase mode is used for the estimation of flow field performance, while liquid water generated under saturated conditions is not considered. Therefore, a two-phase flow model would be highly recommended for the study of water transfer in future work. Otherwise, owing to the superior performance of radial flow fields, PEMFC stacks designed with circular MEA (Membrane Electrode Assembly)s would be an interesting topic, especially the experimental investigation of fuel cell performance.
Disclosure statement
No potential conflict of interest was reported by the authors.
Data availability statement
Fuel cell dimensions, some of the physical parameters and basic operation case conditions used in the single cell simulation are listed in table 1. If required, we can provide the detailed information and other parameters used in the model. The solution of model equaitons is by Ansys-FLUENT. There is no other associated data.
References
- Afshari, E. (2020). Computational analysis of heat transfer in a PEM fuel cell with metal foam as a flow field. Journal of Thermal Analysis and Calorimetry, 139(4), 2423–2434. https://doi.org/10.1007/s10973-019-08354-x
- Ahmadi, N., Rezazadeh, S., Dadvand, A., & Mirzaee, I. (2018). Study of the effect of Gas channels geometry on the performance of polymer electrolyte membrane fuel cell. Periodica Polytechnica-Chemical Engineering, 62(1), 97–105. https://doi.org/10.3311/PPch.9369
- Akella, H., Ebenezer, D., Siddhardha, Sai, R. S., Ahire, A., & Mal, N. K. (2018). Studies on structure property relations of efficient decal substrates for industrial grade membrane electrode assembly development in PEMFC. Scientific Reports, 8. https://doi.org/10.1038/s41598-018-30215-0
- Arvay, A., French, J., Wang, J. C., Peng, X. H., & Kannan, A. M. (2013). Nature inspired flow field designs for proton exchange membrane fuel cell. International Journal of Hydrogen Energy, 38(9), 3717–3726. https://doi.org/10.1016/j.ijhydene.2012.12.149
- Atyabi, S. A., & Afshari, E. (2019). A numerical multiphase CFD simulation for PEMFC with parallel sinusoidal flow fields. Journal of Thermal Analysis and Calorimetry, 135(3), 1823–1833. https://doi.org/10.1007/s10973-018-7270-3
- Bao, N., Zhou, Y. B., Zhou, Y. B., Yin, Y., Du, Q., & Chen, J. X. (2014). Effect of gas diffusion layer deformation on liquid water transport in proton exchange membrane fuel cell. Engineering Applications of Computational Fluid Mechanics, 8(1), 26–43. https://doi.org/10.1080/19942060.2014.11015495
- Cano-Andrade, S., Hernandez-Guerrero, A., von Spakovsky, M. R., Damian-Ascencio, C. E., & Rubio-Arana, J. C. (2010). Current density and polarization curves for radial flow field patterns applied to PEMFCs (proton exchange membrane fuel cells). Energy, 35(2), 920–927. https://doi.org/10.1016/j.energy.2009.07.045
- Choi, J., & Kim, Y. H. (2014). A note on high order Bernoulli numbers and polynomials using differential equations. Applied Mathematics and Computation, 249, 480–486. https://doi.org/10.1016/j.amc.2014.10.074
- Chowdhury, M. Z., & Timurkutluk, B. (2018). Transport phenomena of convergent and divergent serpentine flow fields for PEMFC. Energy, 161, 104–117. https://doi.org/10.1016/j.energy.2018.07.143
- Fontana, E., Mancusi, E., da Silva, A., Mariani, V. C., de Souza, A., & de Souza, S. (2011). Study of the effects of flow channel with non-uniform cross-sectional area on PEMFC species and heat transfer. International Journal of Heat and Mass Transfer, 54(21-22), 4462–4472. https://doi.org/10.1016/j.ijheatmasstransfer.2011.06.037
- Friess, B. R., & Hoorfar, M. (2012). Development of a novel radial cathode flow field for PEMFC. International Journal of Hydrogen Energy, 37(9), 7719–7729. https://doi.org/10.1016/j.ijhydene.2012.02.012
- Hashemi, F., Rowshanzamir, S., & Rezakazemi, M. (2012). CFD simulation of PEM fuel cell performance: Effect of straight and serpentine flow fields. Mathematical and Computer Modelling, 55(3–4), 1540–1557. https://doi.org/10.1016/j.mcm.2011.10.047
- Hsieh, S. S., & Su, Y. W. (2014). Effects of anode and cathode perforated flow field plates on proton exchange membrane fuel cell performance. International Journal of Energy Research, 38(7), 944–953. https://doi.org/10.1002/er.3098
- Hu, G. L., Neagu, R., Wang, Q. P., Zhang, Z. G., Li, G. N., & Zheng, Y. Q. (2012). Mathematical modelling of flow and heat/mass transfer during reactive spraying deposition technology (RSDT) process for high temperature fuel cells. Engineering Applications of Computational Fluid Mechanics, 6(1), 134–143. https://doi.org/10.1080/19942060.2012.11015409
- Huo, S., Shi, W. Y., Wang, R. F., Lu, B. B., Wang, Y., Jiao, K., & Hou, Z. J. (2021). Elucidating the operating behavior of PEM fuel cell with nickel foam as cathode flow field. Science China Technological Sciences, 64(5), 1041–1056. https://doi.org/10.1007/s11431-020-1767-5
- Iranzo, A., Arredondo, C. H., & Rosa, F. (2020). Biomimetic flow fields for proton exchange membrane fuel cells: A review of design trends. Energy, 190, 116435. https://doi.org/10.1016/j.energy.2019.116435
- Kim, H. G. (2008). Investigation of gas flow characteristics in proton exchange membrane fuel cell. Journal of Mechanical Science and Technology, 22(8), 1561–1567. https://doi.org/10.1007/s12206-008-0318-8
- Korkischko, I., Carmo, B. S., & Carmo, B. S. (2017). Shape optimization of PEMFC flow-channel cross-sections. Fuel Cells, 17(6), 809–815. https://doi.org/10.1002/fuce.201700168
- Lakshminarayanan, V., & Karthikeyan, P. (2019). Investigation of PEMFC performance with various configurations of serpentine and interdigitated flow channel. Progress in Computational Fluid Dynamics, An International Journal, 19(5), 328–336. https://doi.org/10.1504/PCFD.2019.102039
- Li, C. Y., & Liu, G. P. (2009). Optimal fuzzy power control and management of fuel cell/battery hybrid vehicles. Journal of Power Sources, 192(2), 525–533. https://doi.org/10.1016/j.jpowsour.2009.03.007
- Li, Y., Yang, J., & Song, J. (2017). Structure models and nano energy system design for proton exchange membrane fuel cells in electric energy vehicles. Renewable and Sustainable Energy Reviews, 67, 160–172. https://doi.org/10.1016/j.rser.2016.09.030
- Lim, B. H., Majlan, E. H., Daud, W. R. W., Rosli, M. I., & Husaini, T. (2020). Numerical investigation of the effect of three-dimensional modified parallel flow field designs on proton exchange membrane fuel cell performance. Chemical Engineering Science, 217, 115499. https://doi.org/10.1016/j.ces.2020.115499
- Liu, H. J., Zhang, G. D., Li, D., Wang, C. K., Bai, S. Z., Li, G. X., & Wang, G. H. (2020). Three-dimensional multi-phase simulation of cooling patterns for proton exchange membrane fuel cell based on a modified Bruggeman equation. Applied Thermal Engineering, 174, 115313. https://doi.org/10.1016/j.applthermaleng.2020.115313
- Nguyen, T. V. (1996). A Gas distributor design for proton-exchange-membrane fuel cells. Journal of the Electrochemical Society, 143(5), L103–L105. https://doi.org/10.1149/1.1836666
- Perng, S. W., & Wu, H. W. (2011). Non-isothermal transport phenomenon and cell performance of a cathodic PEM fuel cell with a baffle plate in a tapered channel. Applied Energy, 88(1), 52–67. https://doi.org/10.1016/j.apenergy.2010.07.006
- Ruan, H., Wu, C., Liu, S., & Chen, T. (2016). Design and simulation of novel flow field plate geometry for proton exchange membrane fuel cells. Heat and Mass Transfer, 52(10), 2167–2176. https://doi.org/10.1007/s00231-015-1737-6
- Sierra, J. M., Moreira, J., & Sebastian, P. J. (2011). Numerical analysis of the effect of different gas feeding modes in a proton exchange membrane fuel cell with serpentine flow-field. Journal of Power Sources, 196(11), 5070–5076. https://doi.org/10.1016/j.jpowsour.2011.01.079
- Solati, A., Nasiri, B., Mohammadi-Ahmar, A., Mohammadi, K., & Safari, A. H. (2019). Numerical investigation of the effect of different layers configurations on the performance of radial PEM fuel cells. Renewable Energy, 143, 1877–1889. https://doi.org/10.1016/j.renene.2019.06.003
- Subramaniam, S., Rajaram, G., Palaniswamy, K., & Jothi, V. R. (2017). Comparison of perforated and serpentine flow fields on the performance of proton exchange membrane fuel cell. Journal of the Energy Institute, 90(3), 363–371. https://doi.org/10.1016/j.joei.2016.04.006
- Timurkutluk, B., & Chowdhury, M. Z. (2018). Numerical investigation of convergent and divergent parallel flow fields for PEMFCs. Fuel Cells, 18(4), 441–448. https://doi.org/10.1002/fuce.201800029
- Velisala, V., Pullagura, G., Yarramsetty, N., Vadapalli, S., Boni, M. K., & Gorantla, K. K. (2021). Three-dimensional CFD modeling of serpentine flow field configurations for PEM fuel cell performance. Arabian Journal for Science and Engineering, 46(12), 11687–11700. https://doi.org/10.1007/s13369-021-05544-4
- Velisala, V., & Srinivasulu, G. N. (2018). Numerical simulation and experimental comparison of single, double and triple serpentine flow channel configuration on performance of a PEM fuel cell. Arabian Journal for Science and Engineering, 43(3), 1225–1234. https://doi.org/10.1007/s13369-017-2813-7
- Wang, C., Zhang, Q. L., Lu, J. B., Shen, S. Y., Yan, X. H., Zhu, F. J., Cheng, X. J., & Zhang, J. L. (2017). Effect of height/width-tapered flow fields on the cell performance of polymer electrolyte membrane fuel cells. International Journal of Hydrogen Energy, 42(36), 23107–23117. https://doi.org/10.1016/j.ijhydene.2017.07.136
- Wang, X. D., Huang, Y. X., Cheng, C. H., Jang, J. Y., Lee, D. J., Yan, W. M., & Su, A. (2009). Flow field optimization for proton exchange membrane fuel cells with varying channel heights and widths. Electrochimica Acta, 54(23), 5522–5530. https://doi.org/10.1016/j.electacta.2009.04.051
- Wang, X. D., Xu, J. L., Yan, W. M., Lee, D. J., & Su, A. (2011). Transient response of PEM fuel cells with parallel and interdigitated flow field designs. International Journal of Heat and Mass Transfer, 54(11–12), 2375–2386. https://doi.org/10.1016/j.ijheatmasstransfer.2011.02.024
- Wang, Y. L., Wang, S. X., Liu, S. C., Li, H., & Zhu, K. (2020). Optimization of reactants relative humidity for high performance of polymer electrolyte membrane fuel cells with co-flow and counter-flow configurations. Energy Conversion and Management, 205, 112369. https://doi.org/10.1016/j.enconman.2019.112369
- Xu, P., & Xu, S. C. (2017). Three-dimensional modeling of gas purge in a polymer electrolyte membrane fuel cell with co-flow and counter-flow pattern. Fuel Cells, 17(6), 794–808. https://doi.org/10.1002/fuce.201700101
- Yuan, L., Jin, Z. L., Yang, P. H., Yang, Y. C., Wang, D. B., & Chen, X. T. (2021). Numerical analysis of the influence of different flow patterns on power and reactant transmission in tubular-shaped PEMFC. Energies, 14(8). https://doi.org/10.3390/en14082127
- Zehtabiyan-Rezaie, N., Arefian, A., Kermani, M. J., Noughabi, A. K., & Abdollahzadeh, M. (2017). Effect of flow field with converging and diverging channels on proton exchange membrane fuel cell performance. Energy Conversion and Management, 152, 31–44. https://doi.org/10.1016/j.enconman.2017.09.009
- Zhang, G. B., Xie, B., Bao, Z. M., Niu, Z. Q., & Jiao, K. (2018). Multi-phase simulation of proton exchange membrane fuel cell with 3D fine mesh flow field. International Journal of Energy Research, 42(15), 4697–4709. https://doi.org/10.1002/er.4215
- Zhang, G. B., Xie, X., Xie, B. A., Du, Q., & Jiao, K. (2019). Large-scale multi-phase simulation of proton exchange membrane fuel cell. International Journal of Heat and Mass Transfer, 130, 555–563. https://doi.org/10.1016/j.ijheatmasstransfer.2018.10.122
- Zhong, D., Lin, R., Liu, D. C., & Cai, X. (2018). Structure optimization of anode parallel flow field for local starvation of proton exchange membrane fuel cell. Journal of Power Sources, 403, 1–10. https://doi.org/10.1016/j.jpowsour.2018.09.067