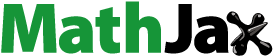
Abstract
Recent technological advances have made it possible to produce particles with nanometer dimensions that are uniformly and steadily suspended in traditional solar liquids and have enhanced the impact of thermo-physical parameters. In this research, a three-dimensional flat plate solar collector was built using a thin flat plate and a single working fluid pipe. The physical model was solved computationally under conditions of conjugated laminar forced convection in the range 500 ≤ Re ≤ 1900 and a heat flux of 1000 W/m2. Distilled water (DW) and different types of hybrid nanofluids (namely, 0.1%-Al2O3@Cu/DW, 0.1%-MWCNTs@Fe3O4/DW, 0.3%-MWCNTs@Fe3O4/DW, 0.5%-Ag@MgO/DW, 1%-Ag@MgO/DW, 1%-S1 and 1%-S2, where MWCNTs are multi-wall carbon nanotubes, S1 means 2CuO–1Cu and S2 means 1CuO–2Cu nanocomposites) were evaluated via a set of parameters. The numerical results revealed that, by increasing the working fluid velocity (the Reynolds number), the average heat transfer coefficient, pressure loss, heat gain and solar collector efficiency were increased. Meanwhile, outlet fluid temperature and flat plate surface temperature were decreased. At Re = 1900, 1%-S2 and 1%-S1 presented higher thermal performance enhancement by 44.28% and 36.72% relative to DW. Moreover, low thermal performance enhancement of 7.59% and 7.44% were reported by 0.1%-Al2O3@Cu/DW and 0.3%-MWCNTs@Fe3O4/DW, respectively.
1. Introduction
1.1. Research background and motivation
The flat plate solar collector (FPSC) is one of the most important solar energy applications for both household and industrial use (Alawi, Kamar, Mallah, Mohammed, Kazi, et al., Citation2021; Alawi, Kamar, Mallah, Mohammed, Sabrudin, et al., Citation2021). The thermal efficiency of FPSCs is lower than that of other types of collector (Akram et al., Citation2020; Alawi et al., Citation2022). The thermo-physical properties of heat transfer fluids are also critical in affecting the thermal efficiency of solar collectors (Mortazavinejad & Mozafarifard, Citation2019; Singh et al., Citation2018; Visa et al., Citation2019; Zhou et al., Citation2019). Experimental, numerical and theoretical studies have used nanofluids as heat transfer fluids (HTFs) inside FPSCs as alternatives to base fluid because of their advanced thermo-physical properties (Bezaatpour & Rostamzadeh, Citation2021; Sheikholeslami et al., Citation2021; Zayed et al., Citation2019). Hybrid nanofluids consist of more than two nanomaterials suspended in the base fluids in order to enhance thermal performance in a variety of applications (Mackolil & Mahanthesh, Citation2021; Mahanthesh, Citation2021a, Citation2021b; Thriveni & Mahanthesh, Citation2021). Experimental and numerical studies have proven that nanocomposite nanofluids have a higher heat transfer efficiency than base fluids (Maleki et al., Citation2021; Tiwar et al., Citation2021).
1.2. Adopted literature review on nanofluids-based FPSCs
According to several earlier research works (Shah & Ali, Citation2019), hybrid nanofluids are more efficient than single nanofluids in terms of thermo-physical properties. Two single nanofluids (0.1 wt.%-Al2O3-H2O with 20 nm and 0.1 wt.%-TiO2-H2O with 15 nm) and a hybrid nanofluid (Al2O3@TiO2-H2O) were evaluated in experiments and numerical investigations as HTFs inside a flat plate solar collector by Farajzadeh et al. (Citation2018). The surfactant cetyltrimethylammonium bromide (CTAB) was added to the mixture to accelerate the chemical reaction, and three volume flow rates, 1.5, 2.0 and 2.5 LPM, were trialled. Their results showed that the overall FPSC thermal efficiency was enhanced by 19%, 21% and 26% when using 0.1 wt.%-Al2O3/H2O, 0.1 wt.%-TiO2/H2O and Al2O3@TiO2/H2O, respectively. In another study (Ranga Babu et al., Citation2018), the entropy generation of Cu@CuO/H2O, Cu/H2O and CuO/H2O nanofluids was reduced by 3.31%, 1.35% and 2.96%, respectively. Furthermore, using the hybrid nanofluid (Cu@CuO/H2O) increased the exergy efficiency by 2.59%. Two hybrid nanofluids, 80%-MgO@20%-MWCNTs and 80%-CuO@20%-MWCNTs, were compared in terms of energy performance by Verma et al. (Citation2018). The nanoparticle concentration was in the range 0.25–2.0% with volume flow rates of 0.5–2.0 LPM. The application of MgO@MWCNTs/H2O and CuO@MWCNTs/H2O inside the FPSC enhanced the thermal performance by 18.05% and 20.52%, respectively, relative to H2O. Hybrid nanofluids containing three nanocomposites, such as CF-MWCNTs@CF-GNPs with h-BN, were suspended in H2O in the presence of Tween-80 by Hussein et al. (Citation2020). In their study, the solar collector efficiency reached up to a value of 85% at 4 LPM. The application of 0.1 wt.%-MWCNTs@GNPs/h-BN increased the heat gain parameter and heat loss parameter up to 21.9% and 78.3%, respectively. Nano-diamond–cobalt oxide (ND@Co3O4) hybrid nanofluids in different concentrations of 0.05–0.15 wt.% were studied at various volume flow rates in the range 0.56–1.35 LPM by Sundar et al. (Citation2021). The thermal conductivity and dynamic viscosity of 0.15 wt.%-ND@Co3O4-H2O were increased by 15.71% and 45.83% at an inlet temperature of 60°C. Moreover, the employment of 0.15 wt.%-ND@Co3O4-H2O increased the average Nusselt number (Nuavg) by 21.23% with a maximum friction factor ( f ) penalty of 1.13 times that of the base fluid. The solar collector efficiency of 0.15 wt.%-ND@Co3O4-H2O was found to be 59%, while it was 48% for H2O. Okonkwo et al. (Citation2020) proved that a single nanofluid (0.1%-Al2O3-H2O) was more efficient than a hybrid nanofluid (0.1%-Al2O3@Fe-H2O) with an enhancement in the thermal performance by 2.16% and 1.79%, respectively, relative to the base fluid. A considerable increase in the dynamic viscosity of the hybrid nanofluid compared with the single nanofluid caused this phenomenon. On the other hand, 0.1%-Al2O3@Fe-H2O enhanced the exergy efficiency by 6.9% as against 5.7% using 0.1%-Al2O3-H2O. The combination of using a porous medium and Ag/Al2O3-H2O hybrid nanofluids was solved computationally in the presence of thermal radiation by Xiong et al. (Citation2021). According to their study, at a given Reynold number the heat transfer was found to be increased substantially by increasing the porosity coefficient and decreasing the thermal radiation parameter, the Darcy number and the thermal conductivity ratio. Different concentrations of hybrid CuO-Cu/water nanofluid were prepared to study its influence on a solar thermal energy storage system by Alrowaili et al. (Citation2022). The collection area was lowered by up to 38% by using a hybrid nanofluid. The calculated heat removal factor was 0.894. Hybrid CuO 2.5 g + Cu 1.5 g nanofluid outperformed water and mono CuO in terms of thermal-optical efficiency by 61.7% and 14.9%, respectively. The results of Khetib et al. (Citation2022) showed that Nuavg was increased by augmenting the Re and concentration of DWCNTs-TiO2/water nanofluid. In addition, at Re = 28,000 and φ = 3%, the installation of turbulators with innovative geometry (TIG) with PR (Pitch Ratio) = 4 within the solar collector increased the Nuavg by 63.46%. In the case of φ = 3% and by augmenting the Re from 7000 to 28,000, the energy and exergy performance was augmented by 22.19% and 23.26% for PR = 4 and PR = 1, respectively. Recently, novel machine-learning-based experimental data was implemented by the use of Bayesian optimisation coupled with boosted regression trees to predict the thermal performance of MWCNT-Fe3O4/water hybrid nanofluids inside FPSC systems (Said et al., Citation2022). The boosted regression tree model was optimised by 99.9% prognostic efficiency, and a peak thermal efficiency of 63.84% was attained at a Reynolds number of 1413.
1.3. Research objectives
A considerable amount of literature has been published on experimental and numerical studies of hybrid nanofluids inside FPSCs. However, there has been relatively little technological literature published on the effects of different nanocomposite types in terms of different nanoparticle shape, different nanoparticle size and different nanomaterial mixing ratios inside solar collectors. Thus, this study aims to discuss the computational study of the influences of using seven different types of hybrid nanofluid, namely 0.1%-Al2O3@Cu/DW, 0.1%-MWCNTs@Fe3O4/DW, 0.3%-MWCNTs@Fe3O4/DW, 0.5%-Ag@MgO/DW, 1%-Ag@MgO/DW, 1%-S1 and 1%-S2. The FPSC thermal model was solved under the conditions 293K inlet temperature, 1000 W/m2 heat flux and 500 ≤ Re ≤ 1900. Six different parameters, i.e. surface heat transfer coefficient, pressure drop, outlet temperature, surface temperature, heat gain and thermal efficiency, were analysed to examine the best alternatives for base fluids. Previous experimental and computational investigations of the use of nanofluids as working fluids in FPSCs are summarised in Table .
Table 1. Previous experimental and computational studies on the use of hybrid nanofluids inside FPSCs.
2. Research methodology
2.1. Problem description
A conjugated forced convection model is solved numerically via a three-dimensional physical problem using a thin flat plate made of aluminium attached to a working fluid pipe made of copper (see Figure ). In this study, the radiation model is not activated and is replaced by constant wall heat flux for heating the solar collector surface (Liu et al., Citation2020). The physical dimensions of the solar collector model are as follows: length of thin flat plate, L = 914.4 mm; width of thin flat plate, w = 128 mm; thickness of thin flat plate, t = 2 mm; inner hydraulic diameter (IDh), 12.5 mm; outside hydraulic diameter (ODh), 14.8 mm; and wall thickness, 1 mm. The computational domain is divided into a large number of elements (cells) in order to improve grid size control and meshing efficiency. The feature of inflation is selected near the working fluid pipe wall, as shown in Figure .
2.2. Mathematical model
A physical model of a 3D FPSC with tilt angle 30° was tested numerically using seven different types of hybrid nanofluid with different nanomaterials and different nanoparticle concentrations. As mentioned above, the thermo-physical properties of the hybrid nanofluids and H2O were collected from the literature review at an inlet temperature of 293 K (Sarsam et al., Citation2022; Vatani & Mohammed, Citation2013). The following assumptions are made in the current model: the flow is a steady state, the flow is Newtonian, the nanofluid is undergoing single-phase flow and the flow is laminar. The wall heat flux was constant at 1000 W/m2, being the intensity of solar radiation. Meanwhile, non-slip and adiabatic boundary conditions were applied in the current simulation cases. Moreover, gravity was activated with a magnitude of −9.81 m/s2 in the normal direction of the y-axis. The conservation equations of continuity, momentum and energy are written in the mathematical expressions as follows (Akbarinia & Behzadmehr, Citation2007; Cerón et al., Citation2015; Edalatpour & Solano, Citation2017):
(1)
(1)
(2)
(2)
(3)
(3) Here, the symbol “i” equals 1,2,3, and the velocity vector “ui” equals (u, v, w).
Meanwhile, the boundary conditions (BCs) and input parameters of the 3D FPSC model are clarified by the following equations (Bianco et al., Citation2014; Charjouei Moghadam et al., Citation2017; Edalatpour & Solano, Citation2017):
(4)
(4)
(5)
(5)
(6)
(6)
(7)
(7)
(8)
(8)
In the following equations, the Reynolds number (Re), Prandtl number (Pr), pressure loss (ΔP), surface heat transfer coefficient (hsur), Nusselt number (Nu) and solar collector efficiency (ηSC), can be represented as (Edalatpour & Solano, Citation2017; Sarsam et al., Citation2020, Citation2022)
(9)
(9)
(10)
(10)
(11)
(11)
(12)
(12)
(13)
(13)
(14)
(14)
2.3. Thermo-Physical properties
A wide range of hybrid nanofluids are compared in this numerical evaluation in terms different nanomaterials, different mixing ratios and different concentrations. Furthermore, the thermo-physical properties and essential data were collected from previously published articles in order to test for the most efficient hybrid nanofluids as alternative working fluids for solar collector applications. Table shows the thermo-physical properties of the base fluid (H2O) and different types of hybrid nanofluid that were involved in the current assessments. Firstly, the nanocomposites used in the experiments of Suresh et al. (Citation2011) were chosen. Also, the MWCNT@Fe3O4 hybrid nanofluid in the mixing ratio 74%@Fe3O4 and 26%@MWCNTs (Lomascolo et al., Citation2015) were used. The Ag@MgO nanocomposite in the mixing ratio 50%-Ag@50%-MgO (Hemmat Esfe et al., Citation2015), and the 2CuO-1Cu (S1) and 1CuO-2Cu (S2) nanocomposites were tested by Balla et al. (Citation2013). In their studies, the viscosity and thermal conductivity were measured experimentally. The density and specific heat capacity were estimated using the data of Pak and Cho (Citation1998).
Table 2. Thermo-physical properties of H2O and different types of hybrid nanofluid at 293 K (Minea, Citation2017).
3. Validation and verification approaches
In order to conduct the grid independence test, five different grids were tested in the current numerical simulations. The grid independence test is carried out firstly using H2O as a working fluid to ensure the validity and accuracy of the tested computational grids. The working fluid was under the conditions Re = 500 and inlet temperature = 293 K. Six different output parameters were used in the evaluations, i.e. surface heat transfer coefficient, pressure drop, outlet temperature, heat gain, solar collector efficiency and surface temperature. Table indicates that, as the number of elements rises, so does the accuracy of the data obtained. Owing to its correctness and accuracy, grid number 5 with 872,000 elements was acceptable for processing the remaining tests in the current research.
Table 3. Grid independence test using different grid domains under the conditions 293 K and Reynolds number 500.
Moreover, the current numerical results were compared with the experimental data reported by Verma et al. (Citation2018) as shown in Figure in order to validate the current physical problem. In Figure (a), the base fluid and CuO@MWCNTs and MgO@MWCNTs hybrid nanofluids under conditions Ta = 300 K, I = 800 W/m2 and 0.025 kg/s were validated with different volume fractions. Meanwhile, as shown in Figure (b), the base fluid and CuO@MWCNTs and MgO@MWCNTs nanocomposites were compared under the settings Ta = 298 and 0.025 kg/s with different intensities. The current data showed an average error of 6.33%, 6.66% and 6.54%, respectively, when compared with the experimental results. Another validation was carried out between the current model and the previous (experimental and numerical) report by Farajzadeh et al. (Citation2018). Figure (a) shows a comparison with DW and 0.1 wt.%-Al2O3@TiO2/DW at 1.5 LPM. Meanwhile, Figure (b) illustrates the comparison for 0.1 wt.%-Al2O3@TiO2/DW at 1.5, 2 and 2.5 LPM. The present results show an average error of 4.7%, 5.2%, 4.8% and 5.6% for DW, and 0.1 wt.%-Al2O3@TiO2/DW at different volume flow rates, respectively.
Figure 2. Comparison between the current data and the previous results of Verma et al. (Citation2018).

Figure 3. Comparison between the present work and the experimental results of Farajzadeh et al. (Citation2018).

4. Results and discussion
4.1. Thermo-physical properties
The addition of solid nanoparticles to the base fluid (DW) changes the thermo-physical properties of the produced nanofluids. In the current investigation, different parameters are taken into consideration such as temperature, different types of nanomaterial, different concentrations and different mixing ratios. As per Figure , the density of produced nanocomposites was increased by 0.28%, 0.38%, 1.16%, 3.02%, 6.05%, 6.12% and 7.14% for 0.1%-Al2O3@Cu/DW, 0.1%-MWCNTs@Fe3O4/DW, 0.3%-MWCNTs@Fe3O4/DW, 0.5%-Ag@MgO/DW, 1%-Ag@MgO/DW, 1%-S1 and 1%-S2, respectively. The thermal conductivity of different hybrid nanofluids was improved by 2.99%, 11.86%, 13.89%, 4.65%, 9.47%, 32.06% and 40.03% for 0.1%-Al2O3@Cu/DW, 0.1%-MWCNTs@Fe3O4/DW, 0.3%-MWCNTs@Fe3O4/DW, 0.5%-Ag@MgO/DW, 1%-Ag@MgO/DW, 1%-S1 and 1%-S2, respectively. Moreover, the dynamic viscosity was significantly increased up to 17.72%, 15.19%, 27.85%, 6.08%, 12.66%, 140.51% and 178.48% for 0.1%-Al2O3@Cu/DW, 0.1%-MWCNTs@Fe3O4/DW, 0.3%-MWCNTs@Fe3O4/DW, 0.5%-Ag@MgO/DW, 1%-Ag@MgO/DW, 1%-S1 and 1%-S2, respectively. On the other hand, the specific heat capacity dropped insignificantly by 0.12%, 0.43%, 0.73%, 0.88% and 0.90% for 0.1%-Al2O3@Cu/DW, 0.5%-Ag@MgO/DW, 1%-Ag@MgO/DW, 1%-S1 and 1%-S2, respectively. 0.1%-MWCNTs@Fe3O4/DW and 0.3%-MWCNTs@Fe3O4/DW showed different behaviour and the specific heat capacity increased slightly by 0.02% and 0.05%. The thermal properties of nanocomposites were influenced by different scenarios like type, size and shape of the nanomaterials (Gupta et al., Citation2018). Nanofluids in cylindrical shapes showed higher values for thermal properties than nanofluids in spherical shapes. The nanofluids with smaller shaped nanoparticles exhibited better thermal performance. Moreover, the nanofluids with metallic materials were superior to non-metallic nanomaterials. The mass/volume fractions were the main parameters in increasing the values of the dynamic viscosity of nanofluids. It was apparently that the thermal properties were dependent on two main mechanisms, i.e. the interfacial layer (nanolayer) and Brownian motion (Gupta et al., Citation2017).
4.2. Outlet and flat plate surface temperatures
The outlet fluid temperature and flat plate surface temperature are discussed in this section as a function of 500 ≤ Re ≤ 1900 for the base fluid (DW) and seven water based hybrid nanofluids. As shown in Figures and , outlet and flat plate surface temperatures decrease as the Reynolds number increases. When the Reynolds number is increased from 500 (lower value) to 1900 (higher value), the outlet and flat plate surface temperatures are decreased by 4.37% and 3.47% for DW, 3.87% and 2.93% for 0.1%-Al2O3-Cu/DW, 3.86% and 3.01% for 0.1%-MWCNTs-Fe3O4/DW, 3.55% and 2.69% for 0.3%-MWCNTs-Fe3O4/DW, 4.18% and 3.27% for 0.5% Ag-MgO/DW, 3.96% and 3.08% for 1% Ag-MgO/DW, 2.66% and 1.86% for 1%-S1, and 2.49% and 1.71% for 1%-S2. The base fluid shows the higher values for outlet fluid temperature and flat plate surface temperature followed by 0.5%-Ag-MgO/DW, 1%-Ag-MgO/DW, 0.1%-Al2O3-Cu/DW, 0.1%-MWCNTs-Fe3O4/DW and 0.3%-MWCNTs-Fe3O4/DW. Meanwhile, 1%-S1 and 1%-S2 present the lower values. This decline in outlet/surface temperatures can be credited to the improved thermal conductivity in the presence of water-based hybrid nanofluids as a result of the increased convective heat transfer coefficient (Sarsam et al., Citation2015, Citation2020). As per Table , 1%-S1 and 1%-S2 nanocomposites showed better improvements in thermal conductivity over the base fluid with 32.06% and 40.03%, respectively. Previous studies on laminar and turbulent nanofluids flows proved that the heat transfer fluids (HTFs) with better thermal conductivities than their base fluids (DW) will also have greater convective heat transfer coefficients (Akram et al., Citation2021; Kumar et al., Citation2021). Moreover, Appendix compares the temperature profiles of different hybrid nanofluids at Re = 700. As can be seen from the temperature labels, in all cases the temperature is increased from the centre of the tube toward the radial direction. The reason for this is justified by the formation of boundary layers and their growth.
4.3. Heat transfer coefficient and pressure drop
The heat transfer coefficient and pressure drop are discussed in this section as a function of 500 ≤ Re ≤ 1900 for the base fluid (DW) and seven water based hybrid nanofluids. As shown in Figures and , the heat transfer coefficient and pressure drop are increased as Reynolds number increases. When the Reynolds number is increased from 500 (lower value) to 1900 (higher value), the heat transfer coefficient and pressure drop are increased by 34.59% and 81.57% for DW, 23.69% and 81.56% for 0.1%-Al2O3-Cu/DW, 33.15% and 81.57% for 0.1%-MWCNTs-Fe3O4/DW, 32.02% and 81.58% for 0.3%-MWCNTs-Fe3O4/DW, 33.91% and 81.56% for 0.5%-Ag-MgO/DW, 33.36% and 81.56% for 1%-Ag-MgO/DW, 29.81% and 81.63% for 1%-S1, and 29.33% and 81.65% for 1%-S2. The base fluid shows the higher values for outlet fluid temperature and flat plate surface temperature followed by 0.5%-Ag-MgO/DW, 1%-Ag-MgO/DW, 0.1%-Al2O3-Cu/DW, 0.1%-MWCNTs-Fe3O4/DW and 0.3%-MWCNTs-Fe3O4/DW. Meanwhile, 1%-S1 and 1%-S2 present the lower values. 1%-S1 and 1%-S2 hybrid nanofluids report the higher values for heat transfer and hydrodynamic properties. On the other hand, DW illustrates the lower values for heat transfer coefficient and pressure drop followed by 0.5%-Ag-MgO/DW, 1%-Ag-MgO/DW, 0.1%-Al2O3-Cu/DW, 0.1%-MWCNTs-Fe3O4/DW and 0.3%-MWCNTs-Fe3O4/DW. As per Equatoin (11), the pressure drop is proportional to the value of working fluid velocity. At constant Reynolds number, the fluid velocity changes with the change of the ratio µ/ρ. 1%-S1 and 1%-S2 nanocomposite fluids showed the higher values for fluid velocity, thus the pressure dropped significantly compared to the other types of hybrid nanofluid. Furthermore, Equation (12) explains why nanofluids have higher heat transfer coefficients than base fluids. The thermal conductivity of nanocomposite fluids is important for increasing the heat transfer parameters. In previous work, as well as in laminar and turbulent regimes, nanofluids with a higher thermal conductivity than their base fluids frequently have higher convective heat transfer coefficients (Sadri et al., Citation2018).
4.4. Heat gain and collector efficiency
The heat gain and thermal efficiency of solar collectors are discussed in this section as a function of 500 ≤ Re ≤ 1900 for the base fluid (DW) and seven water based hybrid nanofluids. As shown in Figures and , useful heat and energy effectiveness increase as Reynolds number increases. When Reynolds number increased from 500 (lower value) to 1900 (higher value), useful heat and energy effectiveness are increased by 45.11% for DW, 47.37% for 0.1%-Al2O3-Cu/DW, 45.76% for 0.1%-MWCNTs-Fe3O4/DW, 47.41% for 0.3%-MWCNTs-Fe3O4/DW, 45.36% for 0.5%-Ag-MgO/DW, 45.72% for 1%-Ag-MgO/DW, 47.29% for 1%-S1 and 47.13% for 1%-S2. 1%-S1 and 1%-S2 hybrid nanofluids report the higher values for heat gain and collector efficiency. On the other hand, DW illustrates the lower values for useful heat and energy effectiveness followed by 0.5%-Ag-MgO/DW, 1%-Ag-MgO/DW, 0.1%-MWCNTs-Fe3O4/DW, 0.3%-MWCNTs-Fe3O4/DW and 0.1%-Al2O3-Cu/DW. The enhanced collector efficiency/heat gain can be attributed to the decreased flat plate surface temperature (Figure ) resulting in lower heat losses from the collector, i.e. increased collector efficiency/heat gain (Elcioglu et al., Citation2020; Tong et al., Citation2019). This behaviour is confirmed through Figures and , which display the variation of energy efficiency/heat gain versus Reynolds number (fluid velocity)for different types of hybrid nanofluid (Moravej et al., Citation2020). Furthermore, according to Equation (14), the values of energy gain and efficiency are related to mass flow rate, specific heat capacity, and the temperature differential between the outlet and input.
5. Conclusions
This paper discusses recent breakthroughs in computational analyses of hybrid nanofluid applications in FPSCs. The use of nanocomposites as new solar fluids can be considered a powerful approach to improving FPSCs’ performance. Base fluids and water-based hybrid nanofluids were tested and evaluated inside solar collectors in terms of heat transfer, fluid flow and energy effectiveness. The thermo-physical parameters of DW and seven hybrid nanofluids at 293 K were collected from published works and used as heat transfer fluids in numerical simulations. The working fluids were simulated under the condition of laminar flow in the range 500 ≤ Re ≤ 1900 and the heating rate was 1000 W/m2. The followings conclusions can be drawn.
The higher increments in thermal conductivity and dynamic viscosity were achieved by 1%-S1 and 1%-S2 with 32.06%, 40.03% and 140.51%, 178.48%, respectively. Also, thermal conductivity and dynamic viscosity for 0.3%-MWCNTs@Fe3O4/DW showed significant increments up to 13.89% and 27.85%.
The outlet temperature was decreased when fluid velocity increased. Relative to the base fluid, the average outlet temperature was decreased by 0.80% for 0.1%-Al2O3-Cu/DW, 0.94% for 0.1%-MWCNTs-Fe3O4/DW, 0.53% for 0.3%-MWCNTs-Fe3O4/DW, 0.87% for 0.5%-Ag-MgO/DW, 2.08% for 1%-Ag-MgO/DW, 2.28% for 1%-S1 and 2.28% for 1%-S2.
The surface temperature was decreased when fluid velocity increased. Relative to the base fluid, the average surface temperature was decreased by 1.23% for 0.1%-Al2O3-Cu/DW, 1.43% for 0.1%-MWCNTs-Fe3O4/DW, 0.79% for 0.3%-MWCNTs-Fe3O4/DW, 1.33% for 0.5%-Ag-MgO/DW, 3.23% for 1%-Ag-MgO/DW, 3.58% for 1%-S1 and 3.58% for 1%-S2.
The surface heat transfer coefficient was increased when fluid velocity increased. Relative to the base fluid, the average surface heat transfer coefficient was increased by 13.10% for 0.1%-Al2O3-Cu/DW, 15.76% for 0.1%-MWCNTs-Fe3O4/DW, 7.91% for 0.3%-MWCNTs-Fe3O4/DW, 15.32% for 0.5%-Ag-MgO/DW, 46.05% for 1%-Ag-MgO/DW, 53.66% for 1%-S1 and 53.66% for 1%-S2.
The pressure drop was increased when fluid velocity increased. Relative to the base fluid, the average pressure loss was increased by 48.01% for 0.1%-Al2O3-Cu/DW, 70.94% for 0.1%-MWCNTs-Fe3O4/DW, 28.70% for 0.3%-MWCNTs-Fe3O4/DW, 92.52% for 0.5%-Ag-MgO/DW, 522.73% for 1%-Ag-MgO/DW, 694.65% for 1%-S1 and 694.65% for 1%-S2.
The heat gain and collector efficiency were increased when fluid velocity increased. Relative to the base fluid, the average heat gain and collector efficiency were increased by 7.29% for 0.1%-Al2O3-Cu/DW, 10.79% for 0.1%-MWCNTs-Fe3O4/DW, 6.40% for 0.3%-MWCNTs-Fe3O4/DW, 11.08% for 0.5%-Ag-MgO/DW, 43.64% for 1%-Ag-MgO/DW, 48.92% for 1%-S1 and 48.92% for 1%-S2.
Disclosure statement
No potential conflict of interest was reported by the authors.
Data availability statement
The data used in this research presented in the article itself.
References
- Akbarinia, A., & Behzadmehr, A. (2007). Numerical study of laminar mixed convection of a nanofluid in horizontal curved tubes. Applied Thermal Engineering, 27, 1327–1337. https://doi.org/10.1016/j.applthermaleng.2006.10.034
- Akram, N., Montazer, E., Kazi, S. N., Soudagar, M. E. M., Ahmed, W., Zubir, M. N. M., Afzal, A., Muhammad, M. R., Ali, H. M., & Márquez, F. P. G. (2021). Experimental investigations of the performance of a flat-plate solar collector using carbon and metal oxides based nanofluids. Energy, 227, 120452. https://doi.org/10.1016/j.energy.2021.120452
- Akram, N., Sadri, R., Kazi, S. N., Zubir, M. N. M., Ridha, M., Ahmed, W., Soudagar, M. E. M., & Arzpeyma, M. (2020). A comprehensive review on nanofluid operated solar flat plate collectors. Journal of Thermal Analysis and Calorimetry, 139, 1309–1343. https://doi.org/10.1007/s10973-019-08514-z
- Alawi, O. A., Kamar, H. M., Abdelrazek, A. H., Mallah, A. R., Mohammed, H. A., Abdulla, A. I., Gatea, H. A., Khiadani, M., Kazi, S. N., & Yaseen, Z. M. (2022). Hydrothermal and energy analysis of flat plate solar collector using copper oxide nanomaterials with different morphologies: Economic performance. Sustainable Energy Technologies and Assessments, 49, 101772. https://doi.org/10.1016/j.seta.2021.101772
- Alawi, O. A., Kamar, H. M., Mallah, A. R., Mohammed, H. A., Kazi, S. N., Sidik, N. A. C., & Najafi, G. (2021). Nanofluids for flat plate solar collectors: Fundamentals and applications. Journal of Cleaner Production, 291, 125725. https://doi.org/10.1016/j.jclepro.2020.125725
- Alawi, O. A., Kamar, H. M., Mallah, A. R., Mohammed, H. A., Sabrudin, M. A. S., Salim Newaz, K. M., Najafi, G., & Yaseen, Z. M. (2021). Experimental and theoretical analysis of energy efficiency in a flat plate solar collector using monolayer graphene nanofluids. Sustainability, 13(10), 5416. https://doi.org/10.3390/su13105416.
- Alrowaili, Z. A., Ezzeldien, M., Shaaalan, N. M., Hussein, E., & Sharafeldin, M. A. (2022). Investigation of the effect of hybrid CuO-Cu/water nanofluid on the solar thermal energy storage system. Journal of Energy Storage, 50, 104675. https://doi.org/10.1016/j.est.2022.104675
- Balla, H. H., Abdullah, S., MohdFaizal, W., Zulkifli, R., & Sopian, K. (2013). Numerical study of the enhancement of heat transfer for hybrid CuO-Cu nanofluids flowing in a circular pipe. Journal of Oleo Science, 62(7), 533–539. https://doi.org/10.5650/jos.62.533
- Bezaatpour, M., & Rostamzadeh, H. (2021). Simultaneous energy storage enhancement and pressure drop reduction in flat plate solar collectors using rotary pipes with nanofluid. Energy and Buildings, 239, 110855. https://doi.org/10.1016/j.enbuild.2021.110855
- Bianco, V., Manca, O., & Nardini, S. (2014). Entropy generation analysis of turbulent convection flow of Al2O3–water nanofluid in a circular tube subjected to constant wall heat flux. Energy Conversion and Management, 77, 306–314. https://doi.org/10.1016/j.enconman.2013.09.049
- Cerón, J. F., Pérez-García, J., Solano, J. P., García, A., & Herrero-Martín, R. (2015). A coupled numerical model for tube-on-sheet flat-plate solar liquid collectors. Analysis and validation of the heat transfer mechanisms. Applied Energy, 140, 275–287. https://doi.org/10.1016/j.apenergy.2014.11.069
- Charjouei Moghadam, M., Edalatpour, M., & Solano, J. P. (2017). Numerical study on conjugated laminar mixed convection of alumina/water nanofluid flow, heat transfer, and entropy generation within a tube-on-sheet flat plate solar collector. Journal of Solar Energy Engineering, 139(4), 041011. https://doi.org/10.1115/1.4036854.
- Edalatpour, M., & Solano, J. P. (2017). Thermal-hydraulic characteristics and exergy performance in tube-on-sheet flat plate solar collectors: Effects of nanofluids and mixed convection. International Journal of Thermal Sciences, 118, 397–409. https://doi.org/10.1016/j.ijthermalsci.2017.05.004
- Elcioglu, E. B., Genc, A. M., Karadeniz, Z. H., Ezan, M. A., & Turgut, A. (2020). Nanofluid figure-of-merits to assess thermal efficiency of a flat plate solar collector. Energy Conversion and Management, 204, 112292. https://doi.org/10.1016/j.enconman.2019.112292
- Farajzadeh, E., Movahed, S., & Hosseini, R. (2018). Experimental and numerical investigations on the effect of Al2O3/TiO2H2O nanofluids on thermal efficiency of the flat plate solar collector. Renewable Energy, 118, 122–130. https://doi.org/10.1016/j.renene.2017.10.102
- Gupta, M., Singh, V., Kumar, R., & Said, Z. (2017). A review on thermophysical properties of nanofluids and heat transfer applications. Renewable and Sustainable Energy Reviews, 74, 638–670. https://doi.org/10.1016/j.rser.2017.02.073
- Gupta, M., Singh, V., Kumar, S., Kumar, S., Dilbaghi, N., & Said, Z. (2018). Up to date review on the synthesis and thermophysical properties of hybrid nanofluids. Journal of Cleaner Production, 190, 169–192. https://doi.org/10.1016/j.jclepro.2018.04.146
- Hemmat Esfe, M., Arani, A. A. A., Rezaie, M., Yan, W. M., & Karimipour, A. (2015). Experimental determination of thermal conductivity and dynamic viscosity of Ag–MgO/water hybrid nanofluid. International Communications in Heat and Mass Transfer. https://doi.org/10.1016/j.icheatmasstransfer.2015.06.003
- Hussein, O. A., Habib, K., Muhsan, A. S., Saidur, R., Alawi, O. A., & Ibrahim, T. K. (2020). Thermal performance enhancement of a flat plate solar collector using hybrid nanofluid. Solar Energy, 204, 208–222. https://doi.org/10.1016/j.solener.2020.04.034
- Khetib, Y., Alzaed, A., Tahmasebi, A., Sharifpur, M., & Cheraghian, G. (2022). Influence of using innovative turbulators on the exergy and energy efficacy of flat plate solar collector with DWCNTs-TiO2/water nanofluid. Sustainable Energy Technologies and Assessments, 51, 101855. https://doi.org/10.1016/j.seta.2021.101855
- Kumar, L. H., Kazi, S. N., Masjuki, H. H., Zubir, M. N. M., Jahan, A., & Bhinitha, C. (2021). Energy, exergy and economic analysis of liquid flat-plate solar collector using green covalent functionalized graphene nanoplatelets. Applied Thermal Engineering, 192, 116916. https://doi.org/10.1016/j.applthermaleng.2021.116916
- Liu, S., Afan, H. A., Aldlemy, M. S., Al-Ansari, N., & Yaseen, Z. M. (2020). Energy analysis using carbon and metallic oxides-based nanomaterials inside a solar collector. Energy Reports, 6, 1373–1381. https://doi.org/10.1016/j.egyr.2020.05.015
- Lomascolo, M., Colangelo, G., Milanese, M., & De Risi, A. (2015). Review of heat transfer in nanofluids: Conductive, convective and radiative experimental results. Renewable and Sustainable Energy Reviews, 43, 1182–1198. https://doi.org/10.1016/j.rser.2014.11.086
- Mackolil, J., & Mahanthesh, B. (2021). Optimization of heat transfer in the thermal marangoni convective flow of a hybrid nanomaterial with sensitivity analysis. Applied Mathematics and Mechanics, 42(11), 1663–1674. https://doi.org/10.1007/s10483-021-2784-6
- Mahanthesh, B. (2021a). Flow and heat transport of nanomaterial with quadratic radiative heat flux and aggregation kinematics of nanoparticles. International Communications in Heat and Mass Transfer, 127, 105521. https://doi.org/10.1016/j.icheatmasstransfer.2021.105521
- Mahanthesh, B. (2021b). Quadratic radiation and quadratic boussinesq approximation on hybrid nanoliquid flow. In B. Mahanthesh (Ed.), Mathematical fluid mechanics advances in convective instabilities and incompressible fluid flow (pp. 13–54). De Gruyter. https://doi.org/10.1515/9783110696080
- Maleki, A., Haghighi, A., & Mahariq, I. (2021). Machine learning-based approaches for modeling thermophysical properties of hybrid nanofluids: A comprehensive review. Journal of Molecular Liquids, 322, 114843. https://doi.org/10.1016/j.molliq.2020.114843
- Minea, A. A. (2017). Challenges in hybrid nanofluids behavior in turbulent flow: Recent research and numerical comparison. Renewable and Sustainable Energy Reviews, 71, 426–434.
- Moravej, M., Bozorg, M. V., Guan, Y., Li, L. K. B., Doranehgard, M. H., Hong, K., & Xiong, Q. (2020). Enhancing the efficiency of a symmetric flat-plate solar collector via the use of rutile TiO2-water nanofluids. Sustainable Energy Technologies and Assessments, 40, 100783. https://doi.org/10.1016/j.seta.2020.100783
- Mortazavinejad, S. M., & Mozafarifard, M. (2019). Numerical investigation of two-dimensional heat transfer of an absorbing plate of a flat-plate solar collector using dual-reciprocity method based on boundary element. Solar Energy, 191, 332–340. https://doi.org/10.1016/j.solener.2019.08.075
- Okonkwo, E. C., Wole-Osho, I., Kavaz, D., Abid, M., & Al-Ansari, T. (2020). Thermodynamic evaluation and optimization of a flat plate collector operating with alumina and iron mono and hybrid nanofluids. Sustainable Energy Technologies and Assessments, 37, 100636. https://doi.org/10.1016/j.seta.2020.100636.
- Pak, B. C., & Cho, Y. I. (1998). Hydrodynamic and heat transfer study of dispersed fluids with submicron metallic oxide particles. Experimental Heat Transfer, 11(2), 151–170. https://doi.org/10.1080/08916159808946559
- Ranga Babu, J. A., Kiran Kumar, K., & Srinivasa Rao, S. (2018). Thermodynamic analysis of hybrid nanofluid based solar flat plate collector. World Journal of Engineering, 15(1), 27–39. https://doi.org/10.1108/WJE-03-2017-0048
- Sadri, R., Mallah, A. R., Hosseini, M., Ahmadi, G., Kazi, S. N., Dabbagh, A., Yeong, C. H., Ahmad, R., & Yaakup, N. A. (2018). CFD modeling of turbulent convection heat transfer of nanofluids containing green functionalized graphene nanoplatelets flowing in a horizontal tube: Comparison with experimental data. Journal of Molecular Liquids, 269, 152–159. https://doi.org/10.1016/j.molliq.2018.06.011
- Said, Z., Sharma, P., Syam Sundar, L., Nguyen, V. G., Tran, V. D., & Le, V. V. (2022). Using Bayesian optimization and ensemble boosted regression trees for optimizing thermal performance of solar flat plate collector under thermosyphon condition employing MWCNT-Fe3O4/water hybrid nanofluids. Sustainable Energy Technologies and Assessments, 53, 102708. https://doi.org/10.1016/j.seta.2022.102708
- Sarsam, W. S., Kazi, S. N., & Badarudin, A. (2015). A review of studies on using nanofluids in flat-plate solar collectors. Solar Energy, 122, 1245–1265. https://doi.org/10.1016/j.solener.2015.10.032
- Sarsam, W. S., Kazi, S. N., & Badarudin, A. (2020). Thermal performance of a flat-plate solar collector using aqueous colloidal dispersions of graphene nanoplatelets with different specific surface areas. Applied Thermal Engineering, 172, 115142. https://doi.org/10.1016/j.applthermaleng.2020.115142
- Sarsam, W. S., Kazi, S. N., & Badarudin, A. (2022). Thermal performance of a flat-plate solar collector using aqueous colloidal dispersions of multi-walled carbon nanotubes with different outside diameters. Experimental Heat Transfer, 35(3), 258–281. https://doi.org/10.1080/08916152.2020.1847215
- Shah, T. R., & Ali, H. M. (2019). Applications of hybrid nanofluids in solar energy, practical limitations and challenges: A critical review. Solar Energy, 183, 173–203. https://doi.org/10.1016/j.solener.2019.03.012
- Sheikholeslami, M., Farshad, S. A., Ebrahimpour, Z., & Said, Z. (2021). Recent progress on flat plate solar collectors and photovoltaic systems in the presence of nanofluid: A review. Journal of Cleaner Production, 293, 126119. https://doi.org/10.1016/j.jclepro.2021.126119
- Singh, V. V., Thakur, J., Agarwal, R., Vyas, G., & Dondapati, R. S. (2018). Computational evaluation of thermal and hydraulic characteristics of flat-plate solar collector for different glazing material. Materials Today: Proceedings, 5(14), 28211–28220. https://doi.org/10.1016/j.matpr.2018.10.065
- Sundar, L. S., Misganaw, A. H., Singh, M. K., Sousa, A. C. M., & Ali, H. M. (2021). Efficiency analysis of thermosyphon solar flat plate collector with low mass concentrations of ND–Co3O4 hybrid nanofluids: An experimental study. Journal of Thermal Analysis and Calorimetry, 143(2), 959–972. https://doi.org/10.1007/s10973-020-10176-1
- Suresh, S., Venkitaraj, K. P., Selvakumar, P., & Chandrasekar, M. (2011). Synthesis of Al2O3–Cu/water hybrid nanofluids using two step method and its thermo physical properties. Colloids and Surfaces A: Physicochemical and Engineering Aspects, 388(1–3), 41–48. https://doi.org/10.1016/j.colsurfa.2011.08.005
- Thriveni, K., & Mahanthesh, B. (2021). Sensitivity computation of nonlinear convective heat transfer in hybrid nanomaterial between two concentric cylinders with irregular heat sources. International Communications in Heat and Mass Transfer, 129, 105677. https://doi.org/10.1016/j.icheatmasstransfer.2021.105677
- Tiwar, A. K., Kumar, V., Said, Z., & Paliwal, H. K. (2021). A review on the application of hybrid nanofluids for parabolic trough collector: Recent progress and outlook. Journal of Cleaner Production, 292, 126031. https://doi.org/10.1016/j.jclepro.2021.126031
- Tong, Y., Hoseong, W. K., & Cho, H. (2019). Energy and exergy comparison of a flat-plate solar collector using water, Al2O3 nanofluid, and CuO nanofluid. Applied Thermal Engineering, 159, 113959. https://doi.org/10.1016/j.applthermaleng.2019.113959
- Vatani, A., & Mohammed, H. A. (2013). Turbulent nanofluid flow over periodic rib-grooved channels. Engineering Applications of Computational Fluid Mechanics, 7(3), 369–381. https://doi.org/10.1080/19942060.2013.11015478
- Verma, S. K., Tiwari, A. K., Tiwari, S., & Chauhan, D. S. (2018). Performance analysis of hybrid nanofluids in flat plate solar collector as an advanced working fluid. Solar Energy, 167, 231–241. https://doi.org/10.1016/j.solener.2018.04.017
- Visa, I., Moldovan, M., & Duta, A. (2019). Novel triangle flat plate solar thermal collector for facades integration. Renewable Energy, 143, 252–262. https://doi.org/10.1016/j.renene.2019.05.021
- Xiong, Q., Tayebi, T., Izadi, M., Siddiqui, A. A., Ambreen, T., & Li, L. K. B. (2021). Numerical analysis of porous flat plate solar collector under thermal radiation and hybrid nanoparticles using two-phase model. Sustainable Energy Technologies and Assessments, 47, 101404. https://doi.org/10.1016/j.seta.2021.101404
- Zayed, M. E., Zhao, J., Du, Y., Kabeel, A. E., & Shalaby, S. M. (2019). Factors affecting the thermal performance of the flat plate solar collector using nanofluids: A review. Solar Energy, 182, 382–396. https://doi.org/10.1016/j.solener.2019.02.054
- Zhou, L., Wang, Y., & Huang, Q. (2019). CFD investigation of a new flat plate collector with additional front side transparent insulation for use in cold regions. Renewable Energy, 138, 754–763. https://doi.org/10.1016/j.renene.2019.02.014