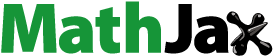
Abstract
Supercavitation has been recently presented as an effective method for the drag reduction of underwater vehicles. However, maintaining the supercavitating state requires a lot of energy, making vehicles difficult to control. Therefore, it is necessary to design an underwater vehicle with low drag in the fully wetted state while being able to move at ultra-high speed in the supercavitating state. In this study, a detachable fairing design for underwater vehicles is proposed, which has the advantage of increasing the total voyage and avoiding the problem of difficult steering in the supercavitating state. On the other hand, the study of non-body-of-revolution (non-BOR) has become a prevalent area of interest in the shape design of underwater vehicles. The cavity generated by an elliptical disk-shaped cavitator is studied numerically. It is found that the cavity profile on the cross-section near the cavitator is approximately elliptical. The cavity length of an elliptical disk-shaped cavitator is almost the same as that of a disk-shaped cavitator when they have the same inflow area. Based on these two characteristics, the parameters of the internal elliptical disk-shaped cavitator are optimized, which provides a promising strategy for the issue of cavitators increasing drag in a fully wetted state.
1. Introduction
When the relative velocity of an underwater body with water exceeds a certain threshold, the static pressure of liquid near the surface will reduce to below the liquid’s vapor pressure, resulting in the formation of small vapor-filled cavities in the liquid, which is cavitation. If the velocity of the underwater body increases further and the generated cavity is large enough to envelop practically the whole body, the cavity is referred to as a supercavity. A supercavity greatly reduces the skin friction drag by acting on the wetted body, allowing an underwater vehicle to move more rapidly. For example, when cavitation number σ = 10−2, the drag reduction rate for slender rotary bodies can reach 95% (Savchenko, Citation2001); however, the following problems must be addressed for supercavitating drag reduction for underwater vehicles to be implemented steadily: shape design of cavitators (Erfanian & Anbarsooz, Citation2018) and underwater vehicles (Jiang et al., Citation2019), ventilation control of ventilated supercavities (Zou et al., Citation2021), and motion pattern design of supercavitating underwater vehicles (Chen et al., Citation2014). The Russian Navy developed the VA-111 Shkval supercavitating torpedo, which employs rocket propulsion and outperforms the speed of conventional torpedoes by a ratio of five (Petitpas et al., Citation2011). The successful launch of the Shkval supercavitating torpedo proves that supercavitation technology has important application value in the drag reduction of high-speed vehicles. Of course, supercavitating vehicles also have some problems. The first one is the weak maneuvering orbit. The cavity will block sound propagation so that torpedoes are enclosed in the cavity that cannot use acoustic homing devices. The problem results in limited steering ability. Second, high energy consumption leads to a shorter voyage. To solve the mentioned problems, it is necessary to design an underwater vehicle with low drag in the fully wetted state while having the ability to move at ultra-high speed in the supercavitating state. There are few types of research on such underwater vehicles. To improve the ability to control the starting position and the shape of the cavity, a device with sharp edges at the nose of the supercavitating underwater vehicle is generally used to induce a large pressure gradient and flow separation phenomenon in the flow field. This device is usually called a cavitator, which is the key to the formation and stability of the cavity. However, the cavitator's non-streamlined design adds drag at low speeds, which must be addressed.
The supercavitating flow can be produced in two ways. The first method is naturally increasing body velocity to reduce the liquid medium pressure to the desired saturated vapor pressure. The second method is to increase the pressure inside the cavity by artificially ventilating noncondensable gas around the body. Both ways are effective in reducing the drag on moving underwater bodies. When the cavitation number is the same, the natural cavity and the ventilated cavity have similar geometrical shapes and hydrodynamic characteristics (Zhang et al., Citation2007). Ventilated cavities are easier to achieve than natural cavities, improving cavities’ stability and lowing cavitation hazards (Sun et al., Citation2021b); therefore, supercavitating vehicles are usually ventilated to generate supercavities, which are also used in experiments. Logvinovich experimentally proved the famous independence principle of cavity section expansion (Savchenko et al., Citation2019), which is the basis of the theoretical model of the cavity. Rashidi et al. (Citation2014) and Ahn et al. (Citation2017) showed that the pressure measurement values at different points in the cavity were almost identical when the supercavity interface remained transparent. Jiang et al. (Citation2019) found that the drag coefficients strongly depend on the body shapes behind the cavitator. Qin et al. (Citation2019) classified cavity regimes into four types through the flow field of ventilated cavitation captured by the imaging system, referred to as the foamy cavity, transition cavity, open cavity, and two-branch cavity. These experiments were mainly carried out in cavitation water tunnels, which was a very effective way to study the cavitation phenomenon. However, water tunnel tests are usually carried out in narrow tunnels, so scaling tests must be carried out. In addition, there are some problems, such as high experimental cost and blockage effect. Ahn et al. (Citation2017) captured the cavity evolution process with high-speed cameras and analyzed several supercavity closure modes. It is discovered that the blockage ratio can determine the critical ventilation velocity in the water tunnel experiment. The supercavity will collapse into a foam cavity when the blockage ratio is too high.
Numerical simulation is an effective way to study the cavitation phenomenon. It has the outstanding advantage of low cost, but a necessary step is to carry out comparative experiments to verify the accuracy. With the development of computational fluid dynamics (CFD) and computer performance, additional equations such as the barotropic relation or volume fraction transport equations have been introduced to capture cavitation phenomena numerically, known as cavitation models (Kunz et al., Citation2000). Ji et al. (Citation2010) simulated the cavity characteristics of natural cavitation and ventilated cavitation around the underwater vehicle, respectively, including the length, diameter, and shape of the cavity. The predicted results were in agreement with the experimental results. Fard and Nikseresht (Citation2012) used the finite volume method to approach the robust solutions of the unsteady cavitating flows around a circular disk and a conical disk cavitator. Nesteruk (Citation2014) assumed that the gas between the slender axisymmetric supercavity and the body of the revolution was incompressible and inviscid. The unsteady evolution of the cavity was numerically calculated, which was shown that the shape of the cavity depends on the ventilation rate and the cavitation number. Erfanian and Anbarsooz (Citation2018) investigated the effect of adding concentric holes of different diameters on the cavity characteristics of a disk-shaped cavitator. The drag coefficient and cavity length decreased together when the hole diameter exceeded a certain threshold.
Unmanned underwater vehicles can replace divers or manned mini-submarines for deep-sea exploration, life-saving, mine removal and other high-risk underwater operations, which have many types of body shapes based on purpose. The traditional underwater vehicle has an elliptical frontal shape, followed by a cylindrical midsection and a conical back portion, whose main body shape belongs to the body-of-revolution (BOR) hull. In recent years, the study of non-body-of-revolution (non-BOR) has become a prevalent area of interest in the shape design of underwater vehicles (Delaney, Citation2013; Citation2021). Non-BOR hulls provide greater internal volume with shorter hull lengths than traditional BOR designs, providing improved operational capabilities. It is critical to conduct a study on non-BOR supercavitating underwater vehicles, particularly cavitator design. Currently, the research on the shape of cavitators is mainly on rotary cavitators, among which the disk-shaped cavitators (Lee et al., Citation2018) and conical-shaped cavitators (Kadivar et al., Citation2017; Pendar & Roohi, Citation2016) are the most widely studied. Special cavitator shapes are being developed to improve hydrodynamic performance. Kuklinski designed cavitators with a variable angle of attack, star cavitators and snowflake cavitators (Huang et al., Citation2020). Other special-shaped cavitators studied include a conical cavitator with fins (Li & Dang, Citation2016), a 1/4 spherical cavitator (Zhang et al., Citation2016), a variable-lateral-force cavitator (Hu et al., Citation2017), a double-disc cavitator (Deng et al., Citation2019), and a retractable cylindrical cavitator (Gieseke, Citation2011). Chen et al. (Citation2020) proposed elliptical disk-shaped cavitators for non-BOR underwater vehicles with the potential to reduce drag.
This study aims to design an underwater vehicle that can move with less drag in both the fully wetted state and supercavitating state, using a bionic non-BOR shape designed by Sun et al. (Citation2021a) as a basis for further shape design. In order to study the hydrodynamic characteristics of the cavities generated by elliptical disk-shaped cavitators, simulations were conducted in the same operating conditions as the experiments. Then, a detachable fairing design for underwater vehicles is proposed, which has the advantage of reducing drag in the fully wetted state to increase the total voyage. Finally, the elliptical disk-shaped cavitator shape was optimized in the simulation to provide technical support for non-BOR underwater vehicles.
2. Numerical method and validation
2.1. High-speed water tunnel experiment
A bionic non-BOR shape of an underwater vehicle designed by Sun et al. (Citation2021a) is used as a basis for further shape design, whose length is kept at 4.9 m, as shown in Figure . The shape obtained by numerical simulation and test has less drag in a straight line. The difference between this shape and the conventional shape is that the main section is an ellipse.
Figure 1. The bionic non-BOR shape of the underwater vehicle (Sun et al., Citation2021a).

The experiment was conducted in the water tunnel of the High-speed Water Tunnel Laboratory of Northwestern Polytechnical University (NPU), China. The bionic non-BOR shape shrinkage model was used in the ventilation cavitation experiment to verify the ability to generate a stable cavity by the elliptical disk-shaped cavitator. The size of the test model used in the water tunnel experiment is shown in Figure (a). The model's nose was fitted with an elliptical disk-shaped cavitator with a long axis length of 32.5 mm and a short axis length of 24.6 mm. Vents designed for 6×Φ3 mm round holes were evenly spaced in the circumferential direction behind the cavitator, and ventilation volume was controlled by a mass flowmeter. The test model was designed with a hollow cavity structure. The three-component strain gauge balance was embedded in the model body to measure the drag of the model. The section view in the xOz plane is shown in Figure (b). The high-speed camera was used to film the cavitation phenomenon during the experiment. More details of the experimental installation can be found in Chen et al. (Citation2020).
Figure 2. The test model used in the cycled high-speed water tunnel experiment. (a). The size of the test model. (b). The section view in the xOz plane.

The following were the experimental conditions. The static pressure of the inner wall of the water tunnel was kept at 75 kPa. The freestream velocity was kept at 8 m/s. The Reynolds number (Re) and Froude number (Fr) can be calculated as.
where ρ is the water density, U∞ is the freestream velocity, L is the model length, g is the gravitational acceleration, and μ is the water viscosity at 20°C. The volumetric air flow rate was kept at 30 L/min. The camera captured the whole process, from forming the gas bubbles to forming a stable cavity enveloping the model, as shown in Figure (left).
2.2. Numerical analysis
2.2.1. Numerical model
The cavitation phenomenon belongs to multi-phase simulation in CFD. Its solution is complicated due to the different material parameters of different phases. A homogeneous equilibrium model is used to solve the Reynolds Averaged Navier-Strokes (RANS) equation to calculate the cavity profiles, similar to the studies (Sun et al., Citation2021b; Kim et al., Citation2020; Mansour et al., Citation2020). The homogeneous equilibrium model assumes that the velocity, pressure, and temperature of various phases or components are equal, which satisfies the fluid continuity hypothesis and shares a set of momentum equations.
The Volume of Fluids (VOF) method was widely utilized to characterize the phase transition process between liquid and vapor phases that both exist in cavitating flows (Pendar & Roohi, Citation2016). It considers that there is only one kind of mixed fluid with variable density in the whole flow field, all phases share the same pressure field and velocity field, and there is no slip between different components. The volume fraction αn of phase n is introduced into each calculation element in the flow field to distinguish each component.
(1)
(1) where Vn is the volume of phase n and V is the volume of the cell.
Natural cavitation flow contains the liquid and vapor phases. For ventilated cavitation flow, non-condensable gas is inside the cavity, which contains the liquid phase, vapor, and gas phases. The governing equations of incompressible gas, vapor, and liquid phases are shown in Equations (Equation2(2)
(2) ) and (Equation3
(3)
(3) ). The mixture density is shown in Equation (Equation4
(4)
(4) ).
(2)
(2)
(3)
(3)
(4)
(4) where ui is the velocity in the direction i, ρk is the density of phase k, gi is the gravity in the direction i, and t is time. The viscous force τij is:
(5)
(5) where the viscosity μm is:
(6)
(6) where α is the volume fraction of the phase and the subscripts l, v, and g denote the liquid, vapor, and gas phases, respectively.
The RNG k-ϵ turbulence model is employed for cavitation simulation. The model was proposed by Yakhot et al. (Citation1992). It is useful for researching boundary layer flow and separation flow, which can deal with flows with high strain rates and large bending degrees. The equation of the RNG k-ϵ is:
(7)
(7)
(8)
(8)
(9)
(9) where Gk is the turbulent kinetic energy generated by the average velocity gradient; Gb is the turbulent kinetic energy generated by buoyancy; YM is the effect of compressible turbulent pulsation expansion on total dissipation rate; σk and σϵ are the negative Prandtl number of k equation and ϵ equation. The specific values obtained by the experimental measurement are σk = σϵ ≈1.393, C1ϵ = 1.42, C2ϵ = 1.68, C3ϵ = tan |v/u|, η = Sk/ϵ, η0 = 4.38, β = 0.012, where v and u are the velocity components parallel to and perpendicular to the gravity field vector, respectively.
The Schnerr-Sauer cavitation model is used to calculate the precise net mass transfer from liquid to vapor. The vapor volume fraction transfer equation is:
(10)
(10) The net mass source term is as follows:
(11)
(11)
The expression for connecting the vapor volume fraction to the number of bubbles per volume of liquid is:
(12)
(12) The derivation of equations (8-10) yields the following equation:
(13)
(13)
(14)
(14) where R is the mass transfer rate; RB is the bubble radius.
2.2.2. Simulation setup
Figure shows the boundary conditions and mesh division of the simulation. The water area is a cylinder. Suppose the length of the underwater vehicle is set to La. In that case, the velocity inlet is 3La from the nose of the underwater vehicle, and the pressure outlet is 6La from the tail of the underwater vehicle. The surface of the underwater vehicle is the wall boundary condition, and the gravity is oriented along the z-axis. Due to the complex shape and thin rudder, smaller grid sizes are required if unstructured grids are used, which is difficult to ensure the economic purpose. The entire water area is meshed with hexahedral structured meshes to improve the computational accuracy of the liquid–gas interface. Figure shows the grids of the underwater vehicle. The mesh quality is further improved by using O-block division for the ventilation holes and Y-block division for the rudder.
The RNG k-ϵ turbulence model with the Schnerr-Sauer cavitation model is employed. The typical measurements across a turbulent flow near the wall have three distinct zones: the viscous sublayer, the buffer layer and the logarithmic layer. In the case of high Re turbulent flow, the first grid node can be set in the logarithmic layer, which is the region of full turbulence. The wall boundary conditions for the solution variables in the viscous sublayer and the buffer layer are all taken care of by the wall functions. The non-equilibrium wall functions are sensitized to pressure-gradient effects, which is suitable for cavitation simulation (Li et al., Citation2018). The range of y+ is 30 < y+<300. The governing equation is the RANS equation based on a homogeneous VOF method. The continuity equations are solved separately in the liquid, vapor, and gas phases. The velocity inlet is set as 8 m/s. The pressure outlet is set as 75 kPa. The reference pressure is set as 0 Pa. The RANS equation solution algorithm is PISO (Pressure-Implicit with Splitting of Operators). The time step is 5 × 10−5 s. The model size, inlet velocity and other parameters in the simulations are the same as in the experiment; therefore, Re and Fr are correspondingly almost the same.
2.3. Morphological characteristics of ventilated cavity
Figure records the development process of the ventilated cavity generated by the elliptical disk-shaped cavitator experimentally and the corresponding numerical simulations. On the left are the experimental images; the time to begin ventilating is set as t0 when the cavitation cloud appears. There is no ventilation before t0, and there are a small number of bubbles in the water. At the moment [t0, t0 + 1 s], a group of cavitation clouds (a) was observed near the cavitator. As time went by, the cavitation cloud continued to accumulate, and many bubbles (b) could be seen on the surface of the model. Starting from t0 + 2s, it can be seen from the image that a long strip of a transparent cavity (c) with a relatively clear interface has been formed. At this time, obvious backflow phenomenon (d) can be observed at the tail of the cavity. From the moment t0 + 3 s, under the influence of gravity, the tail of the cavity floats upward (see the green line in Figure ). So that part of the vehicle is not covered by vapor, which increases the wet area. Over time, the cavity length increases steadily until t0 + 5 s, when the length of the cavity basically does not change, and it is closed at the tail of the model. At this point, the backflow phenomenon (d) can still be obviously seen at the tail of the cavity.
The right side of Figure shows the results of the numerical simulations. It can be seen that the shape and development of the ventilated cavity in the simulations are highly consistent with that in the experiments, which proves the correctness and effectiveness of the numerical simulation method from the morphology perspective.
Note that both the experimental and the simulated results show an upward shift of the cavity body as it extends downstream. As in the present flow conditions, the lower surface velocity of the cavity is larger than the upper surface velocity due to gravity. The velocity difference creates a circulation, which causes the cavity to deform (Wu et al., Citation2019). The internal pressure in the major portion of the supercavity is primarily governed by the hydrostatic pressure of water (Cao et al., Citation2017). A lower Fr (corresponding to lower freestream velocity) leads to a larger change in pressure. As Fr increases, the cavity deformation relative to the length of the underwater vehicle gets less until it becomes insignificant.
2.4. Drag reduction characteristics of ventilated cavitation
Figure shows the drag of the test model measured by the three-component strain gauge balance at a water velocity of 8 m/s and volumetric air flow rate of 30 L/min. The sampling frequency of the three-component strain gauge balance is 1 × 103 Hz. The drag data within 14 s during ventilation were obtained after averaging every 200 sampling points. Before t = 4 s, there is no ventilation in the test section; therefore, the drag data represents the model drag in the fully wetted state. When t = t0 = 4 s began to ventilate, the drag decreased significantly. Within about 2 s, the drag first fell to the lowest value and then rose while we saw a backflow occur downstream of the model by the cavitation-generated bubbles might retard the flow separation in Figures and . When t = 6 s, the drag data increased from the minimum value to a mean drag of about 15.9 N and remained stable, while an interesting Kelvin-Helmholtz-like instability was observed in the simulation results at the model surface in Figure (right). Further detailed time-resolved Particle Image Velocimetry (TRPIV) experimental study should be done on this fascinating phenomenon in the future, which might be the reason that limits drag-reduction, although the cavity continues to grow longer till t = 14 s, and the enveloped area of the model always increases. The supercavitating drag reduction rate in this condition is about 30% based on the mean drag value in the current study.
3. Design of underwater vehicles
3.1. Detachable fairing design for underwater vehicles
Compared to fully wet underwater vehicles, supercavitating vehicles have significantly lower hydrodynamic drag. The main problems of the application are energy limitation and limited steering ability. Given the above problems, a detachable fairing design for underwater vehicles is proposed. Specifically, the supercavitating drag reduction method is used as an additional function to meet the requirements of ultra-high-speed navigation tasks. Underwater vehicles navigate in a fully wetted state in general. The energy consumption in the fully wetted state is much smaller than that in the supercavitating state; therefore, the underwater vehicle can travel a longer distance with the same fuel reserve. Another advantage is that the underwater vehicle can adjust the navigation position and angle in the fully wetted state so that it only needs to navigate in a straight line in the supercavitating state, avoiding the problem of poor steering ability caused by being enveloped by a cavity. When the underwater vehicle navigates in the fully wetted state, the fairing does not fall off, reducing viscous drag. When accepting ultra-high-speed tasks, the fairing falls off and exposes the internal cavitator, forming supercavity and improving sailing speed by improving propulsion force and ventilation assistance.
The detailed scheme of the detachable fairing design for the underwater vehicle is shown in Figure . Figure (a) is the general navigation of the underwater vehicle in a fully wetted state. The detachable fairing falls off when the underwater vehicle receives an ultra-high-speed navigation task, as shown in Figure (b). At this point, the cavitator is at the front, and the underwater vehicle will enter the high-speed supercavitating state by improving propulsion force and ventilation assistance, as shown in Figure (c).
3.2. Cavitators design
The cavitator needs to satisfy two requirements in the scheme mentioned above. On the one hand, the cavitator needs to be inside the underwater vehicle without interfering with the hull in the fully wetted state; on the other hand, the cavity generated by the cavitator has the ability to envelop the underwater vehicle after the detachable fairing falls off.
The shape characteristics of a cavity can be divided into three parts (Choi & Kim, Citation2021). The first part of the front cavity shape is only related to the size of the cavitator. In the second part, the main shape of the cavity is constrained by cavitator size and cavitation number. In the third part, the shape of a cavity is affected by many unsteady vortices. Because supercavitating vehicles are enveloped in cavities, the area of the cavitators at the front is the main factor in determining the drag. In order to provide the underwater vehicle with the capacity to transition from the fully wetted state to the supercavitating state and further lower the drag in the supercavitating state, the paper proposes the following design steps for the cavitator inside the underwater vehicle:
Cavitation number can be estimated according to the design indexes of the sailing depth and speed of the underwater vehicle.
Set the allowable cavity ratio and obtain the minimum cavity shape size requirements according to the shape of the underwater vehicle.
The necessary conditions of the desired size are solved from the two aspects of the maximum diameter and length of the cavity.
Determine the cavitator size and check whether the cavitator interferes with the hull of an underwater vehicle.
Verify the feasibility of cavitator design by numerical simulation or experiment.
4. Flow characteristics study
4.1. Influence of fairing on the drag
Shape design and optimization is an important procedure in underwater vehicle design, which can lower drag and save energy. The total drag Fd of an underwater vehicle in motion mainly includes pressure drag Fp and friction drag Ff. The study compares the drag of the underwater vehicle with and without the fairing to show the advantage of the detachable fairing design in low-speed navigation. The pressure drag of the underwater vehicle without the cavitator is set as Fpa, while the pressure drag of the underwater vehicle with the cavitator is set as Fpb, which is convenient for expression. Friction drag and total drag are also abbreviated in this way. The drag reduction rate for each of the three kinds of drags is set as qp, qf and qd, respectively. Table shows the drag values and the drag reduction rates without the angle of attack at a speed range of 1-5 m/s. The fairing reduces pressure drag by approximately 58%; on the other hand, the fairing increases the wetting area so that the friction drag increase by about 82%. Because the pressure drag is much greater than the friction drag, the fairing reduces the total drag by about 40%. The drag reduction results show that adding the fairing can reduce the drag of the non-cavitation state and increase the total voyage of the underwater vehicle.
Table 1. Comparison of drag with and without fairing.
4.2. Fluid simulations of elliptical disk-shaped cavitators
4.2.1. Simulations of disk-shaped cavitator
At present, the commonly used cavitators are disk-shaped cavitators and conical cavitators. Literature (Kuklinski, Citation2006) studied the drag characteristics of conical cavitators with different cone angles. The results show that the smaller the cone angle is, the smaller the drag coefficient is, and the larger the cone angle is, the larger the cavity scale will be. The cavity generated by a disk-shaped cavitator is larger and has better static stability than the cavity generated by a conical cavitator when the bottom radiuses are equal. Therefore, this study tried to use a disk-shaped cavitator as an internal cavitator. The feasibility of the scheme was verified by numerical simulation. The cavitation number σ is usually regarded as a dimensionless parameter describing the cavitation state, which is defined as.
(8)
(8) where p∞, pv, ρ, and U∞ are freestream ambient pressure, the pressure inside the cavity, fluid density, and freestream velocity. After cavitation occurs, the smaller the cavitation number indicates the cavitation process is more intense.
Figure shows the contour map of volume fraction when cavitation number σ = 0.09. In the xOz plane, the cavity generated by the cavitator is blocked by the posterior body, and the cavity is divided into two parts (a). In the xOy plane, the liquid near the cavitator is not completely vapourized (b), although the cavity is not segmented. If the radius of the disk-shaped cavitator continues to increase, the cavitator will interfere with the fairing. In order to solve this problem, an elliptical disk-shaped cavitator is designed.
4.2.2. Cavity characteristics of elliptical disk-shaped cavitators
A cavitation simulation of an elliptical disk-shaped cavitator with long axis Da = 49.2 mm, and short axis Db = 24.6 mm was carried out. In order to exclude the influence of the shape of the model on the cavity, the posterior body was a short cylinder with a small radius. The governing equation is the RANS equation based on a homogeneous VOF method. The continuity equations are solved separately in the liquid and vapor phases. Note that the natural cavitation simulation excludes the gas phase and ignores the impact of gravity; therefore, the simulated natural cavities should be symmetrical. The velocity inlet is set as 8 m/s. The pressure outlet is set as 75 kPa. The reference pressure is set as 0 Pa.
Figure shows that after a very short time (less than 0.2 ms), the elliptical disk-shaped cavitator generated a cavity in both planes. Over time, the size of the cavity kept increasing. The front contour of the cavity in the xOz plane was obviously larger than that in the xOy plane, while the tail contour was the opposite, reflecting the elliptical disk-shaped cavitator on the shape scale of the cavity. After t = 200 ms, the shape of the cavity was basically stable.
Figure shows the three-dimension (3D) cavity shape and the pressure contour of the elliptical disk-shaped cavitator. From a macroscopic point of view, the cavity generated by the elliptical disk-shaped cavitator is an approximate elliptical spheroid. Figure is the zOy plane pressure contour of the cavity generated by the elliptical disk-shaped cavitator. As shown in Figure (a), a low-pressure region appears near the cavitator, whose shape is similar to that of the elliptical disk-shaped cavitator. In the direction of Y, above and below the short axis of the cavitator, some regions of high pressure appear. Figure (b) shows that the pressure contour lines tend to be circular. Figures (c) - (d), respectively, show that pressure contour lines are convex and concave. Figure (f) shows that the pressure in the direction of Z is higher than that in the direction of Y at the tail part of the cavity.
Figure shows the 3/4 contour lines of the cavity generated by the 2:1 elliptical disk-shaped cavitator in different zOy planes. The grey elliptical object in the center is the elliptical disk-shaped cavitator. In the front area of the cavity, the contour of the cavity is similar to that of the elliptical disk-shaped cavitator in the zOy plane. The radial distance in the direction of Z is larger than that in the direction of Y, as shown in the outline of the red curve. However, the cavity scale growth rate in the direction of Z is smaller than that in the direction of Y. In the zOy plane near the half-length of the cavity, the contour of the cavity is approximately circular, such as the blue curve with 53% of the cavity length. In the second half of the cavity, the cavity scale shrinkage rate in the direction of Z is greater than that in the direction of Y, while the cavity size in the direction of Z is smaller than that in the direction of Y. From the perspective of energy, the non-rotary cavity is in an unbalanced state with a large entropy difference to drive the cavity to repair its own shape.
4.2.3. Comparison of the cavity shapes
In order to study the relationship between the shape of the cavity and the proportion of the long and short axis of the elliptical disk-shaped cavitator, three cavitators with the same inflow area and the ratio of long and short axes of 2:1, 1.5:1, and 1:1 were selected. Figure shows the cavity length generated by the three cavitators in different cavitation numbers. It can be seen from the figure that the cavity lengths of the three cavitators are basically the same. Figure shows the maximum radial distance of the cavity of three cavitators in different cavitation numbers. In order of distance from largest to smallest, they are the Z-direction of the elliptical disk-shaped cavitator, followed by the standard disk-shaped cavitator, and the minimum is the Y-direction of the elliptical disk-shaped cavitator. In conclusion, the cavity length and radial distance of elliptical disk-shaped cavitators are approximately the same as those of disk-shaped cavitators with the same inflow area.
4.3. Shape optimization and validation
The seawater pressure increases with the increase in depth. Assuming that the underwater vehicle navigates at a fixed depth of 5 m. If cavitation number σ = 0.09, the speed should be maintained at about 58 m/s. If cavitation number σ = 0.06, the speed must be maintained at about 70 m/s. The cavitator shape optimization is carried out with a cavitation number σ = 0.09 based on the current speed of underwater vehicles.
The underwater vehicle can drop the fairing and generate supercavity after speeding up in this study. The complicated transition motion state is not considered temporary. Besides, only high-speed linear motion is proposed after entering the supercavitating state. After the preliminary test, the allowable cavity ratio is set to 7%; that is, the distance between the cavity interface and the outer surface of the underwater vehicle is no less than 7% of the maximum radius of the vehicle, as shown in Figure in red dotted line.
For cylindrical underwater vehicles, it is usually necessary to consider whether the cavity length exceeds the length of underwater vehicles under the preset cavitation number. It is more important to determine whether the radial distance of the cavity in the elliptical plane (xOz plane) satisfies the minimum allowable cavity shape for the elliptical spherical underwater vehicle. Figure predicts the actual cavity shape in the xOz plane. The designed elliptical disk-shaped cavitator satisfies the requirements of minimum cavity shape, which will not interfere with the rear body of the underwater vehicle. Table lists the related design parameters.
Table 2. Design parameters, including the internal elliptical disk-shaped cavitator.
Figure shows the natural cavitation gas volume fraction contour of the underwater vehicle when cavitation number σ = 0.09. Under the constraints of the underwater vehicle hull size, the cavity generated by the elliptical disk-shaped cavitator completely envelops the underwater vehicle, which verifies the feasibility of the design scheme. Compared with standard disk-shaped cavitators, elliptical disk-shaped cavitators have better drag reduction characteristics under special size constraints.
5. Conclusion and discussion
This study proposes a detachable fairing design for underwater vehicles to reduce cavitator drag in a fully wetted state. The cavity generated by an elliptical disk-shaped cavitator is studied through numerical analyzes and experiments. The parameters of the internal elliptical disk-shaped cavitator are optimized in the scheme mentioned above.
The VOF method is used to track the interface between liquid and gas phases. Based on the RNG k-ϵ turbulence model and the Schnerr-Sauer cavitation model, the cavity generated by the elliptical disk-shaped cavitator was simulated accurately. The development of the cavity in different periods was described. It is found that the ventilated cavity shape of the numerical simulation is consistent with that of experimental photography, which shows the validity of the simulation.
A detachable fairing design for underwater vehicles is proposed, with the benefit of increasing the total voyage and avoiding the problem of difficult steering in the supercavitating state. Supercavitating drag reduction is used as an extra method to meet the requirements of ultra-high-speed navigation tasks. It is still navigating in the fully wetted state in general, which improves the total range and avoids the problem of poor steering ability caused by being enveloped by a cavity. The design steps of the internal cavitator are put forward to ensure that the internal cavitator has the ability to form a supercavity. Because the shape of the underwater vehicle used in the paper is non-rotary, rotary cavitators are not completely suitable. The morphological characteristics of elliptical disk-shaped cavitators are studied by simulation. The main notable findings are summarized below:
From the perspective of cavity morphology, the cavity section of the elliptical disk-shaped cavitator is non-circular. In the front of the cavity near the cavitator, the cavity contour on the cross-section is approximately ellipse, similar to the surface of the elliptical disk-shaped cavitator;
The cavity length of an elliptical disk-shaped cavitator is almost the same as that of a disk-shaped cavitator when they have the same inflow area. Based on these two characteristics, the parameters of the internal elliptical disk-shaped cavitator are optimized, providing a promising strategy for the issue of cavitators increasing drag in a fully wetted state.
Although some achievements have been made in this study, there are still some limitations. This study shows that the internal elliptical disk-shaped cavitator can form supercavity after the fairing falls off. The transition state between the fully wetted state and the supercavitating state will be considered, including an economic analysis of navigation. We expect this work will be significant for the design of ultra-high-speed underwater vehicles.
Disclosure statement
No potential conflict of interest was reported by the author(s).
Additional information
Funding
References
- Ahn, B. K., Jeong, S., Kim, J., Shao, S., Hong, J., & Arndt, R. E. (2017). An experimental investigation of artificial supercavitation generated by air injection behind disk-shaped cavitators. International Journal of Naval Architecture and Ocean Engineering, 9(2), 227–237. https://doi.org/10.1016/j.ijnaoe.2016.10.006
- Cao, L., Karn, A., Arndt, R., Wang, Z., & Hong J. (2017). Numerical investigations of pressure distribution inside a ventilated supercavity. Journal of Fluids Engineering, 139(2), 021301. https://doi.org/10.1115/1.4035027
- Chen, G., Shao, Q., Sun, T., & Lv, P. (2020). An experimental comparison of ventilated supercavitation with disk-shaped cavitators and elliptical disk-shaped cavitators. 2020 International Conference on System Science and Engineering (ICSSE).
- Chen, X., Lu, C., Chen, Y., & Cao, J. (2014). A numerical analysis of the influence of the cavitator’s deflection angle on flow features for a free moving supercavitated vehicle. Journal of Hydrodynamics, 26(5), 697–705. https://doi.org/10.1016/S1001-6058(14)60078-0
- Choi, J., & Kim, H. (2021). A study on practical method to estimate drag of super-cavitating underwater vehicles. International Journal of Naval Architecture and Ocean Engineering, 13, 817–832. https://doi.org/10.1016/j.ijnaoe.2021.10.007
- Delaney, K. P. (2013). Computational methods in applied sciences. Computational Methods in Applied Sciences, 29, 171–184. https://doi.org/10.1007/978-94-007-6143-8_10
- Deng, F., Xiong, W., Zhou, J., Zheng, D., Su X., & Tang, Y. (2019). Experimental study on morphological characteristics of ventilated supercavity of double disc cavitator projectile. Xibei Gongye Daxue Xuebao/Journal of Northwestern Polytechnical University, 37(1), 7. (in Chinese). https://doi.org/10.1051/jnwpu/20193710093
- Erfanian, M. R., & Anbarsooz, M. (2018). Numerical investigation of body and hole effects on the cavitating flow behind a disk cavitator at extremely low cavitation numbers. Applied Mathematical Modelling, 62, 163–180. https://doi.org/10.1016/j.apm.2018.05.026
- Fard, M. B., & Nikseresht, A. H. (2012). Numerical simulation of unsteady 3D cavitating flows over axisymmetric cavitators. Scientia Iranica, 19(5), 1258–1264. https://doi.org/10.1016/j.scient.2012.07.013
- Gieseke, T. J. (2011). Telescoping cavitator. U.S. Patent, US20090383081.
- Hu, X., Hu, Z., & Peng, H. (2017). Numerical research on shape of supercavity induced by the variable cavitator. Chinese Journal of Hydrodynamics, 32(2), 10. (in Chinese). https://doi.org/10.16076/j.cnki.cjhd.2017.02.010
- Huang, C., Luo, K., Qin, K., Li, D., & Dang, J. (2020). Performance comparison of bow and stern rudder for high-speed supercavitating vehicles. Mathematical Problems in Engineering, 8630842. https://doi.org/10.1155/2020/8630842
- Ji, B., Luo, X., Peng, X., Zhang, Y., Wu, Y., & Xu, H. (2010). Numerical investigation of the ventilated cavitating flow around an underwater vehicle based on a three-component cavitation model. Journal of Hydrodynamics, 22(6), 753–759. https://doi.org/10.1016/S1001-6058(09)60113-X
- Jiang, Y., Jeong, S., Ahn, B., Kim, H., & Jung, Y. (2019). Experimental investigation of drag characteristics of ventilated supercavitating vehicles with different body shapes. Physics of Fluids, 31(5), 0052106. https://doi.org/10.1063/1.5092542
- Kadivar, E., Kadivar, E., Javadi, K., & Javadpour S. M. (2017). The investigation of natural supercavitation flow behind three-dimensional cavitators: Full cavitation model. Applied Mathematical Modelling, 45, 165–178. https://doi.org/10.1016/j.apm.2016.12.017
- Kim, D. H., Paramanantham, S., & Park, W. G. (2020). Numerical analysis of multi-phase flow around supercavitating body at various cavitator angle of attack and ventilation mass flux. Applied Sciences, 10(12), 4228. https://doi.org/10.3390/app10124228
- Klamo, J. T., Yeager, K. I., Cool, C. Y., Turner, T. M., & Kown, Y. W. (2021). The effects of cross-sectional geometry on wave-induced loads for underwater vehicles. IEEE Journal of Oceanic Engineering, 46(3), 765–784. https://doi.org/10.1109/JOE.2020.3023320
- Kuklinski, R. (2006). Experimental studies in the control of cavitating Bodies. AIAA Guidance, Navigation, and Control Conference and Exhibit, 6443.
- Kunz, R. F., Boger, D. A., Stinebring, D. R., Chyczewski, T. S., Lindau, J. W., Gibeling, H. J., Venkateswaran, S., & Govindan, T. R. (2000). A preconditioned Navier–Stokes method for two-phase flows with application to cavitation prediction. Computers & Fluids, 29(8), 849–875. https://doi.org/10.1016/S0045-7930(99)00039-0
- Lee, S., Paik, B., Kim, K., Jung, Y., Kim, M., & Arndt, R. (2018). On axial deformation of ventilated supercavities in closed-wall tunnel experiments. Experimental Thermal and Fluid Science, 96, 321–328. https://doi.org/10.1016/j.exptherm-flusci.2018.03.014
- Li, D., Liu, S., Wei, Y., Liang, R., & Tang, Y. (2018). Numerical investigation on transient internal cavitating flow and spray characteristics in a single-hole diesel injector nozzle: A 3D method for cavitation-induced primary break-up. Fuel, 233, 778–795. https://doi.org/10.1016/j.fuel.2018.06.103
- Li, F., & Dang, J. (2016). Hydrodynamic characteristics of conical cavitator with fins. Torpedo Technology, 24(3), 172–177. in Chinese. https://doi.org/10.11993/j.issn.1673-1948.2016.03.003
- Mansour, M. Y., Mansour, M. H., & Mostafa, N. H. (2020). Numerical and experimental investigation of supercavitating flow development over different nose shape projectiles. IEEE Journal of Oceanic Engineering, 45(4), 1370–1385. https://doi.org/10.1109/JOE.2019.2910644
- Nesteruk, I. (2014). Shape of slender axisymmetric ventilated supercavities. Journal of Computational Engineering, 501590. https://doi.org/10.1155/2014/501590
- Pendar, M. R., & Roohi, E. (2016). Investigation of cavitation around 3D hemispherical head-form body and conical cavitators using different turbulence and cavitation models. Ocean Engineering, 112, 287–306. https://doi.org/10.1016/j.oceaneng.2015.12.010
- Petitpas, F., Saurel, R., Ahn, B. K., & Ko, S. (2011). Modelling cavitating flow around underwater missiles. International Journal of Naval Architecture and Ocean Engineering, 3(4), 263–273. https://doi.org/10.3744/JNAOE.2011.3.4.263
- Qin, S., Wu, Y., Wu, D., & Hong, J. (2019). Experimental investigation of ventilated partial cavitation. International Journal of Multiphase Flow, 113, 153–164. https://doi.org/10.1016/j.ijmultiphaseflow.2019.01.007
- Rashidi, I., Pasandideh, M., Passandideh, M., & Nouri, N. M. (2014). Numerical and experimental study of a ventilated supercavitating vehicle. Journal of Fluids Engineering, 136(10), 101301. https://doi.org/10.1115/1.4027383
- Savchenko, Y. N. (2001). Supercavitation-problems and perspectives. The 4th International Symposium on Cavitation, 20–23. https://resolver.caltech.edu/CAV2001:lecture.003.
- Savchenko, Y. N., Semenenko, V. N., & Savchenko, G. Y. (2019). Peculiarities of supercavitating vehicles’ maneuvering. International Journal of Fluid Mechanics Research, 46(4), 309–323. https://doi.org/10.1615/InterJFluidMechRes.v46.i4.30
- Sun, T., Chen, G., Yang, S., Wang, Y., Wang, Y., Tan, H., & Zhang, L. (2021a). Design and optimization of a bio-inspired hull shape for AUV by surrogate model technology. Engineering Applications of Computational Fluid Mechanics, 15(1), 1057–1074. https://doi.org/10.1080/19942060.2021.1940287
- Sun, T., Ding, Y., Liu, Y., & Zou, L. (2021b). Numerical modeling and investigation of the effect of internal waves on the dynamic behavior of an asymmetric ventilated supercavity. Ocean Engineering, 233(2), 109193. https://doi.org/10.1016/j.oceaneng.2021.109193
- Wu, Y., Liu, Y., Shao, S., & Hong, J. (2019). On the internal flow of a ventilated supercavity. Journal of Fluid Mechanics, 862, 1135–1165. https://doi.org/10.1017/jfm.2018.1006
- Yakhot, V., Orszag, S. A., Thangam, S., Gatski, T. B., & Speziale, C. G. (1992). Development of turbulence models for shear flows by a double expansion technique. Physics of Fluids A: Fluid Dynamics, 4(7), 1510–1520. https://doi.org/10.1063/1.858424
- Zhang, M., Tan, J., Yi, W., & Liao, X. (2016). Large eddy simulation analysis on effect of cavitator parameter on supercavity primary position. Journal of Ballistics, 28(1), 87–91. https://doi.org/10.3969/j.issn.1004-499X.2016.01.016
- Zhang, X., Wei, Y., Zhang, J., Wang, C., & Yu, K. (2007). Experimental research on the shape characters of natural and ventilated supercavitation. Journal of Hydrodynamics, 19(5), 564–571. https://doi.org/10.1016/S1001-6058(07)60154-1
- Zou, W., & Zhang, X. (2021). Shear layer on a ventilated supercavity wall. International Journal of Multiphase Flow, 135, 103504. https://doi.org/10.1016/j.ijmultiphaseflow.2020.103504