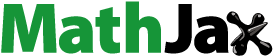
Abstract
Precessing vortex rope (PVR) plays a key role in inducing hydraulic resonance in Francis turbines operating at partial load, possibly degrading power plant stability and availability. Air injection into the runner cone is a suitable mitigating alternative; however, the influence mechanism of air injection on PVR remains unclear. The principal objective of this study was to establish response relationships between the characteristic parameters and air injection by the method of computational fluid dynamics (CFD) considering the fluid components of water, water vapour and air. The findings show that cavitation flow can be completely suppressed by slight air injection; however, the helical vortex structures are persisted at 1.0% and 2.0% air volume fractions, and the static pressure recovery is improved together with a slight increase in the hydraulic loss. At 3.0% air volume fraction, the vortex structure completely disappears, leaving an umbrella-shaped structure, with no pressure vibration arising in the turbine. Moreover, the physical mechanism of reducing the pressure amplitudes is clarified. This results clarify the influence mechanism of air injection on PVR, and contribute to steadily extending the flexibility of the operating range of the turbine during the engineering application.
1. Introduction
Hydropower plays a preponderant role in the process of tremendous development of renewable energy resources and their integration into existing power grids due to its inherent flexibilities in load variation, start-up and shutdown (Nakkina et al., Citation2016; Picone et al., Citation2021; Presas et al., Citation2019; Sun et al., Citation2021). The Francis type of turbine, which provides the advantages of a high-efficiency ratio, wide application heads and a compact structure, covers over 60% of the installed hydropower capacity worldwide (Pasche et al., Citation2019). However, a precessing vortex rope (PVR) is developed and grows in the draft tube of a Francis turbine under partial load conditions owing to fixed pitch blades that create residual swirls at the runner outlet (A. Favrel et al., Citation2015; Goyal et al., Citation2018; Iliescu et al., Citation2008). PVR is one of the most significant flows that cause several adverse effects on turbine performance, even putting the turbine stability and availability at risk (Li et al., Citation2021; Sotoudeh et al., Citation2020; A. Yu et al., Citation2021; Yu et al., Citation2022); thus, the physical mechanism of hydraulic instability toward PVR as well as its alleviation and control have received considerable critical attention among researchers and designers.
Computational fluid dynamics (CFD) is known as a technological tool related to simulation, design and optimization, which can provide all flowing details of interest in the hydraulic turbine or pump, and effectively fill the gap that the experimental test is failed under complex or limiting operation conditions (Espinoza-Jara et al., Citation2022; Liu et al., Citation2021; Zeng et al., Citation2022). Therefore, CFD is widely used to predict cavitation and vortex flowing, pressure fluctuation, as well as a hydraulic response during the transient processes(Cheng et al., Citation2021; Diab et al., Citation2022; Jiang et al., Citation2022; Wang et al., Citation2021, Citation2022), in combination with experimental test. The axial and tangential velocity profiles at the outlet of the runner are the most important driving factors affecting the formation of the PVR in the draft tube. Nishi (Citation1984) decomposed the PVR into synchronous and asynchronous components to describe the motion intensity in the axial and tangential directions, in which the synchronous component represented the PVR plunging and the asynchronous component the PVR rotating. Goyal et al. (Citation2017a, Citation2017b) carried out multiple experimental investigations into load rejection for a high-head Francis turbine and reported that the synchronous component of pressure and velocity fluctuations appeared before the asynchronous component during the PVR formation, and the amplitudes of the former were higher than those of the latter. Duparchy et al. (Citation2015) demonstrated that the asynchronous component of the PVR was the excitation source of hydraulic resonance, while the synchronous component has little influence on the runner even in the case of hydraulic resonance. The PVR was found to be significantly characterized by a large-scale vortex structure with a helical shape and increasing eccentric distance from the draft tube cone to the elbow section, according to experimental visualization in a reduced-scale physical model test of a Francis turbine with a discharge between 30% and 80% of the best value. The PVR was also found to be a leading cause of inducing local low pressure in the draft tube, and PVR evolution is generally accompanied by steep pressure growth and decline, causing marked pressure fluctuations within a frequency range of 0.2–0.45 times the runner frequency. An experimental test of pressure fluctuations within a high-head Francis turbine was conducted by Goyal et al. (Citation2016) at a 50% rated load. The test showed that the pressure fluctuation predominated a vortex rope frequency equal to 1/3 the rotation frequency, and this frequency was detected upstream of the spiral casing inlet, which indicated that the pressure fluctuation caused by the PVR could spread to the runner upstream and thus lead to unfavourable consequences for the whole hydraulic power system. Gao et al. (Citation2018) noted that the highest pressure amplitude found in the runner was attributable to superposition of the pressure fluctuations excited by the PVR and the vortex flow at the leading edge of the runner, according to their pressure fluctuation measurements for a reduced scale model of the 1000 MW Francis turbine in the Baihetan power station. Favrel et al. (Citation2017, Citation2016) highlighted that PVR as the excitation source for a hydraulic system, and its intensity was determined by the vortex trajectory, precession frequency and vortex circulation extracted by the PVR.
The PVR is a major excitation source in a hydraulic power system and severely compromises the stability of the turbine. To extend the flexibility of the operating range and guarantee a smooth flow in the turbine, PVR control and alleviation has received increased attention, with progress achieved in recent years. PVR formation and evolution both involve the stagnation and reverse flow regions in the draft tube cone; therefore, control and alleviation of the PVR has been addressed through elimination of these two singular flow regions. Ancillary fluid injection technology, which generally includes air injection and water injection, has exhibited some promise and potential in PVR mitigation and improving flow instability in the draft tube (Javadi & Nilsson, Citation2017; Kumar et al., Citation2021; Masoodi & Goyal, Citation2021; L.-g. Sun et al., Citation2020).
Bosioc et al. (Citation2012) designed an auxiliary hydraulic circuit to provide power to water injection from upstream of the runner to the draft tube cone in a conical diffuser, and the experiments showed that the amplitude and dominant frequency of the pressure fluctuations associated with the vortex rope both significantly decreased. Khullar et al. (Citation2022) numerically examined the influence of water injection into the draft tube in a Francis turbine on the flow characteristics at partial loads. The results showed that the reverse flow region was shortened by increased axial momentum, and the optimum jet was obtained with 5% of the turbine discharge under PVR conditions; however, an abrupt increment in synchronous and asynchronous components of the pressure fluctuation amplitude was observed at higher injection rates. Foroutan and Yavuzkurt (Citation2014a, Citation2014b) presented a significant analysis and discussion on the control of PVR by water injection into the runner crown cone, jet discharge was supplied from upstream of the spiral casing. The investigation found that the loss coefficient in the draft tube was decreased by 14% at a 70% best efficient point (BEP) flow rate, and the shear strength between the stagnant region and high-velocity outer flow was significantly reduced by an increased axial momentum and decreased wake region of the crown core, which ultimately led to PVR elimination. Water injection into the runner cone and peripheral water injection from the draft tube cone in a Francis protype turbine were numerically examined by Altimemy et al. (Citation2019) with large eddy simulations (LES). A comparative analysis found that the pressure fluctuations amplitude was decreased by 75% at a 6% volumetric rate of central water injection, which was superior to peripheral water injection under the same conditions. Khullar et al. (Citation2018) suggested that the pressure fluctuation of the PVR is significantly affected by the axial water injection size. Similarly, an optimum water injection rate in conjunction with fixed axial momentum was proposed by Jafarzadeh Juposhti et al. (Citation2021) for the Francis99 turbine. Numerical investigation with the scale-adaptive simulation–shear stress transport (SAS-SST) turbulence model identified that low-velocity water injection from the runner cone can provide more effective and efficient PVR mitigation. Tanasa et al. (Citation2019) reported that the amount of water injection required for mitigating PVR-induced pressure fluctuations was 10% to 12% of the main flow, which, however, was not adequate to thoroughly eliminate the low-frequency pressure oscillations. To efficiently mitigate the PVR and associated low-frequency oscillations, a new countermeasure of pulsating water injection along the draft tube axial was developed, and it was demonstrated that this solution was capable of PVR elimination with an 80% drop in the pressure amplitude.
Air injection into the hydraulic turbine is another good alternative to avoid inducing cavitation flow, reduce vibration and even enhance the runner fatigue life (Unterluggauer et al., Citation2019). In experimental investigations performed by Platonov et al. (Citation2020), air was injected into the fittings installed on the spiral casing wall on a medium-scale Francis turbine. The findings illustrated a marked rise in the pressure fluctuation amplitude adjacent to the optimal regions, while there was a significant drop at partial loads between 30% and 60% of the rated power at 1.0% air discharge, even though vortex structures were still recorded by high-speed video. Qian et al. (Citation2007) reported that the pressure difference in the cross-section of a draft tube could be reduced with air injection into the Francis turbine, which decreases the amplitude of pressure fluctuations at low frequency but increased the blade-frequency fluctuations. Three strategies, including air injection into the runner cone, installation of a fin on the inlet section of the draft tube cone and air injection through a fin, were used to clarify the suppression effects in the numerical investigation of Zhu et al. (Citation2021). They concluded that a sufficient air supply could change the helical vortex rope into a columnar rope and reduce the pressure amplitudes, while the installed fin introduced an extra vortex rope that interacts with the main vortex rope and shortens the main vortex rope. In addition, the vortex rope and the associated pressure amplitude were notably reduced in the inlet cone of the draft tube, but a stronger pressure fluctuation was observed in the draft tube elbow. Luo et al. (Citation2017) stated that air injection from the main shaft centre with a suitable air flow rate could change the appearance of the vortex rope and reduce the pressure fluctuation amplitude, which was driven by changes in the vorticity distribution in the draft tube. Numerical investigations with the SST k-ω turbulence model and two-phase model performed by Mohammadi et al. (Citation2019) suggested that air injection was the most effective measure to reduce the vorticity effects in the draft tube of a prototype Francis turbine operating at points greater than 70% of the design discharge compared to the methods of water injection or combined air and water injection. However, the combined injection performed better than either water or air injection alone at the remaining operating points. Khullar et al. (Citation2021) compared the effectiveness of air injection and water injection to suppress PVR and associated pressure fluctuations and reported that both methods could decrease the pressure oscillation strength. However, the mitigation of the pressure amplitude by water injection was attributed to a decrease in the central reverse flow region in the draft tube, whereas this mitigation stemmed from the interaction of air with the PVR for the countermeasure with air injection. In addition, pressure recovery in the draft tube declined upon air injection, but improved upon water injection. The influence of air injection together with two anti-swirl fins installed in the draft tube on the flow characteristics of a model Francis turbine was numerically investigated by Kim et al. (Citation2021). The analysis indicated a rise in the head loss with increasing air discharge, and an approximately 55% decrease in the pressure fluctuation amplitude was obtained with an injection level of 0.5% turbine discharge. In their experimental analysis of air injection for a model Francis turbine, Nakashima et al. (Citation2014) found that the pressure fluctuation disappeared at 1.0% air discharge relative to the turbine discharge; however, the efficiency of the turbine was decreased by 3.0% at this air flow rate. To increase the dissolved oxygen level downstream of the hydropower plant, air was injected into the draft tube cone through a perforated plate installed on the inner wall of the cone, in an experimental test of Bunea et al. (Citation2021). The results showed that this aeration system had no negative effects on turbine performance.
In summary, both air injection and water injection play a critical function in alleviating the PVR and the associated flow instability. Water injection requires relatively strong water discharge, which is incapable of eliminating the unsteady fluctuations and even tends to induce extra unstable flows. The countermeasure of air injection can improve the hydraulic stability with less air discharge under the operating conditions where the PVR is generated; however, most of the work carried out on air injection has failed to consider the cavitation effect that is closely linked to the PVR appearance as well as its induction and evolution in the draft tube, and the influence mechanism of air injection on the PVR has yet to be understood.
In the present paper, an unsteady numerical investigation of the influence of air injection on the PVR is conducted on a reduced-scale model of a Francis turbine under cavitation conditions, which includes the fluid components of water, water vapour and air. The PVR appearance in numerical simulations is validated with experimental visualization, and the PVR evolution and associated pressure fluctuations in the time and frequency domains are analysed. The vortex structure and swirl strength with respect to the air discharges are examined and compared, and the response relationship of pressure recovery and pressure loss to air injection is established. Furthermore, this paper makes a quantitative comparison of the pressure fluctuation amplitudes at the investigated air discharges, and the influence mechanism of air injection on the amplitude is clarified. This study offers some important insights into the influence and alleviation effect of air injection on the PVR and flow instability, which makes a major contribution to improving the operational stability at partial loads of hydraulic turbines.
2. Numerical model and schemes
2.1. Model parameters of Francis turbine
Numerical investigation and experimental test are implemented on a low-head model Francis turbine (see Figure ). The scale ratio of the model to prototype is 1:16 and the specific speed nskW is calculated as 0.196 according to Equation (1). The turbine components include the spiral casing, stay vane, guide vanes, runner, and elbow-type draft tube along with the flow direction. The rated water head and output power for the prototype turbine are 48.0 and 123.8 MW, respectively. As shown in Figure , the model runner diameter measured at the outlet is 0.37 m, the length and height of the draft tube are respectively1.767 and 0.919 m. Operating at the BEP, the peak efficiency of the model turbine is 92.7% at a guide vane opening α = 26.0°, and the corresponding unit speed n11 and unit discharge Q11 written as Equations (2) and (3) are respectively 67.0 r/min and 0.96 m3/s.
(1)
(1)
(2)
(2)
(3)
(3) where n refers to the rotational speed, r/min; H represents the operating water head, m; PkW accounts for the output power of the turbine, kW; D refers to the runner diameter, m. Q is the discharge through the turbine, m3/s; g represents the acceleration of gravity, m/s2.
The operating point of the PVR on the model efficiency hill chart is plotted in Figure , where the normalized unit discharge and speed are reported on the abscissa and ordinate, which are made nondimensional with the unit discharge and speed at the BEP and are sufficient to distinguish the relative relationship of the selected point under investigation to the BEP, as shows in Equations (4) and (5). In terms of the information in Figure , the point of interest operates with a guide vane opening of 25.3°, and the relative unit discharge Q11re and relative unit speed n11re are 92.49% and 117.78%, respectively, providing a 70% rated output power within the partial load zone.
(4)
(4)
(5)
(5)
2.2. Numerical schemes and boundary conditions
The commercial code ANSYS CFX is used to perform the numerical investigations, and the SST k-ω turbulent model is used to complement the unsteady Reynolds-averaged Navier–Stokes (URANS) equation, and the automatic near-wall treatment is activated to automatically switches from wall functions to a low-Re near-wall formulation as the mesh is refined (Botan et al., Citation2021; Menter, Citation1994; Mulu et al., Citation2015; Sun et al., Citation2021). According to the properties of the fluids during the numerical simulation, the investigations are implemented in two stages. The first stage refers to the continuous fluids of water and water vapour under the framework of a homogeneous multiphase model, where the turbulent field is shared by all fluids and the flow behaviour of the PVR in the draft tube case is solved by coupling the SST k-ω turbulent model and the Zwart-Gerber-Belamri cavitation model (L. Sun et al., Citation2020; Zwart et al., Citation2004). In the second stage, an extra dispersed fluid of air with a mean diameter of 0.0001 m is introduced into the homogeneous multiphase model presented in the first stage, which sheds light on the air admission influence on the vortex structure. Different from the first stage, the slip velocity of the dispersed air relative to the continuous water and water vapour is significant and cannot be ignored.
A constant mass flow Qm = 688.0 kg/s is specified at the inlet of the spiral casing for the inlet boundary condition, and a static gauge pressure P2 = 60.8 kPa is set at the outlet of the draft tube. During the unsteady simulation, the interface of ‘Transient Rotor Stator’ was used to handle the relative movement between the rotating runner and static components. No-slip and smooth wall conditions are used to treat all solid walls, where the influence of shear stress is considered and the flow is always static. The high-resolution scheme is activated for the advection term, and second-order backward Euler scheme for the transient term. The unsteady time step is set to the duration corresponding to 1.0 degree of one runner rotation, and a maximum of 15 iterations per time step is set to reduce all maximum residuals to below 10−3.
2.3. Grid generation and independence test
The simulation domain is numerically discretized by the pure hexahedral grid provided in the grid generation tool ICEM-CFD. Figure shows the grid generation for different components. To provide sufficient resolve to the blade or vane surface and the boundary-layer region, a considerably fine O-H type grids is applied in this paper.
The grid independence test performs a critical function in checking the influence of the grid density on the simulation precision. In the presented paper, five sets of girds with different densities are used to carry out the independence test under BEP operation in steady and fully wetted states. Two indicators, namely the hydraulic efficiency and runner torque, are frequently selected to ascertain the prediction trend during the grid independence test, which is documented in References (Botan et al., Citation2021; Diab et al., Citation2022; Jafarzadeh Juposhti et al., Citation2021; Khullar et al., Citation2022; Liu et al., Citation2021). Furthermore, the presented paper focuses the PVR that is affected significantly by the flowing in the runner and the draft tube downstream. Therefore, the minimum pressures are selected extra to test the influence of grid density on the flowing in the runner and draft tube and further validate the grid independence. The indicators of interest with respect to the grid density are shown in Figure , where the grid cell is gradually increased from 6.64 million to 15.0 million. A remarkable increment in efficiency and torque clearly is observed under the condition that the grid cell number is less than 10.79 million, while an opposite trend is presented for the minimum static pressures in the runner and draft tube. However, as noted in Figure , the further increase in grid above 10.79 million cells has almost negligible effect on the predicted results, but with more computational expense. Therefore, the third set of girds is used to performed the numerical investigations. In order to further evaluate the grid reliability, the distribution of y+, which is defined as the dimensionless distance between the first layer of the grid and the solid wall, is revealed in Figure . It is obvious that the maximum value of y+ is 10.9 on the suction side of the runner blade, and 13.9 in the vicinity of the drat tube inlet, respectively, which is applicable to the SST k-ω turbulent model.
2.4. Experimental validation
Energy and visualization experiments are performed on a test rig of a reduced-scale model Francis turbine (see Figure ). The test rig comprises four parts, namely, the foundation structure, electric drive control system, measurement system and visual observation system. The experimental apparatuses are assembled on two floors, where the bottom floor accommodates the water reservoir and pumps that supply recycled water for the experimental system. The model turbine under test, high-head tank, low-head tank and flowmeter calibration unit are installed on the top floor. A steady inlet velocity condition is created by the high-head tank, and the low-head tank is used to guarantee the pressure level downstream of the turbine cell and control the cavitation conditions. The test water head is kept at 30.0 m, and the appearance of PVR in the draft tube is recorded by a high-speed camera. All measurements are performed according to the IEC60193 standard during the experimental campaign (Commission, Citation1999). According to Equations (6) and (7), the maximum random uncertainty fr and systematic uncertainty fs towards the hydraulic efficiency are ±1.0% and ±0.214%, respectively, which provide sufficient accuracy.
(6)
(6)
(7)
(7) where t0.95(N-1) represents the T distribution under the criterion with 95% confidence coefficient and (N-1) degree of freedom; N refers to the number of repeated measuring; η corresponds to the hydraulic efficiency; the subscripts i and ave correspond to the ith measuring and the mean efficiency; fR, fQ, fT and fH respectively quantity the systematic uncertainties in rotating speed, discharge through the turbine, runner torque and water head level.
The hydraulic efficiency and water head measured by experimental investigation are respectively 87.2% and 30.09 m, and the corresponding numerical solutions respectively 88.3% and 30.24 m, which obtains a slight discrepancy within 2.0% between the numerical simulation and experimental test. Furthermore, PVR appearance as well as the relative location from numerical snapshot and the experimental visualization are comparable in Figure . In any case, the PVR behaviours and the characteristic parameters including the levels of efficiency and water head are well validated by experimental test, which is inclined to provide numerical results with higher confidence and robustness.
3. Characteristics analysis of PVR
3.1. Evolution of PVR
Regarding the vortex rope under the partial load condition, the most prominent features are its helical shape and precession movement in the draft tube cone. Figure reveals the vapour volume in the time and frequency domains, which is widely used to describe the intensity of the vortex rope, where N denotes the number of revolutions and f/fn is the normalized frequency. What stands out in this figure is the quasiperiodic increase and decrease in the vapour volume, which indicate that the evolution process of the PVR is associated with the vortex generation, development and collapse with respect to the runner rotation. The frequency domain information obtained from the fast Fourier transform (FFT) shows that the most powerful vibration amplitude of the vapour volume occurs at a low frequency of 0.3fn, which falls into 0.2–0.45 times the rotation frequency that features the frequency range of the PVR and implies that the movement of the vortex rope lags behind that of the runner.
To further provide important insights into the evolution process of the vortex rope, Figure shows the appearance of the PVR at five time instants over one oscillation period, the instants of interest are shown in Figure . At time instant t1, the vortex rope features a twisted helical shape, and the eccentricity increases along the axial direction. Enlargement of the vortex rope radius is visible at time instant t2, and the axial dimension and eccentricity are comparable to those at t1. The vortex volume reaches a peak at t3, and there has been a marked growth in the radius of the predicted vortex rope. However, the vortex rope shortens in the axial direction at the peak time instant. A slight decrease in the vortex volume is observed at time instant t4, and the vortex structure shares a number of key features with that at t3. Subsequently, the vortex volume sharply drops, with a slender appearance, at t5, and the next evolution period begins. In addition, the vortex rope is always attached to the runner cone and keeps a twisted helical shape during one evolution period.
3.2. Time–frequency characteristics of pressure fluctuation
The temporal and spatial evolution of the PVR in the draft tube has a noticeable effect on the velocity and pressure fields. Figure shows the time–frequency pressure fluctuations at three monitoring locations SC, VL and DT, where SC is upstream of the spiral casing, VL is in the vaneless space between the guide vane and runner and DT is on the wall of the draft tube cone immediately downstream of the runner outlet, as shown in Figure . The amplitude of the pressure fluctuation A is evaluated according to the 97% confidence level and defined as follows:
(8)
(8) where ΔH denotes the pressure fluctuation amplitude with a 97% confidence level, m, and H is the test head, m.
According to the time domain results in Figure , the pressure fluctuations extracted from the numerical results show a remarkable periodic oscillation of the pressure signals captured at the three points of interest. Due to the influence of the rotor stator interaction (RSI) between the guide vane and the rotating runner, the pressure wave pattern of VL is dense. The amplitudes of the pressure fluctuations in the draft tube cone, vaneless space and spiral casing inlet are as high as 17.7%, 14.9% and 12.6%, respectively, which reveals a gradual decline in the pressure amplitude from downstream to upstream of the runner during the PVR evolution. The most dominant frequency for all pressure locations in Figure is 0.3 fn, which is equal to the vibration frequency of the vapour volume described in Figure , which indicates that the pressure fluctuations induced at partial load are closely related to the PVR. Furthermore, the pressure amplitude at 13 fn, which is the blade-passing frequency and considered the second dominant frequency for VL, is slightly lower than the first dominant frequency. The aforementioned low-frequency and high-amplitude pressure fluctuations associated with the PVR can develop dynamic stresses and hydraulic resonance and degrade multiple aspects of the machine performance.
4. Alleviation of PVR with air admission
Air injection into the hydraulic turbine is a good alternative to avoid undesirable PVR as well as its adverse consequences. In the presented paper, air is introduced to the centre of the runner cone, as the blue dotted line shown in Figure , where the air is injected into the draft tube and interferes with PVR attaching to the runner cone. Numerical investigations are successively carried out at the air free, 1.0%, 2.0% and 3.0% air volume fractions that represents the ratio of air discharge to water discharge at BEP.
Air admission interferes with the flowing in the draft tube, and clarifying the effect mechanism of air admission on the turbine is critical. Average vapour volume with respect to the air volume fraction is reported in Figure , which indicates that the cavitation flow is completely suppressed at the minimum air injection of 1.0%, as is 2.0% or 3.0% air injection, thereby creating an air–water mixture flow in the draft tube.
Figure compares the predicted vortex structures at air free condition and vortex structure accompanied by the air streamlines from 1.0% to 3.0% air injection conditions in the draft tube. The vortex structure is highlighted by the velocity invariant Q = 8000 s−2 that defines a vortex as a region where the vorticity magnitude is greater than the rate-of-strain magnitude and the local pressure is lower than the surrounding pressure. Under cavitation or air-free conditions, an intensified vortex rope with a helical shape is identified by the velocity invariant Q (Hunt et al., Citation1988; Zhang et al., Citation2018). The vortex structure differs from the cavitation structure plotted in Figure in a number of important ways. First, two kinds of free vortex structures are displayed in the draft tube, namely, the helical vortex rope attached to the runner hub and a single vortex structure beneath it. Second, the vortex volume and cavitation volume are respectively 0.0080m3 and 0.0014m3, where the former is significantly larger than the latter. Finally, extra vortex structures are captured at the inlet of the draft tube and on the medial side of the elbow due to the increased magnitude of vorticity near the solids and trailing edge of the runner blade.
Figure 13. Vortex structure at air free condition and vortex structures accompanied by the air streamlines from 1.0% to 3.0% air injection conditions. (a)Air free (b)1.0% air injection 2.0% air injection (d) 3.0% air injection.

Under the condition with 1.0% air injection into the runner cone, the appearance of the helical vortex rope is maintained, and the vortex strength seems to be comparable to that in the air-free condition. One interesting finding is the motional locus of the air in the draft tube, which primarily shows three categories in the draft tube cone section, elbow section and diffuser section. The air injected into the draft tube is strictly confined to the vortex rope region and almost follows the vortex rope movement in the draft tube cone section, which is attributed to the local low pressure caused by the vortex rope. However, the air path travelled in the elbow section is very special and complex. The vortex structure shrinks to a certain extent in the vicinity of the medial side of the elbow section owing to the interference effect of air on water, and the air is released to the outside of the vortex rope and partly surrounds the vortex structure at the location of vortex shrinkage. The air is finally released to the outlet of the draft tube in the diffuser section, where the air flows adjacent to the top surface of the diffuser due to the effect of gravity.
Increasing the quantity of air injection to 2.0% results in some disordered and discrete vortex structures in the elbow section, although the helical vortex rope in the cone section is well maintained. In addition, the vortex rope is nearly cut off by the injected air at the outlet of the draft tube cone section. The constraint of the vortex rope on air becomes less significant in the cone section, which is confirmed by the air streamlines beyond the vortex rope region and the weaker helical strength in the air. Meanwhile, the air is significantly concentrated close to the inlet of the elbow section where the vortex rope is cut off. Similar to the condition with 1.0% air injection, the air in the diffuser section travels downstream near its top surface. The vortex structures and air streamlines at 3.0% air injection are different from those in the rest of the operating conditions in a number of respects. Figure (d) reveals a steep decrease in the vortex volume. The vortex rope with a helical shape is completely suppressed in the draft tube, leaving an umbrella-shaped vortex structure near the inlet of the cone section, as well as a free vortex in the elbow section. The injected air, which travels through the umbrella-shaped vortex and then surrounds the surface of the vortex structure, is subjected to an abrupt change in flow direction. The air streamlines clearly scatter around and dominate the centre of the draft tube cone and elbow sections. More air injection into the runner cone leads to a stronger mixing of water and air, which can result in less air aggregation in the diffuser section.
In summary, the appearance and strength of the vortex rope with a helical shape are approximately maintained under the conditions with less air injection, and the air is strictly confined to the vortex rope region in the draft tube cone section. The interference intensity of the injected air on flowing in the draft tube is a significant factor affecting the appearances of vortex structure. With increasing air quantity, the helical vortex rope is cut off, creating some discrete vortex structures in the diffuser section, which causes the aggregated air to be released to the outside of the vortex structure. At the maximum air injection of 3.0%, the helical vortex structure is almost completely destructed due to intensified interference effect of air on the flowing in the draft tube, and an umbrella-shaped vortex structure is formed near the inlet of the draft tube. Simultaneously, the constraint effect of the vortex structure on the air path becomes weaker due to suppressed vortex structure at 3.0% air injection, which leads to a diffusible distribution of air streamline and dominates the centrr of the draft tube cone and elbow sections. Correspondingly, the air undertakes a steep change in velocity direction by travelling through the umbrella-shaped vortex and inversely surrounding the vortex structure.
To provide a quantitative comparison of the vortex strengths under different air injection quantities, Figure plots the vortex volume extracted from the draft tube described in Figure . The variable of the vortex volume is defined as follows:
(9)
(9)
Figure reveals that the vortex volume highlighted by the velocity invariant Q decreases with increasing air volume fraction, especially under the condition with higher air injection, which further shows that the vortex strength is inversely proportional to the air volume fraction. Compared to the air-free condition, the calculated vortex volume decreases by 8.4%, 36.4% and 74.4% at air volume fractions of 1.0%, 2.0% and 3.0%, respectively.
The hydraulic efficiency and hydraulic loss respectively in the runner and draft are shown in Figure . Especially notable is the gradual drop in the hydraulic efficiency and slight growth of the hydraulic loss in the draft tube within the air volume range below 2.0%. However, a steep decline in the efficiency and increase in the draft tube loss are observed at air volume fraction above 2.0%. The influence of air admission on the hydraulic loss in the runner becomes less significant compared to the draft tube owing to the air injection location downstream of the runner.
Figure 15. Hydraulic efficiency and hydraulic loss in the runner and draft tube with respect to the air volume fraction.

The draft tube plays a decisive role in energy recovery by converting the kinetic energy into pressure energy downstream of the runner. Air injection into the draft tube affects the performance in several aspects, for example, reduction in hydraulic efficiency, as mentioned in Figure . To provide unambiguous details of how the air injection influences the performance of the draft tube, two variables are introduced in this paper, namely, the static pressure recovery coefficient χ and the total pressure loss coefficient ξ, which account for the capability to recover the kinetic energy and the hydraulic loss defined by Equations (10) and (11), respectively.
(10)
(10)
(11)
(11) where Ain and Aout refer to the areas of the inlet and outlet of interest in the draft tube, respectively, m2; p1 and p2 are the static pressure and total pressure, respectively, Pa; Qin is the discharge at the inlet, m3/s; and ρ is the density of water, kg/m3.
Figure shows 17 evaluation planes of the static pressure recovery coefficient χ and the total pressure loss coefficient ξ from the inlet to outlet in the draft tube. Figure shows the evaluated static pressure recovery coefficient χ and the total pressure loss coefficient ξ as a function of the dimensionless draft tube length l plotted in Figure . The draft tube operates well on the premise of a higher static pressure recovery coefficient and a lower total pressure loss coefficient.
Figure 16. Evaluation planes of the static pressure recovery coefficient χ and the total pressure loss coefficient ξ.

Figure 17. Static pressure recovery coefficient χ and the total pressure loss coefficient ξ with respect to dimensionless draft tube length.

According to the subcomponents of the draft tube, the static pressure recovery coefficient χ and total pressure loss coefficient ξ can be analysed in the draft tube cone section, elbow section and diffuser section. Figure shows that χ has a slight drop and subsequently rises in the draft tube cone section under the conditions of air-free to 2.0% air injection, while a continuous drop in χ is observed at 3.0% air injection. The predicted value of ξ monotonically increases in the cone section under all investigated conditions. The most remarkable variation in both χ and ξ is displayed in the elbow section. Here, χ first increases rapidly, then decreases steeply, and finally increases again, which is may attributed to the combined effect of variation in pressure caused by the vortex structure and the geometry features of the draft tube elbow. The variation trends of χ at 1.0% and 2.0% air injections are comparable to that in the air-free condition but with a higher value of χ; however, the predicted χ at 3.0% is completely lower than that in the air-free condition, which means that air injection within 2.0% is capable of improving the kinetic energy recovery efficiency, while excessive air injection has a negative impact on the energy recovery. The trend and relative variation in ξ are similar to those of χ, but the hydraulic loss is directly proportional to the air injection amount. The curves of ξ at 1.0% and 2.0% almost follow the air-free condition, and more significant hydraulic loss is generated at 3.0% air injection than under the rest of the conditions. One can observe a steady increase in χ and ξ in the diffuser section as a result of the suppressed vortex structure in this region.
The presented results reveal that air admission causes marked variations in the static pressure recovery coefficient χ and total pressure loss coefficient ξ in the elbow section of the draft tube, and the values of χ and ξ generally increase from the inlet to the outlet of the draft tube. The air injection quantities of 1.0% and 2.0% can improve the kinetic energy recovery together with a slight increase in the hydraulic loss, while 3.0% air injection causes rapid degradation in the performance of the draft tube by decreasing χ and increasing ξ.
The swirl intensity is one of the most significant features for evaluating the consequences of the vortex rope at partial load on the performance of the machine. In this paper, a variable named the swirl number S (Favrel, Citation2016; Gupta et al., Citation1984), which is defined by the ratio between the axial flux of angular momentum Gθ and the axial flux of axial momentum Gz as in Equation (12), is introduced to quantify the swirl intensity,
(12)
(12) where ρ refers to the water density, kg/m3; Va and Vt respectively account for the axial and tangential velocity profiles, m/s; r and R represent the radial coordinate and the radius, respectively, m.
Four elevations of interest in the draft tube cone section, as plotted in Figure and referred to as S1 to S4, are used to evaluate the swirl number by using the time-averaged axial and tangential velocity profiles obtained by numerical simulations. The variation in the swirl number with the air volume fraction is depicted in Figure at the selected elevations. The graph shows a slight decline in the swirl number S from elevations S1 to S3 under the air-free condition, while it sharply drops at the elevation of S4 compared to the rest of the locations, which implies that the most remarkable fall in S is developed adjacent to the outlet of the draft tube cone section. At the air volume fraction below 2.0%, linear decreased swirl numbers are observed with an increment of air volume fraction at every elevation, and the variation trends in swirl number at the four elevations are similar to those in the air-free condition. However, the level of swirl number is slightly higher at the S2 and S3 elevations than at S1 at 3.0% air volume fraction. In any event, air injection into the runner cone has a significant function in decreasing the swirl strength in the draft tube, and a higher air volume fraction results in a lower swirl number within the air range of this investigation.
As revealed in Section 3.2, high-amplitude pressure fluctuation reaching up to 17.7% of the test water head are induced by the movement of the PVR in the draft tube. Therefore, the influence of air injection on the pressure fluctuation characteristics is considered another important indicator to evaluate the alleviation effectiveness of this countermeasure. Figure compares the frequency domain information obtained from the FFT at different elevations of the draft tube cone section, where the pressure probes are referred to as DT01, DT02 and DT03. The highest amplitude is captured at pressure probe DT02 for the air-free condition, followed by DT01 and DT03. One of the most striking features in Figure is that significant drops in the pressure amplitude are displayed under the conditions with air volume fractions of 1.0% and 2.0%. For example, the amplitudes decline by 41.1% and 49.3% with 1.0% and 2.0% air volume fractions at DT01 and by 41.3% and 46.3% at DT02, respectively. More importantly, the pressure vibration in the draft tube cone section is completely suppressed in the condition with a 3.0% air volume fraction. The results firmly demonstrate that the countermeasure of air injection is capable of improving the hydraulic stability of a Francis turbine operating at partial loads. The variation trends of the pressure amplitude are in accordance with the vortex volumes described in Figures and , which implies that the sharp drop and even disappearance of the pressure amplitude are closely associated with the control and elimination of the vortex rope.
Clarifying the influence mechanism of air injection on the pressure fluctuation amplitude is fundamental. Figure is used to elaborate this issue by presenting and comparing the absolute pressure contours on the S2 plane in the draft tube cone section at different air volume fractions.
Figure 20. Absolute pressure contours on the S2 planes in the draft tube cone section at different air volume fractions.

A prominent area with low pressure is displayed in the air-free operating condition, which is caused by the strong PVR that inherently has a local pressure lower than the surrounding fluid. The low-pressure area rotates clockwise as the PVR moves, as indicated by the curve marked with an arrow in Figure . The pressure probe is subjected to some pressure variations resulting from the significant pressure difference between the low-pressure area and the rest of the locations. In other words, local low and high-pressure regions periodically sweep the pressure probe, which is the physical mechanism by which the pressure fluctuations are created, and a larger pressure difference leads to more intensified pressure fluctuations. Figure shows that the range of the low-pressure area is increased to a certain extent; however, the pressure level in the vortex-free region is significantly reduced. This minimizes the pressure difference between the vortex region and the vortex-free region and causes a drop in the pressure amplitude in the draft tube. By increasing the air injection volume fraction to 2.0%, the difference in the pressure levels in the vortex and vortex-free regions is further alleviated. Regardless of the low-pressure area region corresponding to the vortex structure, one can observe that the pressure levels in the S2 plane are very close to each other at 1.0% and 2.0% air volume fractions, which is an underlying factor of the pressure amplitudes being comparable for these two volume fractions. The pressure clearly distributes uniformly at a 3.0% air volume fraction, and the pressure difference on this plane, especially in the central region of the plane, is almost negligible compared to the other cases, which stems from the control and elimination of the vortex structure at this air volume fraction. Therefore, air injection into the runner cone can be concluded to have a significant function in alleviating the pressure level difference in the draft tube and minimizing the pressure difference that determines the pressure fluctuation intensity.
5. Conclusions
This study set out to explore the influence of air injection on the PVR and establish the relationship between the hydrodynamic parameters and air injection by the combined methods of numerical simulation of multiphase flow and experimental tests of high-speed photography. The primary conclusions are as follows:
The PVR quantified by vapour volume presents a quasiperiodic oscillation and is dominated by 0.3 times the rotation frequency. A remarkable pressure fluctuation reaching up to 17.7% of the test water head is captured in the draft tube cone, and the dominant frequency is consistent with the oscillated frequency of vapour volume, which indicates that the PVR movement has a significant influence on the pressure characteristics.
The hydraulic loss in the draft tube is increased by air injection, which is responsible for the reduction in the hydraulic efficiency. The static pressure recovery is increased both at 1.0% and 2.0% air volume fractions, accompanied by a slight increase in total pressure loss. In contrast, a sharp decrease in the static pressure recovery and an increase in the total pressure loss occur at 3.0% air volume fraction, which causes rapid degradation in the hydraulic performance of the draft tube.
The cavitation flowing is completely suppressed at all air volume fraction s; however, the strength of the helical vortex structure is approximately maintained at 1.0% and 2.0% air volume fractions. Furthermore, 3.0% air volume fraction leads to the destruction of the helical vortex structure disappears, and forms an umbrella-shaped vortex structure that is surrounded by the injected air.
The maximum pressure fluctuation amplitudes at 1.0% and 2.0% air volume fractions are decreased by over 40% in the draft tube cone, and there is no pressure vibration at a 3.0% air volume fraction. Air injection has an positive effect on increasing the absolute pressure in the draft tube, and a higher air volume fraction leads to a smaller pressure difference between the vortex region and the vortex-free region, which is the physical mechanism of alleviating and eliminating unfavourable pressure fluctuations.
Future research will be required to determine the influence of air injection on the PVR as well as the hydraulic performance in the turbine at different operating points where the PVR is induced, both by numerical and experimental investigations.
Abbreviations
PVR | = | Precessing vortex rope |
BEP | = | Best efficiency point |
D | = | Runner diameter (m) |
Α | = | Guide vane opening (°) |
nskW | = | Specific speed |
n11 | = | Unit speed (r/min) |
Q11 | = | Unit discharge (m3/s) |
H | = | Operating head (m3/s) |
g | = | acceleration of gravity (m/s2) |
n11opt | = | Unit speed at the best efficiency point (r/min) |
Q11opt | = | Unit discharge at the best efficiency point (m3/s) |
Q11re | = | Relative unit discharge |
n11re | = | Relative unit speed |
URANS | = | Unsteady Reynolds-Average Navior-Stokes equation |
SST | = | Shear Stress Transport |
T | = | Torque (N·m) |
η | = | Hydraulic efficiency (%) |
pmin | = | Minimum pressure |
SC | = | Spiral casing |
VL | = | Vaneless space |
RN | = | Runner |
DT | = | Draft tube |
y+ | = | Dimensionless distance of the first grid cell from wall |
N | = | Number of the runner revolution |
f | = | Relative frequency (-) |
fn | = | Rotating frequency (Hz) |
FFT | = | Fast Fourier transform |
t | = | Time (s) |
ΔH | = | pressure fluctuation amplitude with 97% confidence level (m) |
A | = | Amplitude of pressure fluctuation (%) |
lc | = | Hydraulic loss (m) |
φ | = | Air volume fraction (%) |
χ | = | Recovery coefficient of static pressure |
ξ | = | Loss coefficient of total pressure |
Vvor | = | Volume of the vortex structure (m3) |
S | = | Swirl number |
S | = | Elevations in the draft tube |
p | = | Pressure (m) |
Disclosure statement
No potential conflict of interest was reported by the author(s).
Additional information
Funding
References
- Altimemy, M., Attiya, B., Daskiran, C., Liu, I. H., & Oztekin, A. (2019). Mitigation of flow-induced pressure fluctuations in a Francis turbine operating at the design and partial load regimes—LES simulations. International Journal of Heat and Fluid Flow, 79, 108444. https://doi.org/10.1016/j.ijheatfluidflow.2019.108444
- Bosioc, A. I., Susan-Resiga, R., Muntean, S., & Tanasa, C. (2012). Unsteady pressure analysis of a swirling flow with vortex rope and axial water injection in a discharge cone. Journal of Fluids Engineering, 134(8), 081104. https://doi.org/10.1115/1.4007074
- Botan, A. C. B., Camacho, R., Tiago Filho, R. G., & C, G. L., Botan, O., & C, M. (2021). Comparative analysis for distributed generation using ultra-low head hydro, solar and wind energies. International Journal of Energy Research, 45(11), 16310–16328. https://doi.org/10.1002/er.6877
- Bunea, F., Ciocan, G. D., Bucur, D. M., Dunca, G., & Nedelcu, A. (2021). Hydraulic turbine performance assessment with implementation of an innovative aeration system. Water, 13(18), 2459. https://www.mdpi.com/2073-4441/13/18/2459. https://doi.org/10.3390/w13182459
- Cheng, H., Long, X., Ji, B., Peng, X., & Farhat, M. (2021). A new Euler-Lagrangian cavitation model for tip-vortex cavitation with the effect of non-condensable gas. International Journal of Multiphase Flow, 134, 103441. https://doi.org/10.1016/j.ijmultiphaseflow.2020.103441
- Commission, I. E. (1999). IEC 60193: 1999, Hydraulic turbines, storage pumps and pump-turbines-Model acceptance tests Geneva, Switzerland.
- Diab, G., Elhakeem, M., & Sattar, A. M. A. (2022). Performance assessment of lift-based turbine for small-scale power generation in water pipelines using OpenFOAM. Engineering Applications of Computational Fluid Mechanics, 16(1), 536–550. https://doi.org/10.1080/19942060.2021.2019129
- Duparchy, F., Favrel, A., Lowys, P. Y., Landry, C., Müller, A., Yamamoto, K., & Avellan, F. (2015). Analysis of the part load helical vortex rope of a Francis turbine using on-board sensors. Journal of Physics: Conference Series, 656, 012061. https://doi.org/10.1088/1742-6596/656/1/012061
- Espinoza-Jara, A., Walczak, M., Molina, N., Jahn, W., & Brevis, W. (2022). Erosion under turbulent flow: A CFD-based simulation of near-wall turbulent impacts with experimental validation. Engineering Applications of Computational Fluid Mechanics, 16(1), 1526–1545. https://doi.org/10.1080/19942060.2022.2099978
- Favrel, A., Müller, A., Landry, C., Gomes, J., Yamamoto, K., & Avellan, F. (2017). Dynamics of the precessing vortex rope and its interaction with the system at Francis turbines part load operating conditions. Journal of Physics: Conference Series, 813, 012023. https://doi.org/10.1088/1742-6596/813/1/012023
- Favrel, A., Müller, A., Landry, C., Yamamoto, K., & Avellan, F. (2015). Study of the vortex-induced pressure excitation source in a Francis turbine draft tube by particle image velocimetry. Experiments in Fluids, 56(12), 215. https://doi.org/10.1007/s00348-015-2085-5
- Favrel, A., Müller, A., Landry, C., Yamamoto, K., & Avellan, F. (2016). Space and time reconstruction of the precessing vortex core in Francis turbine draft tube by 2D-PIV. IOP Conference Series: Earth and Environmental Science, 49, 082011. https://doi.org/10.1088/1755-1315/49/8/082011
- Favrel, A. T. (2016). Dynamics of the cavitation precessing vortex rope for Francis turbines at part load operating conditions. EPFL. https://infoscience.epfl.ch/record/215867/files/EPFL_TH6880.pdf.
- Foroutan, H., & Yavuzkurt, S. (2014a). Flow in the simplified draft tube of a Francis turbine operating at partial load—part I: Simulation of the vortex rope. Journal of Applied Mechanics, 81(6), 061010. https://doi.org/10.1115/1.4026817
- Foroutan, H., & Yavuzkurt, S. (2014b). Flow in the simplified draft tube of a Francis turbine operating at partial load—part II: Control of the vortex rope. Journal of Applied Mechanics, 81(6), 061011. https://doi.org/10.1115/1.4026818
- Gao, Z., Zhu, W., Meng, L., Zhang, J., Zhang, F., Pan, L., & Lu, L. (2018). Experimental study of the Francis turbine pressure fluctuations and the pressure fluctuations superposition phenomenon inside the runner. Journal of Fluids Engineering, 140(4), 041208. https://doi.org/10.1115/1.4038535
- Goyal, R., Cervantes, M. J., & Gandhi, B. K. (2017a). Characteristics of synchronous and asynchronous modes of fluctuations in Francis turbine draft tube during load variation. International Journal of Fluid Machinery and Systems, 10(2), 164–175. https://doi.org/10.5293/IJFMS.2017.10.2.164
- Goyal, R., Cervantes, M. J., & Gandhi, B. K. (2017b). Vortex rope formation in a high head model Francis turbine. Journal of Fluids Engineering, 139(4), 041102. https://doi.org/10.1115/1.4035224
- Goyal, R., Gandhi, B. K., & Cervantes, M. J. (2018). PIV measurements in Francis turbine – a review and application to transient operations. Renewable and Sustainable Energy Reviews, 81, 2976–2991. https://doi.org/10.1016/j.rser.2017.06.108
- Goyal, R., Trivedi, C., Gandhi, B. K., Cervantes, M. J., & Dahlhaug, O. G. (2016). Transient pressure measurements at part load operating condition of a high head model francis turbine. Sādhanā, 41(11), 1311–1320. https://doi.org/10.1007/s12046-016-0556-x
- Gupta, A. K., Lilley, D. G., & Syred, N. (1984). Swirl flows. https://ui.adsabs.harvard.edu/abs/1984tw…book…G.
- Hunt, J. C., Wray, A. A., & Moin, P. (1988). Eddies, streams, and convergence zones in turbulent flows. (Ed.),(Eds.). Studying Turbulence Using Numerical Simulation Databases. Proceedings of the 1988 summer program, San Francisco, USA.
- Iliescu, M. S., Ciocan, G. D., & Avellan, F. (2008). Analysis of the cavitating draft tube vortex in a Francis turbine using particle image velocimetry measurements in Two-phase flow. Journal of Fluids Engineering, 130(2), 021105. https://doi.org/10.1115/1.2813052
- Jafarzadeh Juposhti, H., Maddahian, R., & Cervantes, M. J. (2021). Optimization of axial water injection to mitigate the rotating vortex rope in a Francis turbine. Renewable Energy, 175, 214–231. https://doi.org/10.1016/j.renene.2021.05.038
- Javadi, A., & Nilsson, H. (2017). Active flow control of the vortex rope and pressure pulsations in a swirl generator. Engineering Applications of Computational Fluid Mechanics, 11(1), 30–41. https://doi.org/10.1080/19942060.2016.1235515
- Jiang, L.-J., Zhang, R.-H., Chen, X.-B., & Guo, G.-Q. (2022). Analysis of the high-speed jet in a liquid-ring pump ejector using a proper orthogonal decomposition method. Engineering Applications of Computational Fluid Mechanics, 16(1), 1382–1394. https://doi.org/10.1080/19942060.2022.2089733
- Khullar, S., Singh, K., Gandhi, B., & Cervantes, M. J. (2018, December 10-12). Effect of axial water jet size and velocity on unsteady pressure pulsations in a deaccelerating swirling flow. (Ed.),(Eds.). Proceedings of the 7th International and 45th National Conference on Fluid Mechanics and Fluid Power (FMFP), Mumbai, India.
- Khullar, S., Singh, K. M., Cervantes, M. J., & Gandhi, B. K. (2021). Comparison of axial water and air injections in the draft tube of a Francis Turbine for RVR Mitigation. (Ed.),(Eds.). ASME 2021 Fluids Engineering Division Summer Meeting.
- Khullar, S., Singh, K. M., Cervantes, M. J., & Gandhi, B. K. (2022). Numerical analysis of water Jet injection in the draft tube of a Francis turbine at part load operations. Journal of Fluids Engineering, 144(11), 111201. https://doi.org/10.1115/1.4054564
- Kim, S.-J., Cho, Y., & Kim, J.-H. (2021). Effect of air injection on the internal flow characteristics in the draft tube of a Francis turbine model. Processes, 9(7), 1182. https://www.mdpi.com/2227-9717/9/7/1182. https://doi.org/10.3390/pr9071182
- Kumar, S., Cervantes, M. J., & Gandhi, B. K. (2021). Rotating vortex rope formation and mitigation in draft tube of hydro turbines – a review from experimental perspective. Renewable and Sustainable Energy Reviews, 136, 110354. https://doi.org/10.1016/j.rser.2020.110354
- Li, D., Yu, L., Yan, X., Wang, H., Shi, Q., & Wei, X. (2021). Runner cone optimization to reduce vortex rope-induced pressure fluctuations in a Francis turbine. Science China Technological Sciences, 64(9), 1953–1970. https://doi.org/10.1007/s11431-021-1867-2
- Liu, Y., Wang, D., & Ran, H. (2021). Computational research on the formation mechanism of double humps in pump–turbines. Engineering Applications of Computational Fluid Mechanics, 15(1), 1542–1562. https://doi.org/10.1080/19942060.2021.1977711
- Luo, X., Yu, A., Ji, B., Wu, Y., & Tsujimoto, Y. (2017). Unsteady vortical flow simulation in a Francis turbine with special emphasis on vortex rope behavior and pressure fluctuation alleviation. Proceedings of the Institution of Mechanical Engineers, Part A: Journal of Power and Energy, 231(3), 215–226. https://doi.org/10.1177/0957650917692153
- Masoodi, F. A., & Goyal, R. (2021). Efficacy of ancillary fluid injection technique for mitigation of vortex rope in hydraulic turbines: A review. Materials Today: Proceedings, 47, 3043–3053. https://doi.org/10.1016/j.matpr.2021.05.618
- Menter, F. R. (1994). Two-equation eddy-viscosity turbulence models for engineering applications. AIAA Journal, 32(8), 1598–1605. https://doi.org/10.2514/3.12149
- Mohammadi, M., Hajidavalloo, E., & Behbahani-Nejad, M. (2019). Investigation on combined air and water injection in Francis turbine draft tube to reduce vortex rope effects. Journal of Fluids Engineering, 141(5), 051301. https://doi.org/10.1115/1.4041565
- Mulu, B. G., Cervantes, M. J., Devals, C., Vu, T. C., & Guibault, F. (2015). Simulation-based investigation of unsteady flow in near-hub region of a Kaplan turbine with experimental comparison. Engineering Applications of Computational Fluid Mechanics, 9(1), 139–156. https://doi.org/10.1080/19942060.2015.1004816
- Nakashima, T., Matsuzaka, R., Miyagawa, K., Yonezawa, K., & Tsujimoto, Y. (2014). Study on flow instability and countermeasure in a draft tube with swirling flow. IOP Conference Series: Earth and Environmental Science, 22(3), 032007. https://doi.org/10.1088/1755-1315/22/3/032007
- Nakkina, P. R., Arul Prakash, K., & Saravana Kumar, G. (2016). Numerical studies on fluid flow characteristics through different configurations of spiral casing. Engineering Applications of Computational Fluid Mechanics, 10(1), 296–310. https://doi.org/10.1080/19942060.2016.1149103
- Nishi, M. (1984). Surging characteristics of conical and elbow type draft tubes. (Ed.),(Eds.). Proc. 12th IAHR Symposium on Hydraulic Machinery and System, Stirling, 1984.
- Pasche, S., Gallaire, F., & Avellan, F. (2019). Origin of the synchronous pressure fluctuations in the draft tube of Francis turbines operating at part load conditions. Journal of Fluids and Structures, 86, 13–33. https://doi.org/10.1016/j.jfluidstructs.2019.01.013
- Picone, C., Sinagra, M., Aricò, C., & Tucciarelli, T. (2021). Numerical analysis of a new cross-flow type hydraulic turbine for high head and low flow rate. Engineering Applications of Computational Fluid Mechanics, 15(1), 1491–1507. https://doi.org/10.1080/19942060.2021.1974559
- Platonov, D., Minakov, A., Dekterev, D., & Maslennikova, A. (2020). An experimental investigation of the air injection effect on the vortex structure and pulsation characteristics in the Francis turbine. International Journal of Fluid Machinery and Systems, 13(1), 103–113. https://doi.org/10.5293/IJFMS.2020.13.1.103
- Presas, A., Luo, Y., Wang, Z., & Guo, B. (2019). Fatigue life estimation of Francis turbines based on experimental strain measurements: Review of the actual data and future trends. Renewable and Sustainable Energy Reviews, 102, 96–110. https://doi.org/10.1016/j.rser.2018.12.001
- Qian, Z.-d., Yang, J.-d., & Huai, W.-x. (2007). Numerical simulation and analysis of pressure pulsation in francis hydraulic turbine with air admission. Journal of Hydrodynamics, Ser. B, 19(4), 467–472. https://doi.org/10.1016/S1001-6058(07)60141-3
- Sotoudeh, N., Maddahian, R., & Cervantes, M. J. (2020). Investigation of rotating vortex rope formation during load variation in a Francis turbine draft tube. Renewable Energy, 151, 238–254. https://doi.org/10.1016/j.renene.2019.11.014
- Sun, L., Guo, P., & Luo, X. (2020). Numerical investigation on inter-blade cavitation vortex in a Franics turbine. Renewable Energy, 158, 64–74. https://doi.org/10.1016/j.renene.2020.05.034
- Sun, L., Guo, P., & Luo, X. (2021). Numerical investigation of inter-blade cavitation vortex for a Francis turbine at part load conditions. IET Renewable Power Generation, 15(6), 1163–1177. https://doi.org/10.1049/rpg2.12096
- Sun, L., Guo, P., & Yan, J. (2021). Transient analysis of load rejection for a high-head Francis turbine based on structured overset mesh. Renewable Energy, 171, 658–671. https://doi.org/10.1016/j.renene.2021.02.151
- Sun, L.-g., Guo, P.-c., & Wu, L.-c. (2020). Numerical investigation of alleviation of undesirable effect of inter-blade vortex with air admission for a low-head francis turbine. Journal of Hydrodynamics, 32(6), 1151–1164. https://doi.org/10.1007/s42241-020-0081-6
- Tanasa, C., Stuparu, A., Stroita, C., Popescu, C., & Susan-Resiga, R. (2019). 3D numerical analysis of pulsating water jet in the draft tube cone of hydraulic machinery. (Ed.),(Eds.). AIP Conference Proceedings.
- Unterluggauer, J., Maly, A., & Doujak, E. (2019). Investigation on the impact of air admission in a prototype Francis turbine at Low-load operation. Energies, 12(15), 2893. https://www.mdpi.com/1996-1073/12/15/2893. https://doi.org/10.3390/en12152893
- Wang, Z., Cheng, H., & Ji, B. (2021). Euler–Lagrange study of cavitating turbulent flow around a hydrofoil. Physics of Fluids, 33(11), 112108. https://doi.org/10.1063/5.0070312
- Wang, Z., Cheng, H., & Ji, B. (2022). Numerical prediction of cavitation erosion risk in an axisymmetric nozzle using a multi-scale approach. Physics of Fluids, 34(6), 062112. https://doi.org/10.1063/5.0095833
- Yu, A., Tang, Y., Tang, Q., Cai, J., Zhao, L., & Ge, X. (2022). Energy analysis of Francis turbine for various mass flow rate conditions based on entropy production theory. Renewable Energy, 183, 447–458. https://doi.org/10.1016/j.renene.2021.10.094
- Yu, Z.-F., Yan, Y., Wang, W.-Q., & Liu, X.-S. (2021). Entropy production analysis for vortex rope of a Francis turbine using hybrid RANS/LES method. International Communications in Heat and Mass Transfer, 127, 105494. https://doi.org/10.1016/j.icheatmasstransfer.2021.105494
- Zeng, Y. S., Li, N., Wang, C. Y., Xiao, R. F., Wang, F. J., & Yao, Z. F. (2022). Resonance risk assessment method on a storage pump’s centrifugal impeller by considering the hydrodynamic damping ratio. Engineering Applications of Computational Fluid Mechanics, 16(1), 2175–2189. https://doi.org/10.1080/19942060.2022.2143902
- Zhang, Y., Liu, K., Xian, H., & Du, X. (2018). A review of methods for vortex identification in hydroturbines. Renewable and Sustainable Energy Reviews, 81, 1269–1285. https://doi.org/10.1016/j.rser.2017.05.058
- Zhu, L., Zhang, R.-z., Yu, A., Lu, L., & Luo, X.-w. (2021). Suppression of vortex rope oscillation and pressure vibrations in Francis turbine draft tube using various strategies. Journal of Hydrodynamics, 33(3), 534–545. https://doi.org/10.1007/s42241-021-0038-4
- Zwart, P. J., Gerber, A. G., & Belamri, T. (2004). A two-phase flow model for predicting cavitation dynamics. (Ed.),(Eds.). Fifth international conference on multiphase flow, Yokohama, Japan.