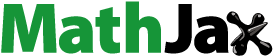
Abstract
To examine the cavitation noise characteristics of centrifugal pumps, the combination of test and simulation was applied in this study. The numerical method is based on spherical cavity radiation theory and the acoustic finite element method. A multi-field synchronized test-rig was built to study and validate the numerical results under cavitating flow and cavitation noise conditions. The three-dimensional unsteady flow-field simulation was carried out inside the centrifugal pump based on DES and the improved-RZGB cavitation model. The constructed numerical method for cavitation noise was used to solve the acoustic-field based on the flow-field solutions. The test–simulation comparison reveal that the numerical simulation results are consistent and reliable. The development of cavitation has a stronger influence on the strong vorticity regions in the impeller flow channel, and the vortex has a promotional-effect on the formation of cavitation. With the same cavitation number, the acoustic-power on the blade suction-side is greater than the pressure-side of the blade. As the cavitation number decreases, the pulsating radiated noise of cavitation volume is the primary noise-source for the increased acoustic-power value. At this stage, the SPL and total SPL at the model pump inlet show a decreasing trend during the severe cavitation-stage.
Nomenclature
= | Head (m) | |
Qd | = | Design flow rate (m3/h) |
n | = | Rotational speed (rpm) |
ns | = | Specific speed |
z | = | Blade number of impeller |
p | = | Pressure (Pa) |
= | Vapour pressure | |
T | = | Temperature (°C) |
Abbreviations
FW-H | = | Ffowcs Williams–Hawkings model |
ZGB | = | Zwart-Gerber-Belamri cavitation model |
RZGB | = | Rotating-based Zwart-Gerber Belamri cavitation model |
CA | = | Computational acoustics |
CFD | = | Computational fluid dynamics |
DES | = | Detached eddy simulation |
FFT | = | Fast Fourier transform |
SPL | = | Sound pressure level |
Greek letters
σ | = | Cavitation number |
φ | = | Flow coefficient |
ψ | = | Head coefficient |
= | Efficiency | |
ρl | = | Liquid density (kg/m3) |
ρg | = | Gas density (kg/m3) |
1. Introduction
Cavitation, a phenomenon where water changes from liquid to gas when the local pressure is less than the saturated vapour pressure, occurs during the operation of hydraulic machinery (Cao et al., Citation2022; Cheng et al., Citation2019). Intense cavitation combined with cavitation clouds results in large-scale vortex structures (Gong et al., Citation2022; Kadivar et al., Citation2020), which can cause noise (cavitation noise) and vibration in hydraulic machinery, resulting in severe fatigue and destabilisation and, in some cases, leading to complete fracture of hydraulic machinery (S. Wu et al., Citation2022). Therefore, it is essential to study the cavitation noise characteristics in centrifugal pumps.
Cavitation noise is an essential component of underwater radiated noise (URN). It reduces the ship’s security and comfort and impacts marine life (Geng & Escaler, Citation2020; L. Yu et al., Citation2022). Usually, high cavitation numbers result in only sparsely distributed transient cavitation bubbles that move along with the flow. This regime is generally steady or nearly steady. However, lower cavitation numbers give birth to other mechanisms, such as the breakup of a sheet cavity and the development of enormous vapour structures, such as cavitation clouds (Peng et al., Citation2016). These clouds are mainly responsible for generating cavitation noise as they grow and collapse simultaneously (Chen et al., Citation2015). Cavitation noise is classified into two types: tonal noise (blade rate) and broadband noise (tip-vortex cavitation). Sheet cavitation raises noise levels in the low-frequency region (i.e. tonal blade rates). The tip-vortex cavitation significantly contributes to the broadband noise at high-frequency and medium–low-frequency areas (H.-y. Cheng, Ji, et al., Citation2021; H. Cheng, Long, et al., Citation2021). It should be noted that the tonal noise levels rise when the collapsing phenomenon is present (Sezen et al., Citation2020).
The prediction of cavitation noise relies on accurate modelling of cavitation. Several studies evaluated the discrepancies in cavitation noise predicted by various turbulence models (Kamali-Moghadam et al., Citation2016). It was discovered that RANS could not estimate noise accurately because it neglects the pulsations of the quantities (Ianniello et al., Citation2013). LES is the most accurate technique to treat cavitating flow (Bai et al., Citation2022; Z. Wang et al., Citation2021), especially the LES-SGS models. However, it overestimates the dominant frequencies of the cavity oscillations, underestimates the side-wall effects, and requires an enormous number of grids and computations, making it inappropriate for dealing with complex engineering applications such as cavitation issues (Z. Li, Qian, et al., Citation2020). On the other hand, the DES turbulence model is most widely applied in forecasting cavitation noise (Hu et al., Citation2021). It can better forecast various cavitating flows, capture a broader range of turbulent scales (Kinzel et al., Citation2007), and better predict the cavity oscillations’ main frequency, which is overpredicted by the LES model (Sedlar et al., Citation2016). Therefore, an approach based on the DES model was adopted in this study, in combination with an improved RZGB cavitation model for simulating cavitating flow.
The noise generated by the pulsation of cavitation volume is a monopole sound source with strong radiation capacity (Si, Ali, et al., Citation2019). Therefore, the noise source radiated by the cavitation volume pulsation should not be neglected. The solution of cavitation-induced noise using the Ffowcs Williams–Hawking (FW-H) model area fraction formula is a common method, and (Seol et al., Citation2005) solved the monopole noise generated by the cavitation volume pulsation of the propeller based on the FW-H Farassat 1A integral formula. Still, it is more challenging to extract the normal velocity vector of the cavitation surface using this method. Reference (Wei et al., Citation2022) predicted and analysed the noise of hydrofoils in the cavitation state based on the FW-H acoustic analogy method, while (Sakamoto & Kamiirisa, Citation2018) did not use the FW-H acoustic analogy method but predicted the near-field propeller cavitation-induced noise based on the viscous CFD semi-empirical method, and the effectiveness of the method was verified by comparing it with the experimental data. Some scholars in China, such as the authors of (A. Yu et al., Citation2019) and (T. Sun et al., Citation2021), also used the FW-H area fraction formula to solve the cavitation-induced noise. Reference (A. Yu et al., Citation2019) used the improved turbulence model and Zwart–Gerber–Belamri (ZGB) cavitation model to predict the cavitation flow field accurately. Then, the FW-H acoustic analogy method was used to simulate the cavitation-induced noise. They found that the amplitude of the acoustic power spectral density increased sharply with the decrease in the cavitation number.
The experimental studies play an essential role in promoting the study of cavitation flow-induced noise, where the researchers can measure the cavitation-induced noise with the help of water cavities and centrifugal pump multifunctional test benches. Reference (Reisman et al., Citation1998) studied the dynamic and acoustic properties of cloud cavitation and concluded that the shock wave generated by the rupture of cloud cavitation is a crucial factor in determining the noise intensity and material failure. Reference (Callenaere et al., Citation2001) found that the noise and destruction produced by the complex flow formed by cavitation clouds on the surface of oscillating hydrofoils are stronger than those produced by non-oscillatory flows. Therefore, the intensity of noise can be quantified for the intensity of cavitation. Reference (Chudina, Citation2003) investigated the cavitation noise characteristics of centrifugal pumps in the early stage and found that the cavitation-induced noise spectra can be used to diagnose the initial cavitation of centrifugal pumps. The size of the SPL of cavitation-induced noise increases as the cavitation number decreases, then decreases after reaching the extreme value. The experimental studies of (ČDina, Citation2003) and (Čudina & Prezelj, Citation2009) found that a specific frequency (147 Hz) in the noise spectrum is closely related to the cavitation phenomenon of the pump, and it is more accurate in determining the cavitation of the pump than the traditional method of determining the 3% reduction in the pump head. Reference (Shin et al., Citation2021) measured the vortex cavitation and its induced noise at the leaf-top of the 3D hydrofoil, and the outcomes revealed that the SPL of the cavitation condition was significantly more extensive than that of the non-cavitation state.
In summary, several researchers have carried out plenty of research on the cavitation-induced noise in propellers and hydrofoils, mainly based on spherical cavitation radiation theory and with some reference to centrifugal pump cavitation-induced noise research. However, the acoustic characteristics of centrifugal pumps in different cavitation stages and the energy conversion mechanism between the flow field, structural field, and acoustic field inside the centrifugal pump under cavitation conditions are highly complex and have not been properly studied. Therefore, based on spherical cavitation radiation theory and the acoustic finite element method, the method of combining the CFD/CA approach was conducted to analyse the cavitation hydrodynamics and their induced noise characteristics in a centrifugal pump. A multi-field synchronous test measurement technique and modern signal processing methods were also applied to study the dynamic behaviour in different time and spatial scales and to validate the CFD-simulation results. Finally, the cavitation hydrodynamics and cavitation noise characteristics were comprehensively analysed.
2. Numerical simulation theory
In recent years, with the quick advancement of computer technology, computational fluid dynamics (CFD) (A. Ali et al., Citation2021) and computational acoustics (CA) have been widely used in the research of cavitation hydrodynamics and its induced noise characteristics of centrifugal pumps, which has the advantage of low cost and short time cycles as compared with experiments. In this study, a combination of CFD/CA was used for the acoustic simulation of a centrifugal pump. CA simulations used the spherical cavitation radiation theory to solve the monopole sound source. In contrast, the acoustic finite element method was used to solve the dipole and quadrupole sound sources. This section mainly provides the theoretical and mathematical overview of numerical simulation methods for analysing a centrifugal pump’s cavitation and acoustic characteristics and lays the theoretical foundation for subsequent numerical simulation studies.
2.1. Acoustic simulation theory of cavitation
Cavitation is a complex hydrodynamic phenomenon, and the homogeneous flow cavitation model was adopted in this study for the numerical simulation of cavitation. Its flow field and acoustic field are regulated by N-S equations (continuity, momentum, and transport equation). The N-S equations are stated as Equations (1-3), which are reordered in several shapes of inhomogeneous acoustic-wave equations. All the mathematical derivations used in Section 2 are represented in Cartesian/rectangular coordinates by following the studies of (X. Sun & Wang, Citation2021) and (Si, Shen, et al., Citation2020).
(1)
(1)
(2)
(2)
(3)
(3) where
denotes the density of the mixed phase;
denotes the dynamic viscosity coefficient of the mixed phase;
denotes the eddy viscosity coefficient of the mixed phase; and p denotes the flow field pressure, Pa.
In acoustic simulations, the direct method is to obtain the physical solution of the flow field and acoustic field by solving the N-S equations directly. This method does not need any additional acoustic model and is under the physical effect of flow-induced noise generation and propagation (See Figure for more details). However, an indirect method (called ‘acoustic analogy’) is obtained from N-S equations and refers to substituting an equivalent sound source for complex acoustic problems.
Figure 1. Generation and propagation path of cavitation- induced noise sources in a centrifugal pump.

2.1.1. Acoustic analogy and noises sources in centrifugal pump
The acoustic analogy method is based on the Lighthill acoustic analogy, in which the fluid is first regarded as incompressible to complete the hydrodynamic analysis. The CFD technique is usually used to solve the unsteady flow information of the ‘hydraulic near field’. Then, considering the fluid’s compressibility, the fluid dynamics calculation is transformed into the fluid source calculations. Finally, the acoustic field is solved by solving the acoustic wave equation. Compared with the direct method of the acoustic field, the analysis of the acoustic analogy method is relatively small. The theoretical basis of the indirect method is the Lighthill acoustic analog equation, which is derived from the N-S square path:
(4)
(4)
(5)
(5) where
is the Lighthill stress tensor;
is the fluid density;
represents the Kronecker function;
indicates fluid pressure;
indicates the undisturbed pressure of the fluid;
represents the time;
indicates the sound speed;
is the space coordinate; and
are the coordinate axis locations.
The acoustic analogy provides valuable information about the flow field, including mean velocity, pressure, and turbulent kinetic energy. The velocity fluctuation characteristics of the flow field can be obtained from available information, and then the distribution and intensity of the cavitation-induced noise sources can be predicted. DES models are used to calculate the flow field and provide accurate information on acoustic-field calculation. The obtained acoustic information is then transformed into acoustic simulation software (such as ACTRAN), and the response of the flow field at the far-field monitoring point can be calculated.
Lighthill proposed a point source method to describe the hydrodynamic noise sources in the last century, which divides hydrodynamic noise into monopole, dipole, and quadrupole sound sources (Ali, Si, Yuan, et al., Citation2022; Lighthill, Citation1954). However, the cavitation-induced noise generation mechanism in a centrifugal pump is more complex, and its sound source mainly comes from the following aspects:
(1) the monopole noise radiated by the volume pulsation and collapse of the cavitation bubbles; (2) the structural vibration radiated noise produced due to the interaction between the cavitation and fluid acting on the blades where the volute is the dipole sound source; (3) the quadrupole noise source, which is considered to be the noise produced by turbulent flow in the impeller and volute flow channel of centrifugal pumps; and (4) the strong acoustic impulses for both sheet and cloud cavitation generated by the collapse of the detached small cloud cavity downstream. The rapid pressure fluctuations caused by the strong contact between the re-entrant jet and the main flow produce severe dipole noise, explaining another characteristic method to generate intense acoustic impulses for cloud cavitation (Wei et al., Citation2022; Q. Wu et al., Citation2018).
For the centrifugal pump acoustic field, the impeller region is the ‘hydraulic near field’, which is regarded as both the sound generation and propagation area. However, the volute and the volute outlet pipe area are the ‘hydraulic far field’, regarded as the sound propagation area in a centrifugal pump. The sound source and propagation path of cavitation noise from a centrifugal pump are shown in Figure .
2.2. Acoustic simulation theory for predicting cavitation-induced noise
2.2.1. Cavitation-induced noise with no cavitation volume pulsation
The changes in the flow field pressure, velocity, and density caused by the occurrence of cavitation (dipole and quadrupole noise sources) are solved by the acoustic finite element method for cavitation-induced noise that does not consider the cavitation volume pulsation. The expression for the finite element variational scattered weak solution form of the Lighthill acoustic analog equation is as follows, which is achieved after various derivations of the above equations in Section 2.1.1 (Si, Shen, et al., Citation2019):
(6)
(6) The first term at the right side of the equation represents the volume sound source, and the second at the right side represents the surface sound source. Additionally, the cavitation-induced noise with no cavitation volume pulsation can be solved using Equation (Equation6
(6)
(6) ).
The radiation noise is described in terms of time and space. This relationship can be mathematically represented by an acoustic wave equation, the final form of which is stated as follows, which is achieved after several mathematical derivations that can be found in Reference (Si, Wang, et al., Citation2019):
(7)
(7) where
denotes the number of waves, while omega denotes the angular velocity.
2.2.2. Cavitation-induced noise with cavitation volume pulsation
The monopole noise source of cavitation volume in the flow field can be solved using the spherical cavitation radiation theory. The following briefly introduces the spherical cavitation radiation theory (Ghasemian & Nejat, Citation2015; Mohamed, Citation2016). Considering the cavitation as a simple pulsation source, suppose the cavitation bubble diameter is much smaller than the characteristic wavelength. In that case, the cavitation bubble could be considered as a point pulsation source, and the monitoring point sound pressure at distance r from the centre of the sphere is:
(8)
(8) For spherical cavitation bubbles with uniform pulsation, the volume velocity is:
(9)
(9) The above method shows that the volume of the cavitation-generated bubbles is equivalent to an equal-volume pulsating sphere source. After determining the sound source of the pulsating sphere source, the sound pressure radiated due to the pulsating volume of the bubbles at any acoustic monitoring point can be calculated based on Equation (Equation8
(8)
(8) ).
2.2.3. Acoustic evaluation
In practical engineering applications, the noise magnitude is not convenient to measure using the absolute value of the sound pressure, so logarithmic coordinates are often used to measure its intensity. Sound pressure level (SPL) and total SPL can be determined using a calculation formula from the studies of (Si, Shen, et al., Citation2020) and (Si, Shen, et al., Citation2019).
3. Numerical simulation method and test validation setup
3.1. Numerical methodology for the prediction of cavitation
3.1.1. Computational domain
To study the cavitation hydrodynamics of the centrifugal pump and its induced noise characteristics, Creo5.0 (3D modelling software) was used in this study to build the calculation domain of the water bodies of the model pump. The calculation domain comprises six parts, i.e. inlet and outlet extension sections, impeller wear-ring clearance of impeller, shroud impeller, pump cavity, and volute. The length of the inlet and outlet section is extended by five times the pipe diameter to maintain the stability of fluid flow within the model pump inlet and outlet pipelines, thereby improving the accuracy of numerical simulation. The calculation domain of the model pump water body is shown in Figure .
3.1.2. Meshing and grid-independence analysis
To enhance the simulation accuracy and speed up the convergence mechanism, the structured grids of the calculation domain were built to capture more details of the flow inside the model pump. The grid division results are shown in Figure . To avoid the impact of mesh numbers on the numerical simulation results, a total of five sets of a different number of meshes were divided for the numerical simulation at the design conditions of the model pump for grid independence verification (See Figure ). According to Figure , when the grid numbers are larger than 6,342,000, the head and efficiency are almost stabilised with the increase in the grid numbers. The relative errors of both head and efficiency are less than 1%. The corresponding studies (Y.-b. Li, Fan, et al., Citation2020; Si, Shen, et al., Citation2019) indicate that the impact of the mesh components can be neglected if the absolute inaccuracy of the calculated value of the two heads before and after is less than 2%. Therefore, the third set of grids (6,342,000) was finally selected for numerical simulation while ensuring the calculation accuracy and computational cycle.
The different turbulence models have additional requirements on the y + value of the grid near the wall (Zhang et al., Citation2021), and the average y + value of the water body grid of the model pump in this study is about 5. It is known from the literature (Zhang et al., Citation2021) that for the centrifugal pump with complex geometry and internal flow, the obtained y + value agrees well with the numerical simulation of cavitation requirements.
3.1.3. Numerical simulation approach (DES and improved RZGB models)
To capture the vortex characteristics more effectively, the steady calculation based on the Reynolds time average is usually carried out before the detailed unsteady analysis. The most widely used turbulence models mainly include the k-ε model, RNG k-ε model, Wilcox k-ω model, standard k-ω model, and SST k-ω model (Gao et al., Citation2017). The SST k-ω model is used for the steady cavitation flow for the respective advantages in the near-wall region and free shear layer. Further, the numerical simulation of the unsteady cavitation flow adopts the DES method based on the SST k-ω model, and its mathematical derivations are provided in the literature (H. Cheng, Long, et al., Citation2021). When the cavitation occurs in the centrifugal pump, the air bubbles flow along the edge of the blade, where both turbulence and shear force break the bubbles of a specific diameter into many tiny bubbles, which indicates the existence of the largest diameter of the bubble in the centrifugal pump. However, the traditional cavitation models (such as ZGB and Kunz) do not consider this characteristic of the cavitation bubble in the impeller flow channel. Therefore, to more accurately simulate the unsteady cavitation flow characteristics of the model pump, it is necessary to further improve the ZGB cavitation model with the help of secondary development technology. The specific process and mathematical derivations for an improved RZGB cavitation model are given in Appendix A (2) (Jian et al., Citation2018).
3.1.4. Boundary conditions
To analyse the internal-flow characteristics of the model pump under different operating conditions, CFD simulations for predicting cavitation were conducted using ANSYS CFX, where the working medium is clean water (ρl = 998 kg/m3) at room temperature. The boundary conditions for the inlet were set to total pressure, while the reference pressure was set to 0 Pa. The outlet was set to mass flow, and the wall boundary condition was assessed as no-slip wall. The wall function was arranged as an automatic wall function, and a frozen rotor was placed as the interface of dynamic-static intersection. The convection term was in high-resolution differential format, while the RMS convergence residual was arranged as 10−5.
Firstly, the numerical simulation of pure water was carried out for eleven flow conditions, namely 0.2∼1.2Qd of the model pump. After the numerical simulations were converged, the pure water numerical simulation results were used as the initial conditions to carry out the numerical simulation of steady cavitation flow with different cavitation numbers. The saturated vapour pressure was 3169 Pa at 25°C, which means cavitation would appear when pressure was lower than this value inside the pump channel. For the CFD simulations of unsteady cavitation flow, the dynamic-static intersection was set to transient rotor stator, and the impeller rotated 3° as a time step, i.e. Δt = 0.00017182 s. A suitable time step is essential for calculation validation for unsteady simulations. The Courant number is usually used to determine if the time step meets the periodic numerical simulation and is stated by Equation (Equation10(10)
(10) ) (Si, Bois, et al., Citation2020). After the required convergence was complete, the data from ten full rotations were saved, and this information was then utilised to create the sound source file.
(10)
(10) where L denotes the grid cell’s smallest size, v denotes the main flow’s highest velocity, and Δt is the time step. According to the grid information in this paper, when the model pump operates at 2910 r/min, the smallest grid size is about 0.043 mm, whereas the largest value of v is around 18.59 m/s. Then, the obtained value of the highest C0 from the calculations is about 74.2, which validates that the selected time step is credible. The steady cavitation numerical simulation results were used as the initial conditions for the CFD simulations of the unsteady cavitation flow of the model pump.
3.2. Acoustic simulation methodology for the prediction of cavitation-induced noise
3.2.1. Acoustic computational domain and meshing
The computational domain for acoustic-field simulations is similar to flow-filed simulations (discussed in Section 3.1). However, the meshing principles for both simulations differ significantly from each other. The major difference is associated with the grid size, which is larger in the acoustic field than the flow field, and there is no impeller in the acoustic calculation. The sound source information received from CFD calculations is converted into computational acoustics using grids. The interface between the rotating impeller and the volute receives source data from the inlet pipe and the rotating impeller as a surface acoustic source. However, the volute domain itself loads the sound source data from the volute region as a volume acoustic source. The acoustic grid is often coarser than the CFD grid. To guarantee that all information is preserved throughout the information transfer process, the integration solution of the CFD findings was used on the acoustic mesh in this study, as illustrated in Figure . The preprocessor iCFD from the programme Actran was used for this study. When CFD nodes are located in the acoustic mesh structure, it gives the mean flow interpolation technique. Additionally, smoothing procedures are possible since the acoustic mesh is coarser than the CFD mesh at the boundary. This regularises the flow field and automatically eliminates the boundary layer calculated in the RANS simulation to guarantee the convergence of the acoustic equations (Y. Wang et al., Citation2022).
3.2.2. Acoustic simulation accuracy analysis
To ensure the accuracy of the acoustic finite element calculation, the maximum size of the acoustic grid should be less than 1/6 of the wavelength corresponding to the maximum calculated frequency, i.e.
where
denotes the maximum size of the acoustic grid; c denotes the speed of sound at 33°C, and its value in water is 1517 m/s.
represents the maximum frequency of acoustic calculation, and the maximum calculation frequency of the acoustic finite element method is 2910 Hz, which finally provides the value of
<0.087 m. The maximum mesh size is 0.006 m, which can fully satisfy the mesh’s acoustic finite element calculation requirements.
3.2.3. Solution methods of cavitation-induced noise in the model pump
3.2.3.1. Cavitation-induced noise with no cavitation volume pulsation part
When solving the model pump cavitation flow-induced noise, it is first necessary to achieve convergence in the CFD simulation of the unsteady cavitation flow of the model pump. We selected the flow-field information gained through simulation outcomes in the last ten cycles in CFX-Solver Manager. The flow-field information includes the pressure, velocity, and density terms. It is outputted in.ensightgold format as sound source information loaded into the water body of the inlet extension of the centrifugal pump calculation domain. A 0.003 m thick shell was added to the outer wall surface of the inlet extension calculation domain to prepare the test conditions. The pipe modes were included in the calculation domain’s inlet and outlet. The purpose is to make the sound non-reflective from the inside to the outside propagation.
The total time for 10 cycles of unsteady cavitation flow calculation is 0.2062 s, with the time step value of Δt = 0.0001718 s. Following the Nyquist-sampling hypothesis, the frequency range of acoustic analysis can be taken as 0∼2910 Hz, and the frequency resolution is 4.85 Hz. To study the frequency-domain response characteristics of noise, a direct frequency-domain response analysis is required, so the sound field monitoring points that remain uniform with the installation position of the inlet hydrophone of the pump model were set up, as shown in Figure .
The flowchart of the whole acoustic simulation process is presented in Figure , which signifies the entire calculation mechanism of acoustic simulation. According to the figure, the unsteady numerical simulations are performed by selecting the DES turbulence model and cavitation model, which consider all the velocity, pressure, density, and other flow-field information, including all kinds of bubble information. The achieved data from numerical simulations is then processed in Actran, which extracts all type of noise sources based on CFD results, including the nonlinear bubble volume fluctuation noise source. Finally, all these results are post-processed to obtain the required acoustic calculation results.
3.2.3.2. A solution method for cavitation volume pulsating radiated noise
The calculation model of the cavitation volume pulsating radiation noise is shown in Figure . The impeller cavitation area in the figure is regarded as a volume source of equal volume, so the actual surface of the impeller cavitation area of the air bubbles can be transformed into the virtual surface of the spherical bubble. The volume of cavitation bubbles at different times can be substituted into Equation (Equation8(8)
(8) ) to acquire sound pressure at any sound field monitoring location. The frequency-domain data of the pulsating radiation noise can be obtained by performing an FFT transformation of the collected time-domain sound pressure data. The calculation formula for evaluating the model pump’s internal cavitation volume is as follows:
(12)
(12)
3.3. Experimental validation setup
To study the cavitation noise characteristics of a centrifugal pump, a multi-field synchronised experimental system was designed and built in the Jiangsu University pump laboratory (national research centre of pump). It consists of three main systems: (1) The liquid delivery system, which includes a closed storage tank, ball valve, fluid delivery lines, electric control valve, electromagnetic flow meter, and the model pump. (2) The acquisition system, which contains a hydrophone, vacuum pump, hydrostatic and dynamic pressure sensor, torque meter, NI USB-6343 acquisition card, LMS Test Lab signal acquisition and analysis system, LabVIEW acquisition system, and computer. (3) A vacuum regulation system that includes a vacuum pump.
3.3.1. Design of hydraulic cavitation system
Cavitation system has been designed by ensuring that the cavitation bubbles and degassing produced by the cavitation inside the pump shall not affect the operational characteristic of instrumentation particularly the flow measuring device (Patil & Baral, Citation2021). Piping setup and instrument accuracy meet the requirements of grade 2B in the international standards ISO 9906:2012 ‘Rotodynamic Pumps-Hydraulic performance acceptance tests-Grades 1, 2 and 3’. All the test results were taken at a constant temperature of 25°C. When testing the performance of the centrifugal pump without cavitation, it is necessary to open the valve on the top of the closed water storage tank, and the whole testing system is an open system connected with the atmosphere. Frequency converter is used to ensure that the pump operates at a stable speed and the electric control valves is used to change the flowrate of the pump. The instruments such as electromagnetic flowmeter, pressure sensor, torque power meter combined with the NI card and the acquisition programme are used to obtain the pump performance curves after data processing. It is necessary to reduce the inlet pressure for cavitation test of centrifugal pump. At this time, it is necessary to close the valve on the top of the closed tank, thus the test system is a closed system unrelated to the atmosphere. The pressure of the whole system is reduced by running the vacuum pump connected with the closed tank, thus causing the hydraulic cavitation developed inside the centrifugal pump under different flowrate. The vacuum pump, electromagnetic flowmeter and regulating valve are linked to ensure that a group of cavitation experiments are tested under constant flowrate. At the same time, to obtain pump performance under different cavitation conditions, LMS test system and hydrophones are used to collect the hydrodynamic noise. Figure shows the layout of the experimental bench, which highlights the design of cavitation system as well.
3.3.2. Model pump design parameters
The model pump is an NKG65-50-125 single-stage single-suction centrifugal pump, the main hydraulic design parameters of which are presented in Table .
Table 1. Model pump hydraulic parameters.
3.3.3. Main equipment and parameters
The main equipment used to collect the experimental performance parameters and dynamic signals of the model pump operation is shown in Table , along with its relevant parameters. This equipment contains different parameters needed to calculate the pump head and efficiency. The formulas used for head and efficiency calculation methods are well known and can be found in several studies conducted on turbomachinery (Ali, Si, Yuan, et al., Citation2022; Qiaorui et al., Citation2022; Si, Shen, et al., Citation2020).
Table 2. Main equipment functions and parameters.
3.3.4. Uncertainty analysis of the test measurements
During the experiments, systematic and random errors are the primary sources of uncertainties in measuring various physical quantities, and these errors cannot be avoided. To enhance the test accuracy, it is necessary to perform uncertainty analysis on the test data. Thus, a total of 10 sets of data for flow rate, head, torque, and rotational speed were collected, and the random (Er), systematic (Es), and integrated uncertainty (E) of the above physical quantities were obtained.
All the uncertainty results for the present study were obtained according to the uncertainty calculation formula used by (H. Sun et al., Citation2018) and based on the standard ISO 9906:2012. The results for uncertainty measurement were calculated as ±0.20% for flow rate, ±0.25% for the head, ±0.014% for torque, ±0.0365% for rotational speed, and ±0.34% for efficiency. The maximum value of uncertainty measurement of the physical quantities does not exceed 0.34%, which indicates that the experimental results of this paper have a certain reliability. These assessments are based on the irregularities observed during the experimental measurements.
3.3.5. Design of inlet cavitation-induced noise measuring device
The hydrophone can be installed on the inlet pipe of the model pump directly to capture the cavitation-induced noise signal effectively. However, the pump operates in a cavitation state when the inlet is a low-pressure area. Therefore, the cavitation noise measurement device needs excellent sealing at the inlet. If the hydrophone is inserted directly into the inlet pipe through a device, it is difficult to ensure that the device has excellent sealing. Moreover, if the sealing is not covering the gap properly, the outside air entering the pump system pipeline through the gap of the measurement device affects the test measurements. Thus, this study involved the design of a model pump inlet cavitation-induced noise measurement device that can solve the sealing problem and evaluate the effect of the variation of components (sound transmission performance) on the noise measurement (C. Baker & Sorbie, Citation2001; Ciochon et al., Citation2023), as shown in Figure .
Figure 10. Two-dimensional and physical diagram of the model pump inlet cavitation-induced noise. measurement device; (a) 2D schematic diagram, (b) physical diagram.

The figure shows that the device connects the polyurethane acoustic permeable window to the location where the acoustic window is placed to ensure that the device has excellent sealing. The acoustic window and the pipe (where the window is placed) have exact dimensions. Therefore, there is no variation in the components, which could influence the noise measurement. The hydrophone is located in the closed water tank, and the fixing device controls its height on top of the sealing cover. The cavitation-induced noise signal enters the closed water tank through the permeable window from the inlet pipeline and then captures the cavitation-induced noise signal through the hydrophone.
As the material of the sound-transmitting window is polyurethane, it has good sound-transmitting and mechanical properties, which can ensure that the noise signal passes through the sound-transmitting window almost without reflection or loss (Tiuc et al., Citation2019). The available studies in the literature (Das & Mahanwar, Citation2020; Gupta & Parey, Citation2021; Kurańska et al., Citation2020) and data provided by the suppliers show that polyurethane rubber has an excellent sound-transmission performance in the wide frequency range, and the noise attenuation is less than 0.1 dB per centimeter of thickness. Furthermore, polyurethane materials can eliminate the reflection of sound waves in water and maintain sound wave transmission without distortion, such as tetrahydrofuran co-polyether polyurethane (Shimizu et al., Citation2004) with a sound transmission rate of more than 99%, which is used in the transducer seals of marine sounding. In summary, this device can make the sound waves of cavitation-induced noise enter the closed water tank through the sound-transmitting window without reflection or loss and then capture the cavitation-induced noise signal through the hydrophone. The inlet cavitation noise measuring device has an uncertainty value of about 0.9%, which was included during the data reduction process of the obtained results.
4. Results and discussion
4.1. Numerical simulation results of cavitation in model pump
4.1.1. Validation of model pump external characteristics
To analyse the external characteristics of the model pump under non-cavitation conditions, the data on the external features of the model pump under various flow conditions were obtained by testing and numerical simulation. Figure shows the comparison of the test and the numerical simulation of external characteristics of the model pump under designed working conditions. To facilitate the performance prediction process, the flow rate and head are shown in the dimensionless parameters (head coefficient ψ and flow coefficient φ), and their calculation method can be found in the studies of (Qiaorui et al., Citation2022) and (Ali et al., Citation2021; Ali, Si, Wang, et al., Citation2022).
Figure 11. Comparison of experimental and simulation results of external characteristics of the model pump.

From the figure, it can be observed that the trends of the numerical simulation results agree well with the test measurement results in the whole flow range. Concerning the head curve, the numerical simulation head is slightly higher than the experimental head in the entire flow range, but the deviation of the head at small flow rate conditions is smaller. On the other hand, for the efficiency curve, the variation of the simulated efficiency from the experimental one is smaller in the small flow rate conditions. However, the numerical simulation efficiency deviation from the experimental one is more significant under large flow rate conditions. The reason for some errors between simulation and test may be the inconsistent leakage at the impeller eye. Under the designed flow conditions, the model pump experimental head is 22.02 m (ψ = 0.95), while the simulated head is 22.90 m (ψ = 0.99), indicating that the relative error between the two is around 3.99%. On the contrary, the simulated efficiency of the model pump is 75.47%, whereas the experimental efficiency is 72.65%, indicating that the related error among these two values is around 3.88%. The relative error for both head and efficiency are less than 5% under both design and other flow rate conditions, indicating that the selection of turbulence model and boundary conditions setting of the numerical simulation are credible.
4.1.2. Validation and selection of model pump cavitation model
The cavitation characteristics of the rated conditions (Q = 43 m3/h, φ = 0.95) of the model pump were predicted using the improved RZGB model and conventional cavitation models. The results were then compared with the test results to obtain the cavitation characteristics comparison graph with different cavitation numbers, as shown in Figure . The cavitation number is a non-dimensional parameter determined based on the calculation formula provided in previous studies (Kozmus et al., Citation2022; Soyama, Citation2021). Under design flow rate conditions, the initial test and simulated head coefficients are 0.95 and 0.99, respectively.
Figure 12. Comparison of calculation results of different cavitation models with experimental results.

From the figure, it can be seen that the prediction of the external characteristics of the model pump by using different cavitation models has certain differences, with the ZGB cavitation model having the largest error and the Singhal cavitation model the second. However, the prediction results of the RZGB cavitation model are closer to the experimental values than the ZGB and Singhal cavitation models. When σ = 0.053, a 3% drop in head occurs, indicating that the selection and improvement of the cavitation model are crucial for the prediction of the cavitation characteristics of the model pump. Moreover, as the cavitation number decreases (σ = 0.09), the model pump head coefficient decreases significantly in the test and simulation. With a further reduction in cavitation number (below 0.053), the external characteristic curve predicted by the RZGB cavitation model fits the test curve more closely than ZGB and Singhal cavitation models. This is because both the ZGB and Singhal cavitation models are derived from the simplified R-P equation, which can predict the unsteady cavitation flow more accurately, but neither of them considers the effect of impeller rotation effect on cavitation, while the evaporation and condensation source term of the RZGB cavitation model considers the effect of impeller rotation on the cavitation, making the prediction result of the RZGB cavitation model closer to the experimental result. This is why the RZGB cavitation model was used in this study for the numerical simulation of the model pump with complex unsteady cavitation flows.
4.1.3. Cavitation evolution process
Figure shows the cloud plot of the volume fraction distribution of cavitation in the impeller runner of the model pump at rated speed and with different cavitation numbers and pressure values. Cavitation happens in four different stages: incipient cavitation, cavitation development, critical cavitation, and fracture cavitation. Therefore, all the cavitation numbers were selected by considering all these different cavitation stages for the deep acoustic analysis. Moreover, the iso-surface of 10% in the figure indicates the location of the region where the cavitation is located, and the pressure value renders the iso-surface of the cavitation. The region rendered by blue is the low-pressure region, which indicates that cavitation is generated in this region. In contrast, the region rendered by red is the high-pressure region, which suggests that the cavitation is collapsed in this region. Furthermore, this volume fraction is calculated for a mean value over all blades, which can vary due to cavitation instability and/or during rotation due to effects of unsteadiness related to the shaft frequency.
Figure 13. Evolution of internal cavitation in model pump with different cavitation numbers and pressures.

As shown in the figure, when σ = 0.43, the impeller inlet pressure is high, and cavitation does not occur at the blade leading edge. However, as the pressure of the impeller inlet decreases (when σ = 0.16), a small part of the cavitation area appears at the suction surface of the blade leading edge. This indicates that the local pressure of the water inlet of the model pump at this stage is less than the saturation vapour pressure of the water at this temperature, resulting in the cavitation-incipient phenomenon. With the further reduction in the inlet pressure (when σ = 0.07), the cavitation appears along the blade suction surface to the impeller runner outlet. At this stage, the cavitation covers nearly half the blade suction area. When σ = 0.062, the cavitation steadily begins to occur at the blade pressure surface. This is because the cavitation is more severe at this time, where cavitation bubbles begin to start blocking the impeller flow channel. Furthermore, when σ = 0.06 and σ = 0.051, the low-pressure region is quite apparent, indicating that the cavitation phenomenon is more serious. Moreover, the low-pressure area in the impeller flow channel is almost entirely blocked by the cavitation bubbles, reducing the hydraulic performance of the model pump.
4.1.4. Effect of cavitation on vortex structure
The cavitation inside the pump is usually accompanied by the generation of vortices. These vortices consist of a vortex field, which describes the spatial distribution of vortices, and refer to the concentrated vortex. These transient vortex structures were tracked in ANSYS CFX using CFX expression language (CEL). Using CEL allows us to declare inputs as variables, record outcomes as variables, and control those variables. Moreover, the vortex core inside the model pump can be determined by the regularised spiral Hn, and its expression is given as follows:
(13)
(13) where
is the velocity vector, and
is the vortex vector. The positive and negative signs of the regularised spiral Hn indicate the direction of rotation of the vortex. However, the flow direction is defined as positive, i.e. Hn is positive, meaning that the direction of rotation of the vortex is counterclockwise, and Hn is negative, indicating that the direction of rotation of the vortex is clockwise.
The Q-criterion is a widely used method for identifying and visualising the vortex structures in complex flow fields inside hydrofoils and pumps. Reference (Hunt et al., Citation1988) proposed the Q-criterion in 1988, identifying the vortex structure based on the positive region of the second matrix invariant Q of the velocity gradient tensor in the flow field. This criterion reflected the balance between fluid deformation and fluid rotation in the flow field. For instance, Q > 0 indicates that the fluid in the flow field is mainly rotating, and Q < 0 means that the fluid in the flow field is significantly deformed. The expression of the Q-criterion is as follows:
(14)
(14) where
denotes the symmetric part of the velocity gradient tensor,
represents the asymmetric part of the velocity gradient tensor, and
indicates the Frobenius parameter.
Figure shows the cloud plot of the regularised spiral (Hn) distribution on the middle section of the impeller at rated speed and different cavitation numbers. From the figure, it can be seen that there is a vortex with a counterclockwise rotational direction in the impeller runner outlet area near the blade suction surface. There is a vortex with a clockwise rotational movement in the impeller runner inlet area near the blade suction surface. This indicates that a pair of vortices with opposite rotational directions exist on the blade suction surface. With the periodic rotation of the impeller, this pair of vortices is continuously generated and collapsed.
With the occurrence and development of cavitation, these pairs of vortices with the opposite rotational direction are gradually merged. In the impeller runner inlet near the blade pressure surface, there is a vortex with a counterclockwise direction of rotation. Moreover, with the continuous development of cavitation, the vortex and the counterclockwise vortex near the blade suction surface in the outlet area of the impeller flow channel gradually merge into a counterclockwise vortex in the same direction of rotation. At this stage, the vortex at the inlet of the impeller runner and the direction of rotation also gradually disappear. From the above analysis, it can be seen that the evolution process of cavitation in the impeller runner has an important influence on the position of the vortex core.
Figure illustrates the cloud map of Q-value distribution on the middle section of the impeller at rated speed and different cavitation numbers. It can be seen from the figure that the range of Q-value located in the middle region of the impeller suction surface regularly increases with the occurrence and development of cavitation in the impeller runner, while the Q-value situated in the outlet area of the impeller flow channel gradually decreases and disappears with the effect of cavitation in the impeller runner, indicating that the change of Q-value affects the distribution of the vortex core in the impeller channel. At different stages of cavitation, the generation of the vortex in the pump is mainly associated with the impeller’s rotational motion, and there is a considerable energy loss in the vortex region.
Combined with Section 4.1.3 of the blade surface cavitation distribution cloud map, it can be found that the Q-value distribution and blade surface cavitation volume fraction distribution are more consistent. With a certain correspondence, the Q-value at the inlet area of the impeller flow channel is significant, indicating that the rotation and strain of the fluid micro-clusters in this area are not in equilibrium, resulting in flow instability in the cavitation area. This also shows that the vortex has a promoting effect on the formation of cavitation. Moreover, the range of Q-value in the non-cavitation area is small or negative, and there is a strong vortex movement in the cavitation area at the suction surface and pressure surface of the blade, indicating that the cavitation also has a promoting effect on the formation of the vortex.
4.2. Cavitation-induced noise results and analysis
4.2.1. Acoustic power level distribution of cavitation flow field
Sound power is the total energy of sound radiated by a sound source into space per unit of time, in units of W. In 1952, (Proudman & Taylor, Citation1952) proposed a formula for calculating sound power based on theoretical analysis. However, there was a problem with the delay time difference in the derivation process. Therefore, (Lilley, Citation1994) solved the issue of the delay time difference in 1993 and derived a new equation for sound power with the following expressions:
(15)
(15) where α is a constant, generally takes the value of 0.1, while c denotes the speed of sound in water, valued about 1500 m/s; l denotes the turbulence scale; and u indicates the turbulence velocity, and the expression is as follows:
(16)
(16) where k denotes the turbulence energy of the fluid. Thereby, Equation (15) can be expressed as:
(17)
(17) where
, and the formula for calculating the sound power level is:
(18)
(18) where Wref represents the reference sound power, taking the value of 10−12 W/m3.
Cavitation is mainly generated inside the impeller flow channel. Therefore, to study the impact of cavitation on the variation of the model pump’s internal acoustic power, the acoustic power of the middle section of the model pump is selected for comparative analysis. Figure represents the cloud map of the sound power distribution in the model pump’s middle region at rated operating conditions and different cavitation numbers. The figure shows that under non-cavitation conditions, the blade outlet near the volute tongue has a large sound power. This indicates that the static and dynamic interferences between the blade and volute tongue at this stage are the primary source of flow-induced noise inside the model pump. With the development of cavitation, the acoustic power value and the area with a more significant acoustic power value are gradually increased due to the cavitation volume pulsation of the radiating monopole noise.
Figure 16. The sound power distribution of the cavitation flow field at the model pump middle section.

The area with increased acoustic power value is mainly observed in the downstream region of the impeller flow channel and the intersection region among the rotor and volute inlet. However, in this process, the acoustic power value of the flow channel near the impeller inlet area is always kept at a minimal value. As the cavitation number reduces by about σ = 0.06, the sound power value increases significantly by 10–20 dB compared with the previous cavitation stages. As the cavitation number further reduces by about σ = 0.051, the sound power value inside the impeller flow channel is the largest due to the air bubble’s collapse in the downstream high-pressure area.
Figure illustrates the distribution of sound power of the cavitation flow field on the blade surface of the model pump under rated operating conditions and different cavitation numbers. According to the figure, in various stages of cavitation, the inlet side suction surface has a large acoustic power value, which may be caused by the influence between the flow and the inlet edge. With the same cavitation number, the sound power value of the blade suction surface is generally larger than the blade pressure surface. It can be observed that the sound power value of the blade pressure surface near the impeller rear cover plate region is significantly smaller than near the front cover plate region. In addition, whether it is a blade suction surface or pressure surface, its sound power value near the leading edge of the blade to the trailing edge of the blade shows a trend of first decreasing and then increasing. With the further development of cavitation, the blade suction surface is gradually covered by a large number of air bubbles, and its acoustic power value is also increased. At this stage, the monopole noise of the cavitation volume pulsation is the primary noise source for the increase in acoustic power value. On the suction surface of the blade, when σ = 0.16, the cavitation is generated at the edge of the blade inlet.
Figure 17. Cavitation flow-field and acoustic power distribution at the blade surface of the model pump.
Note: The blue iso-surface (10%) in the figure indicates the location of the region where the cavitation bubble is located, and the pressure change renders it.

With the further development of cavitation, the area of the cavitation covering the suction surface gradually increases, and the cavitation collapses after the bubbles move with the mainstream to the high-pressure area downstream of the impeller. At this stage, the sound power value of the rotor-stator interaction area is the largest, as shown in Figure (f). Compared with the previous cavitation stage, the sound power value increases by about 30 dB, while in the blade pressure surface, when σ = 0.062, the blade pressure surface has not yet produced any cavitation bubbles. However, when σ = 0.06, the cavitation bubbles cover about half of the area of the blade pressure surface, and the sound power value of the area covered by cavitation bubbles also increases. Moreover, when σ = 0.051, the cavitation bubbles almost blocked the whole impeller flow channel due to the collapse of the air bubble. The same happened in the impeller outlet and volute casing intersection region, where the value of sound power is the largest. Furthermore, when the sound power distribution on the blade’s pressure surface and the air bubble’s volume distribution are compared, it was discovered that the fluctuation in sound power on the blade suction surface is more noticeable than on the blade pressure surface.
4.2.2. Acoustic properties of cavitation noise with no cavitation volume pulsations
For centrifugal pumps, the reduction in inlet pressure first produces cavitation at the blade’s leading edge, which affects the pressure, speed, and density of the flow field in the impeller flow channel to a certain extent. Then, the cavitation collapses and generates noise after moving to the high-pressure area of the flow channel with the mainstream. The time of cavitation inside the pump is very short. Therefore, capturing the noise radiated by the nonlinear collapse process is challenging through numerical simulation and experimental means. However, the noise radiated by the flow field’s pressure, velocity, and density changes caused by cavitation can be calculated and analysed through numerical simulation. Figure shows the spectral curve of cavitation-induced noise at the model pump monitoring point with no cavitation volume pulsation. The last two stages of cavitation were selected to be shown below because cavitation is more severe during these stages. The Strouhal number (St) used in this figure is known as the dimensionless frequency of cavitation shedding corresponding to different cavitation numbers calculated using the method proposed by (Yang et al., Citation2021) and is calculated as follows:
(19)
(19) where D2 indicates the impeller outlet diameter, mm; z shows the number of blades; f indicates frequency, Hz; and fBPF indicates the blade passage frequency, Hz.
According to the figure, the more significant value of the cavitation-induced noise SPL at different cavitation numbers is gathered in the lower frequency band, i.e. 0∼2StBPF. This means that the acoustic energy of the cavitation-induced noise of the model pump with no cavitation volume pulsation is mainly concentrated in this frequency band, and the SPL is smaller in the middle- and high-frequency bands. The size of the SPL shows a pattern of first increasing and then decreasing simultaneously with the increase in frequency. When σ = 0.051, the cavitation inside the model pump is severe at this time, and the spectral curve of cavitation-induced noise shows a prominent discrete characteristic. In addition, the SPL’s primary frequency is controlled by the blade passing frequency and its multiples, indicating that the cavitation of the model pump causes a change in the pressure, velocity, and density of the flow field. The blade sweep still modulates the cavitation-induced noise with no cavitation volume pulsation.
4.2.3. Cavitation noise SPL and sound source distribution with no cavitation volume pulsations
4.2.3.1. Sound source distribution in the inlet area of the model pump
Figure illustrates the cloud chart of the sound source distribution in the model pump inlet area at the shaft frequency and the blade frequency when σ = 0.06. According to the figure, for the model pump inlet extension at shaft frequency, the intensity of the sound source at the inlet extension is mainly delivered at the intersection of the impeller inlet and the inlet section. However, the sound source distribution is not uniform, and the sound source intensity along the upstream inlet section shows a trend of first decreasing and remaining unchanged, while at the blade frequency, the sound source intensity at inlet extension is collected in the area in front of the impeller inlet, and the distribution is uniform. In this case, the sound source intensity along the upstream inlet section shows the same pattern as the axial frequency.
4.2.3.2. Distribution of SPL at inlet area in model pump
Figure illustrates a cloud map of the SPL distribution in the last two stages of cavitation in the model pump’s inlet area under rated working conditions and different cavitation numbers. From the figure, one can observe that the maximum SPL of each cavitation stage is located in the area before the impeller inlet, indicating that this area is the primary source of the cavitation-induced noise that does not consider the cavitation volume pulsation.
As the cavitation-induced noise propagates upstream towards the centrifugal pump’s inlet section, the size of SPL is almost attenuated. However, the upstream SPL is smaller than the SPL in the area before the impeller inlet. In addition, in the area before the impeller inlet, there are six alternating maximum and minimum SPL regions corresponding to the number of blades. This indicates that the impeller’s rotational effect significantly influences the change of cavitation-induced noise SPL in the impeller inlet front region of the model pump, with no cavitation volume pulsation. In the meantime, the magnitude of SPL in the inlet region tends to decrease with the decrease in the cavitation number.
4.2.4. Acoustic characteristics of cavitation volume pulsating radiated noise:
After the transient calculation is stabilised, the cavitation volume was calculated. Figure illustrates the variation of cavitation volume in the flow channel with time iteration with different cavitation numbers. The abscissa indicates the last 10 cycles of impeller rotation.
Figure shows that when the cavitation number reduces, the cavitation becomes more significant, and the volume of cavitation bubbles increases. At this stage, many cavitation bubbles are already gathered in the rotor flow passage. At the initial phase of cavitation, it can be observed that the volume of cavitation bubbles has multiple ‘peaks and valleys’ with time. As the number of cavitation decreases, the number of peaks and valleys gradually decreases in both the cavitation development and the severe cavitation stage, indicating that with the rotation of the impeller, the cavitation volume is affected by the law of periodic generation, development, and collapse inside the model pump. However, the time taken by this dynamic process varies with different cavitation numbers. The reason for this phenomenon is that when the centrifugal pump inlet pressure is high, even if the blade inlet suction surface produces a small amount of cavitation, because of the high fluid pressure in the impeller flow channel, the cavitation bubbles are yet to collapse with the mainstream movement to the downstream of the impeller flow channel. Therefore, an impeller rotation cycle has more than one cavitation evolution cycle.
When the inlet pressure in the model pump is low, the blade inlet suction surface produces a large number of cavitation bubbles, and the fluid pressure in the impeller runner is also low at this time. The cavitation only collapses when the cavitation moves downstream of the impeller flow channel with the mainstream movement, so the multiple cycles of impeller rotation in the severe stage of cavitation form a cavitation evolution cycle. Furthermore, according to cavitation volume inside the model pump at different cavitation stages as a function of time, the frequency of cavitation shedding (Strouhal number, St) corresponding to different cavitation numbers is calculated, as shown in Table . The Strouhal number (St) is calculated using the method proposed by (Yang et al., Citation2021).
Table 3. Frequency of cavitation bubble shedding at different cavitation stages.
Figure illustrates the frequency-domain analysis of the pulsating radiated noise of the cavitation volume of the model pump with different cavitation numbers. The figure explains that when the frequency changes, the SPL of the cavitation volume pulsating radiated noise shows a trend of first increasing, then decreasing, and then basically remaining unchanged. This is characterised by discrete spectra at low frequencies and continuous spectral features at high frequencies. The acoustic energy of cavitation volume pulsating radiation noise is mainly concentrated in the middle- and low-frequency bands, caused by the growth and shedding of cavitations in the impeller flow channel. The characteristic frequencies of different cavitation stages are not the same, and the frequency corresponding to the maximum peak of the SPL is consistent with the cavitation shedding frequency. Thus, the cavitation shedding modulates the pulsating radiation noise of the model pump cavitation volume.
4.3. Test results and analysis of cavitation-induced noise
4.3.1. Acoustic characteristics of inlet cavitation-induced noise
Figure (a) depicts the time-domain sound pressure curve of the model pump inlet at a speed of 2910 r/min and a flow rate of 43 m3/h. The figure illustrates that the sound pressure of the prototype pump inlet has a more noticeable periodic pattern during the development and collapse of cavitation with no cavitation state and before initial cavitation. This noise is generally generated by each single bubble implosion. It has a particular frequency (tonal noise), and this spectrum is related to some noise coming from far-field pressure variations, not because of cavitation. Moreover, the periodic pattern of the inlet sound pressure in both the development and severe stage of cavitation is weakened compared to the no-cavitation state and before the initial cavitation.
Figure 23. (a, b) Time-domain sound-pressure curve at the model pump inlet; (c) frequency-domain diagram of the model pump inlet hydrophone SPL.

With the reduction in cavitation number, the sound pressure of the model pump inlet displays a progressive decline pattern during the whole stage of cavitation development. The sound pressure of the model pump inlet shows a gradual decrease trend. This phenomenon can be well explained by noting that the primary cause of flow-induced noise in pumps is the dynamic and static interference of the blade and volute tongue in the non-cavitation stage. However, with the reduction in inlet pressure, the low-pressure area of the model pump impeller is cavitation. At this time, the type of cavitation in the impeller flow channel is an independent, free type of cavitation, which collapses after the mainstream movement to the high-pressure area, resulting in strong radiation noise. With the further reduction in cavitation number, the degree of cavitation is more serious, and a large number of individual and free cavitation bubbles form larger cloud-like cavitation bubbles. At this stage, the impact force and radiation noise generated by the collapsed bubbles in the higher-pressure zone are absorbed by the impeller runner’s cloud-like bubbles in the lower-pressure area. In contrast, the collapse rate of the cavitation bubbles is reduced accordingly after the inlet pressure is reduced.
To create the frequency-domain diagram (See Figure (c)), the collected time-domain noise signal was obtained by FFT transformation of the model pump inlet SPL with a speed of 2910 r/min and a flow rate of 43 m3/h. The horizontal coordinate in the figure is in the logarithmic form to reflect the characteristic frequencies of the model pump noise during the development of cavitation.
The figure explains that the main frequency of sound SPL is dominated by the shaft and blade passing frequency along with their multiples. The amplitude of SPL on blade passing frequency under σ = 0.33 working conditions is more significant than that at other working conditions, while the SPL amplitude at the shaft frequency does not change much. With the development of cavitation, the band in the frequency range of 0.344–3.436StBPF tends to decrease and become narrower. The frequency band declines in the frequency range of 3.436–34.364StBPF, indicating that the cavitation-induced noise energy at the inlet monitoring point of the model pump decreases at high frequencies with the development of cavitation. This is because, on the one hand, with the continuous reduction in the inlet pressure of the model pump, the degree of cavitation is intensified, and the number of cavitation bubbles in the rotor-flow passage increases, forming an apparent liquid–gas multiphase flow region.
Meanwhile, the acoustic energy generated by the partially collapsed cavitation is absorbed by the cavitation that has not collapsed. On the other hand, many cavitation bubbles may gather in the impeller inlet, causing the blockage. Additionally, the incoming flow in the inlet pipeline interacts with the cavitation to form an impact, which promotes the downstream movement of the cavitation, leading the sound wave to propagate upstream. This is why acoustic energy reduction occurs in the cavitation process with the joint action of the two causes mentioned above.
4.3.2. Comparison of test and simulation results of TSPL of inlet cavitation-induced noise
The TSPLs of the two noise sources obtained from the numerical simulation and at each cavitation number were superimposed using the Section 2.2.3 method and compared with the TSPLs of the experimentally measured cavitation-induced noise analysis, as shown in Figure . According to the figure, the trends of CFD simulation and test results are consistent, indicating that this study’s constructed method for numerical simulation of cavitation-induced noise is reliable. The difference between the test and simulation curve is due to the combination of mechanical and cavitation noise in the centrifugal pump. This difference is also due to the sound transmission material and the acoustic window used during the test that loses some SPL value during the experiment. In addition, as the cavitation number decreases, the TSPL of the cavitation noise decreases, and the specific reasons for these outcomes are analysed and discussed in Section 4.3.1.
5. Conclusions
In this study, the numerical simulation of the cavitation-induced noise of centrifugal pumps was constructed based on the acoustic finite element method and spherical bubble radiation theory. The model pump’s unsteady cavitation flow and cavitation-induced noise characteristics were analysed by combining them with experimental measurements. The main conclusions are as follows:
The comparison between the test and simulation results shows that the relative errors of the simulated and test values of head and efficiency are within 5% of the whole flow range, indicating that the numerical simulation is consistent and credible. The cavitation characteristics predicted by the improved RZGB cavitation model are closer to the experimental values than the ZGB and Singhal cavitation models. This indicates that the improved RZGB cavitation model is reasonable and can be used for the numerical simulation of unsteady cavitation flow in model pumps. Moreover, the development of cavitation inside the model pump has a stronger influence on the vortex position in the impeller region, and the vortex has a promotional effect on the formation of cavitation. With the rated speed and three different flow rates, the pressure pulsation of the model pump outlet shows different variation laws with the decrease in cavitation number.
With the same cavitation number, the acoustic power value at the impeller blade’s suction side is larger than the blade’s pressure side. As the cavitation number decreases, the area covered by the cavitation at the blade’s suction side increases regularly, and the acoustic power value also increases. At this stage, the pulsating radiated noise of the cavitation volume is the primary noise source for the increase in acoustic power value.
With different cavitation numbers, the acoustic power of the model pump cavitation-induced noise is mainly distributed in the frequency of lower band 0∼2StBPF, and the principal frequency of the SPL is the blade-passing frequency and multiples. When the cavitation volume pulsation is not considered, the pre-inlet areas of the impeller are leading sound source areas of cavitation noise, and the intensity of SPL does not decay along the inlet section upstream. The frequency corresponding to the maximum peak of the SPL of the cavitation volume pulsating radiated noise is the cavitation shedding frequency. During the severe cavitation stage, the SPL and TSPL at the model pump inlet decreased with the reduction in cavitation number.
Our current study investigates the cavitation flow field and noise characteristics in centrifugal pumps at different operating points. However, our future study will focus on controlling the noise produced due to the cavitation phenomena.
Acknowledgements
The authors gratefully acknowledge the financial support provided by the National Natural Science Foundation of China (51976079, 12002136), the National Key R&D Program of China (2020YFC1512403), and Research Project of State Key Laboratory of Mechanical System and Vibration (MSV202201).
Disclosure statement
No potential conflict of interest was reported by the author(s).
Data availability statement
The data supporting this study’s findings are available from the corresponding author upon reasonable request.
Additional information
Funding
References
- Ali, A., Si, Q., Wang, B., Yuan, J., Wang, P., Rasool, G., Shokrian, A., Ali, A., & Zaman, M. A. (2022). Comparison of empirical models using experimental results of electrical submersible pump under two-phase flow: Numerical and empirical model validation. Physica Scripta. http://iopscience.iop.org/article/10.10881402-4896/ac6e96
- Ali, A., Si, Q., Yuan, J., Shen, C., Cao, R., Saad AlGarni, T., Awais, M., & Aslam, B. (2022). Investigation of energy performance, internal flow and noise characteristics of miniature drainage pump under water–air multiphase flow: Design and part load conditions. International Journal of Environmental Science and Technology, 19(8), 7661–7678. https://doi.org/10.1007/s13762-021-03619-1
- Ali, A., Yuan, J., Deng, F., Wang, B., Liu, L., Si, Q., & Buttar, N. A. (2021). Research progress and prospects of multi-stage centrifugal pump capability for handling gas–liquid multiphase flow: Comparison and empirical model validation. Energies, 14(4), 896. https://doi.org/10.3390/en14040896
- Bai, X., Cheng, H., & Ji, B. (2022). LES investigation of the noise characteristics of sheet and tip leakage vortex cavitating flow. International Journal of Multiphase Flow, 146, 103880. https://doi.org/10.1016/j.ijmultiphaseflow.2021.103880
- Baker, R. C., & Sorbie, I. (2001). A review of the impact of component variation in the manufacturing process on variable area (VA) flowmeter performance. Flow Measurement and Instrumentation, 12(2), 101–112. https://doi.org/10.1016/S0955-5986(01)00004-8
- Callenaere, M., Franc, J.-P., Michel, J.-M., & Riondet, M. (2001). The cavitation instability induced by the development of a re-entrant jet. Journal of Fluid Mechanics, 444, 223–256. https://doi.org/10.1017/S0022112001005420
- Cao, W., Jia, Z., Zhao, Z., & Zhou, L. (2022). Validation and simulation of cavitation flow in a centrifugal pump by filter-based turbulence model. Engineering Applications of Computational Fluid Mechanics, 16(1), 1724–1738. https://doi.org/10.1080/19942060.2022.2111363
- ČDina, M. (2003). Detection of cavitation phenomenon in a centrifugal pump using audible sound. Mechanical Systems and Signal Processing, 17(6), 1335–1347. https://doi.org/10.1006/mssp.2002.1514
- Chen, G., Wang, G., Hu, C., Huang, B., Gao, Y., & Zhang, M. (2015). Combined experimental and computational investigation of cavitation evolution and excited pressure fluctuation in a convergent–divergent channel. International Journal of Multiphase Flow, 72, 133–140. https://doi.org/10.1016/j.ijmultiphaseflow.2015.02.007
- Cheng, H., Long, X., Ji, B., Peng, X., & Farhat, M. (2021). A new Euler-Lagrangian cavitation model for tip-vortex cavitation with the effect of non-condensable gas. International Journal of Multiphase Flow, 134, 103441. https://doi.org/10.1016/j.ijmultiphaseflow.2020.103441
- Cheng, H.-y., Ji, B., Long, X.-p., Huai, W.-x., & Farhat, M. (2021). A review of cavitation in tip-leakage flow and its control. Journal of Hydrodynamics, 33(2), 226–242. https://doi.org/10.1007/s42241-021-0022-z
- Cheng, X., Li, Y., & Zhang, S. (2019). Effect of inlet sweepback angle on the cavitation performance of an inducer. Engineering Applications of Computational Fluid Mechanics, 13(1), 713–723. https://doi.org/10.1080/19942060.2019.1640134
- Chudina, M. (2003). Noise as an indicator of cavitation in a centrifugal pump. Acoustical Physics, 49(4), 463–474. https://doi.org/10.1134/1.1591303
- Ciochon, A., Kennedy, J., Leiba, R., Flanagan, L., & Culleton, M. (2023). The impact of surface roughness on an additively manufactured acoustic material: An experimental and numerical investigation. Journal of Sound and Vibration, 546, 117434. https://doi.org/10.1016/j.jsv.2022.117434
- Čudina, M., & Prezelj, J. (2009). Detection of cavitation in situ operation of kinetic pumps: Effect of cavitation on the characteristic discrete frequency component. Applied Acoustics, 70(9), 1175–1182. https://doi.org/10.1016/j.apacoust.2009.04.001
- Das, A., & Mahanwar, P. (2020). A brief discussion on advances in polyurethane applications. Advanced Industrial and Engineering Polymer Research, 3(3), 93–101. https://doi.org/10.1016/j.aiepr.2020.07.002
- Gao, F., Wang, H., & Wang, H. (2017). Comparison of different turbulence models in simulating unsteady flow. Procedia Engineering, 205, 3970–3977. https://doi.org/10.1016/j.proeng.2017.09.856
- Geng, L., & Escaler, X. (2020). Assessment of RANS turbulence models and Zwart cavitation model empirical coefficients for the simulation of unsteady cloud cavitation. Engineering Applications of Computational Fluid Mechanics, 14(1), 151–167. https://doi.org/10.1080/19942060.2019.1694996
- Ghasemian, M., & Nejat, A. (2015). Aero-acoustics prediction of a vertical axis wind turbine using Large Eddy Simulation and acoustic analogy. Energy, 88, 711–717. https://doi.org/10.1016/j.energy.2015.05.098
- Gong, J., Luo, W.-z., Wu, T.-c., & Zhang, Z.-y. (2022). Numerical analysis of vortex and cavitation dynamics of an axial-flow pump. Engineering Applications of Computational Fluid Mechanics, 16(1), 1921–1938. https://doi.org/10.1080/19942060.2022.2122570
- Gupta, P., & Parey, A. (2021). To investigate the influence of sound-absorbing materials on the transmission loss of double-wall panel. Materials Today: Proceedings, 44, 1500–1503. https://doi.org/10.1016/j.matpr.2020.11.691
- Hu, J., Ning, X., Zhao, W., Li, F., Ma, J., Zhang, W., Sun, S., Zou, M., & Lin, C. (2021). Numerical simulation of the cavitating noise of contra-rotating propellers based on detached eddy simulation and the Ffowcs Williams–Hawkings acoustics equation. Physics of Fluids, 33(11), 115117. https://doi.org/10.1063/5.0065456
- Hunt, J. C., Wray, A. A., & Moin, P. (1988). Eddies, streams, and convergence zones in turbulent flows. Studying Turbulence Using Numerical Simulation Databases, 2. Proceedings of the 1988 Summer Program.
- Ianniello, S., Muscari, R., & Di Mascio, A. (2013). Ship underwater noise assessment by the acoustic analogy. Part I: Nonlinear analysis of a marine propeller in a uniform flow. Journal of Marine Science and Technology, 18(4), 547–570. https://doi.org/10.1007/s00773-013-0227-0
- Jian, W., Yong, W., Houlin, L., Qiaorui, S., & Dular, M. (2018). Rotating corrected-based cavitation model for a centrifugal pump. Journal of Fluids Engineering, 140(11), 11. https://doi.org/10.1115/1.4040068
- Kadivar, E., Timoshevskiy, M. V., Nichik, M. Y., el Moctar, O., Schellin, T. E., & Pervunin, K. S. (2020). Control of unsteady partial cavitation and cloud cavitation in marine engineering and hydraulic systems. Physics of Fluids, 32(5), 052108. https://doi.org/10.1063/5.0006560
- Kamali-Moghadam, R., Javadi, K., & Kiani, F. (2016). Assessment of the LES-WALE and Zonal-DES turbulence models in simulation of the flow structures around the finite circular cylinder. Journal of Applied Fluid Mechanics, 9(2), 909-923. https://doi.org/10.18869/acadpub.jafm.68.225.24280
- Kinzel, M., Lindau, J., Peltier, L., Kunz, R., & Sankaran, V. (2007). Detached-Eddy simulations for cavitating flows. 18th AIAA Computational Fluid Dynamics Conference. American Institute of Aeronautics and Astronautics. https://doi.org/10.2514/6.2007-4098
- Kozmus, G., Zevnik, J., Hočevar, M., Dular, M., & Petkovšek, M. (2022). Characterization of cavitation under ultrasonic horn tip – Proposition of an acoustic cavitation parameter. Ultrasonics Sonochemistry, 89, 106159. https://doi.org/10.1016/j.ultsonch.2022.106159
- Kurańska, M., Barczewski, R., Barczewski, M., Prociak, A., & Polaczek, K. (2020). Thermal insulation and sound absorption properties of open-cell polyurethane foams modified with bio-polyol based on used cooking oil. Materials, 13(24), 5673. https://doi.org/10.3390/ma13245673
- Li, Y.-b., Fan, Z.-j., Guo, D.-s., & Li, X.-b. (2020). Dynamic flow behavior and performance of a reactor coolant pump with distorted inflow. Engineering Applications of Computational Fluid Mechanics, 14(1), 683–699. https://doi.org/10.1080/19942060.2020.1748720
- Li, Z., Qian, Z., & Ji, B. (2020). Transient cavitating flow structure and acoustic analysis of a hydrofoil with whalelike wavy leading edge. Applied Mathematical Modelling, 85, 60–88. https://doi.org/10.1016/j.apm.2020.04.004
- Lighthill, M. J. (1954). On sound generated aerodynamically II. Turbulence as a source of sound. Proceedings of the Royal Society of London. Series A. Mathematical and Physical Sciences, 222(1148), 1–32. https://doi.org/10.1098/rspa.1954.0049
- Lilley, G. M. (1994). The radiated noise from isotropic turbulence. Theoretical and Computational Fluid Dynamics, 6(5), 281–301. https://doi.org/10.1007/BF00311842
- Mohamed, M. H. (2016). Reduction of the generated aero-acoustics noise of a vertical axis wind turbine using CFD (Computational Fluid Dynamics) techniques. Energy, 96, 531–544. https://doi.org/10.1016/j.energy.2015.12.100
- Patil, A. D., & Baral, S. S. (2021). Process intensification of thumba methyl ester (biodiesel) production using hydrodynamic cavitation. Chemical Engineering Research and Design, 171, 277–292. https://doi.org/10.1016/j.cherd.2021.05.007
- Peng, X. X., Ji, B., Cao, Y., Xu, L., Zhang, G., Luo, X., & Long, X. (2016). Combined experimental observation and numerical simulation of the cloud cavitation with U-type flow structures on hydrofoils. International Journal of Multiphase Flow, 79, 10–22. https://doi.org/10.1016/j.ijmultiphaseflow.2015.10.006
- Proudman, I., & Taylor, G. I. (1952). The generation of noise by isotropic turbulence. Proceedings of the Royal Society of London. Series A. Mathematical and Physical Sciences, 214(1116), 119–132. https://doi.org/10.1098/rspa.1952.0154
- Qiaorui, S., Ali, A., Biaobiao, W., Wang, P., Bois, G., Jianping, Y., & Kubar, A. A. (2022). Numerical study on gas–liquid two phase flow characteristic of multistage electrical submersible pump by using a novel multiple-size group (MUSIG) model. Physics of Fluids, 34(6), 063311. https://doi.org/10.1063/5.0095829
- Reisman, G. E., Wang, Y. C., & Brennen, C. E. (1998). Observations of shock waves in cloud cavitation. Journal of Fluid Mechanics, 355, 255–283. https://doi.org/10.1017/S0022112097007830
- Sakamoto, N., & Kamiirisa, H. (2018). Prediction of near field propeller cavitation noise by viscous CFD with semi-empirical approach and its validation in model and full scale. Ocean Engineering, 168, 41–59. https://doi.org/10.1016/j.oceaneng.2018.08.061
- Sedlar, M., Ji, B., Kratky, T., Rebok, T., & Huzlik, R. (2016). Numerical and experimental investigation of three-dimensional cavitating flow around the straight NACA2412 hydrofoil. Ocean Engineering, 123, 357–382. https://doi.org/10.1016/j.oceaneng.2016.07.030
- Seol, H., Suh, J.-C., & Lee, S. (2005). Development of hybrid method for the prediction of underwater propeller noise. Journal of Sound and Vibration, 288(1), 345–360. https://doi.org/10.1016/j.jsv.2005.01.015
- Sezen, S., Atlar, M., Fitzsimmons, P., Sasaki, N., Tani, G., Yilmaz, N., & Aktas, B. (2020). Numerical cavitation noise prediction of a benchmark research vessel propeller. Ocean Engineering, 211, 107549. https://doi.org/10.1016/j.oceaneng.2020.107549
- Shimizu, A., Furukawa, M., Kato, K., & Asahina, Y. (2004). Polyether polyurethane. Google Patents.
- Shin, S., Hong, J.-W., Nagarathinam, D., Ahn, B.-K., & Park, S.-G. (2021). Tip vortex cavitation and induced noise characteristics of hydrofoils. Applied Sciences, 11(13), 5906. https://doi.org/10.3390/app11135906
- Si, Q., Ali, A., Yuan, J., Fall, I., & Muhammad Yasin, F. (2019). Flow-induced noises in a centrifugal pump: A review. Science of Advanced Materials, 11(7), 909–924. https://doi.org/10.1166/sam.2019.3617
- Si, Q., Bois, G., Liao, M., Zhang, H., Cui, Q., & Yuan, S. (2020). A comparative study on centrifugal pump designs and two-phase flow characteristic under inlet gas entrainment conditions. Energies, 13(1), 65. https://doi.org/10.3390/en13010065
- Si, Q., Shen, C., Ali, A., Cao, R., Yuan, J., & Wang, C. (2019). Experimental and numerical study on gas-liquid two-phase flow behavior and flow induced noise characteristics of radial blade pumps. Processes, 7(12), 920. https://doi.org/10.3390/pr7120920
- Si, Q., Shen, C., He, X., Li, H., Huang, K., & Yuan, J. (2020). Numerical and experimental study on the flow-induced noise characteristics of high-speed centrifugal pumps. Applied Sciences, 10(9), 3105. https://doi.org/10.3390/app10093105
- Si, Q., Wang, B., Yuan, J., Huang, K., Lin, G., & Wang, C. (2019). Numerical and experimental investigation on radiated noise characteristics of the multistage centrifugal pump. Processes, 7(11), 793. https://doi.org/10.3390/pr7110793
- Soyama, H. (2021). Luminescence intensity of vortex cavitation in a Venturi tube changing with cavitation number. Ultrasonics Sonochemistry, 71, 105389. https://doi.org/10.1016/j.ultsonch.2020.105389
- Sun, H., Luo, Y., Yuan, S., & Yin, J. (2018). Hilbert spectrum analysis of unsteady characteristics in centrifugal pump operation under cavitation status. Annals of Nuclear Energy, 114, 607–615. https://doi.org/10.1016/j.anucene.2018.01.004
- Sun, T., Ding, Y., Huang, H., Xie, B., & Zhang, G. (2021). Numerical study on the effects of modulated ventilation on unsteady cavity dynamics and noise patterns. Physics of Fluids, 33(12), 123307. https://doi.org/10.1063/5.0067559
- Sun, X., & Wang, X. (2021). Chapter 1 - Basic equations of aeroacoustics. In X. Sun & X. Wang (Eds.), Fundamentals of aeroacoustics with applications to aeropropulsion systems (pp. 1–32). Academic Press. https://doi.org/10.1016/B978-0-12-408069-0.00001-4
- Tiuc, A. E., Nemeş, O., Vermeşan, H., & Toma, A. C. (2019). New sound absorbent composite materials based on sawdust and polyurethane foam. Composites Part B: Engineering, 165, 120–130. https://doi.org/10.1016/j.compositesb.2018.11.103
- Wang, Y., Mikkola, T., & Hirdaris, S. (2022). A fast and storage-saving method for direct volumetric integration of FWH acoustic analogy. Ocean Engineering, 261, 112087. https://doi.org/10.1016/j.oceaneng.2022.112087
- Wang, Z., Cheng, H., & Ji, B. (2021). Euler–Lagrange study of cavitating turbulent flow around a hydrofoil. Physics of Fluids, 33(11), 112108. https://doi.org/10.1063/5.0070312
- Wei, A., Wang, S., Gao, X., Qiu, L., Yu, L., & Zhang, X. (2022). Investigation of unsteady cryogenic cavitating flow and induced noise around a three-dimensional hydrofoil. Physics of Fluids, 34(4), 042120. https://doi.org/10.1063/5.0088092
- Wu, Q., Huang, B., Wang, G., Cao, S., & Zhu, M. (2018). Numerical modelling of unsteady cavitation and induced noise around a marine propeller. Ocean Engineering, 160, 143–155. https://doi.org/10.1016/j.oceaneng.2018.04.028
- Wu, S., Wu, Y., Tian, J., & Ouyang, H. (2022). On the cavitation-induced collapse erosion of a turbofan fuel pump. Engineering Applications of Computational Fluid Mechanics, 16(1), 1048–1063. https://doi.org/10.1080/19942060.2022.2067243
- Yang, J., Xie, T., Liu, X., Si, Q., & Liu, J. (2021). Study of unforced unsteadiness in centrifugal pump at partial flow rates. Journal of Thermal Science, 30(1), 88–99. https://doi.org/10.1007/s11630-019-1241-2
- Yu, A., Wang, X., Zou, Z., Tang, Q., Chen, H., & Zhou, D. (2019). Investigation of cavitation noise in cavitating flows around an NACA0015 hydrofoil. Applied Sciences, 9(18), 3736. https://doi.org/10.3390/app9183736
- Yu, L., Zhao, W., Wan, D., & Wu, J. (2022). Nonlinear noise of hydrofoil cavitation considering sound velocity variation and phase transitions. Ocean Engineering, 264, 112506. https://doi.org/10.1016/j.oceaneng.2022.112506
- Zhang, X.-Y., Jiang, C.-X., Lv, S., Wang, X., Yu, T., Jian, J., Shuai, Z.-J., & Li, W.-Y. (2021). Clocking effect of outlet RGVs on hydrodynamic characteristics in a centrifugal pump with an inlet inducer by CFD method. Engineering Applications of Computational Fluid Mechanics, 15(1), 222–235. https://doi.org/10.1080/19942060.2021.1871961
Appendix A
(2) Improved RZGB cavitation model:
The relationship between the maximum radius of cavitation (Rm), the Weber number (We), and the blade pitch (t) is:
(A1)
(A1) The Weber number (We) is defined as:
(A2)
(A2) where L∞ and u represent the characteristic length and velocity separately. Let’s take the blade inlet circumferential velocity () as the characteristic velocity and the blade pitch (t) as the characteristic length. The expressions for the characteristic velocity and characteristic length are as follows:
(A3)
(A3)
(A4)
(A4) where D1 indicates the intersection between the blade inlet diameter and the impeller front cover plate, mm; n represents the pump’s rotational speed, r/min; z shows the number of impeller blades. According to the above Equation, the maximum radius of cavitation in the centrifugal pump can be defined concerning the impeller geometry and pump speed as:
(A5)
(A5) In addition, studies have shown that the maximum size of the cavitation is inversely proportional to the square of the turbulent kinetic energy, and the average size of the cavitation is about 0.6 times its maximum size. Considering the above factors, the size of the cavitation inside the model pump can be finally defined as:
(A6)
(A6) From the above Equation, it can be seen that the size of the cavitation bubble depends on the blade numbers and rotational speed of the centrifugal pump. Through the above relationship, we finally obtain a cavitation model of RZGB that considers the rotational effect and geometric characteristics of the impeller of the model pump. Moreover, the expressions of its evaporation and condensation source term are as follows:
(A7)
(A7)
(A8)
(A8)