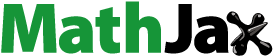
Abstract
To create a highly efficient photovoltaic-thermal (PV-T) system and maximise the energy and exergy efficiency, this study aims to propose an innovative configuration of a PV-T system comprising wavy tubes with twisted-tape inserts. Following the validation of a numerical model, a parametric study has been conducted to assess the geometrical effects of twisted tape and wavy tubes, as well as the coolant fluid type and velocity, on the overall performance of a PV-T system, located in Shiraz, Iran. It is found that employing twisted tape improves the energy and exergy efficiency by approx. 6.3%. The best configuration yields 12.4% and 16.8% increase in energy and exergy efficiency compared to conventional PV systems. This is achieved at 15% volumetric concentration of microencapsulated phase change material slurry. The monthly variation of global horizontal irradiance in Shiraz highly affected the energy and exergy efficiency of PV-T, with July and October exhibiting the most efficient months, corresponding to 90% and 11.3%, respectively.
Highlights
Energy and exergy of photovoltaic-thermal system with twisted tape and wavy tubes.
Reduction in the temperature of the PV panel by 8.3°C compared to the base case.
12.4% and 16.8% increase in energy and exergy efficiency in the optimum configuration.
Higher energy and exergy efficiency from microencapsulated phase change material.
Nomenclature
A | = | Area |
c | = | Volumetric concentration |
= | Specific heat | |
Ex | = | Exergy (w) |
= | Generation of kinetic | |
g | = | Gravity |
h | = | Heat transfer coefficient |
H | = | Daily irradiation |
= | Twisted tape height (m) | |
I | = | Hourly irradiation |
= | Daily clearness index | |
= | Monthly daily clearness index | |
K | = | Turbulent kinetic energy (J/kg) |
k | = | Thermal conductivity ( |
= | Boltzmann constant | |
= | 180° twisted pitch (m) | |
= | Mass flow rate (kg/s) | |
P | = | Packing factor/pressure (pa) |
q | = | Heat flux |
R | = | Ratio of total radiation on a tilted plane to the horizontal plane |
= | Monthly average of R | |
= | Ratio of beam radiation on a tilted plane to the horizontal plane | |
= | Ratio of diffuse radiation on a tilted plane to the horizontal plane | |
r | = | radius |
= | Ratio of diffuse radiation in an hour to diffuse in a day | |
= | Ratio of total radiation in an hour to total in a day | |
= | Solar radiation intensity ( | |
T | = | temperature |
= | Velocity component (m/s) | |
V | = | volume |
= | Velocity vector | |
= | Width of the twisted tape (mm) | |
= | Fluctuation dilation |
Greek
= | absorptivity | |
= | Temperature coefficient | |
= | Kronecker delta/declination | |
= | Emissivity/turbulent dissipation (W/kg) | |
= | Surface azimuth angle | |
= | Solar azimuth angle | |
= | Efficiency | |
= | Volume fraction/latitude | |
= | viscosity | |
= | Density | |
= | Hour angle | |
= | Sunset hour angle | |
= | Stefan Boltzmann constant |
Superscripts
= | Mean value | |
= | Random fluctuation |
Subscripts
a | = | Ambient |
AP | = | Absorber plate |
b | = | Beam |
c | = | Convection |
d | = | Diffuse |
e | = | Electrical |
ex | = | Exergy |
f | = | Carrier fluid |
fr | = | Friction |
in | = | Tube inlet |
l | = | liquid |
m | = | Mean thermodynamic/melting |
MP | = | Micro-encapsulated PCM |
MPS | = | Micro-encapsulated PCM slurry |
nf | = | Nanofluid |
np | = | Nanoparticle |
out | = | Tube outlet |
p | = | particle |
PV | = | Photovoltaic panel |
ref | = | Reference |
s | = | solid |
th | = | thermal |
tu | = | Turbulent |
Abbreviation
HTF | = | Heat transfer fluid |
MPCM | = | Micro-encapsulated PCM |
PCM | = | Phase change material |
PEC | = | Performance Evaluation Criteria |
PV-T | = | Photovoltaic/thermal |
UDF | = | User Define Function |
1. Introduction
A key challenge associated with using photovoltaic (PV) modules to produce electricity is an increased panel temperature, leading to reduced electrical efficiency (Essam et al., Citation2022). To overcome this challenge, heat transfer fluid (HTF) is used underneath the PV panel to cool it down. The heated fluid can then be utilised for thermal applications (Huang et al., Citation2020). These photovoltaic-thermal (PV-T) systems are cutting-edge renewable-powered technology that combines the power of PV cells and thermal collectors in one integrated system (Li et al., Citation2022). PV-T systems have gained increasing attention in recent years as an efficient and cost-effective way to meet energy demands while reducing greenhouse gas emissions. They can be utilised in a wide range of applications such as space heating, water heating, building, and industrial process heat.
The geometrical configuration of the PV-T system is crucial for achieving higher efficiency (Kim & Tae, Citation2022). Researchers have evaluated various configurations to improve the performance of PV-T systems. Shahsavar et al. (Citation2020) experimentally investigated a PV-T system equipped with cooling tubes with different shapes. They found that electrical efficiency was increased by 12% by adding a coolant tube. Moreover, the use of nanoparticles enhanced the exergy and energy efficiencies by 7% and 6.6%, respectively. Tomar et al. (Citation2019) employed glass-to-glass PV modules in the PV-T system and found a maximum hourly-averaged electrical efficiency of ∼13%. In another study, Hossain et al. (Citation2019) focused on the use of serpentine pipe PV-T collector design. The results indicated a 5.34% increase in electrical efficiency with 3.92°C reduction in the PV temperature. Employing polycrystalline and monocrystalline panels in PV-T systems, Özakin and Kaya (Citation2019) achieved 55% and 70% increase in energy efficiency and a 70% and 30% increase in exergy efficiency compared to conventional PV-T systems, respectively.
Wavy tubes have shown great potential to improve the thermal performance compared to straight tubes. This is due to an increased heat exchange area and enhanced mixing, resulting in a more uniform temperature distribution and a greater net efficiency in the PV panel. Eisapour et al. (Citation2020) numerically evaluated the use of wavy tubes in PV-T modules. In their best design, the energy and exergy efficiencies were increased by 6.1% and 4.3%, respectively. In another study by the same research group (Eisapour et al., Citation2021), the authors examined an innovative layout of wavy tubes with ascending and descending amplitudes. The authors enhanced electrical and thermal efficiencies from 10.94% and 61.04% in the case of a straight tube to 11.32% and 65.21%, respectively. The authors indicated that using SiC and micro-encapsulated phase change material (MPCM) (MPCM-28) as a working fluid instead of water improved the electrical efficiency by 0.4%.
The use of vortex generators to create swirling patterns and disrupt the formation of boundary layers has gained significant attention in recent years (Darbari et al., Citation2020; and Tian et al., Citation2020). Aridi et al. (Citation2022) focused on the hydrothermal performance of a trapezoidal vortex generator in a concentric tube heat exchanger through numerical simulation. They improved the heat transfer ratios and thermal factor by 97% and 210%. Using a longitudinal vortex generator, Carpio and Valencia (Citation2021) increased the thermal performance of the system by 52%. Arasteh et al. (Citation2021) numerically analysed the hydrothermal performance of twisted rotational tape pitch distance inside a heat-exchanging tube. The results showed a higher performance evaluation criterion (PEC) at lower Reynolds in the case of rotating twisted tape. However, the maximum PEC was found at a Reynolds number of 1000 and a pitch distance of L/6 for a stationary twisted tape. Mashayekhi et al. (Citation2021) corroborated the same finding of Arasteh et al. (Citation2021) in terms of better enhancement in the thermal characteristics of rotating twisted tape at lower Reynolds numbers under the laminar regime, 32.8–39.6% increase in the Nusselt number at Reynolds number of 250.
The cooling fluid also plays a crucial role in the overall electrical performance of the PV-T system (Akbar et al., Citation2021; Moore & Wei, Citation2021). Menon et al. (Citation2022) evaluatean unglazed PV-T system’s electrical and thermal performance using water and copper oxide-based nanofluid. They reduced the panel temperature by 15°C and 23.7°C and achieved an electrical efficiency of 14.6% and 17.6% when the system was cooled with water and nanofluid, respectively. Some researchers investigated the impact of using two nanoparticles (Ghalambaz et al., Citation2020a; Mehryan et al., Citation2020), composite nanoparticles (Ghalambaz et al., Citation2019; Ho et al., Citation2020), or non-Newtonian fluids (A. Saleem et al., Citation2020; S. Saleem et al., Citation2021) on the heat transfer enhancement of PV-T systems. In this regard, Abdallah et al. (Citation2019) reduced the temperature of the PV panel by 12°C – resulting in a maximum system efficiency of 61% – using multi-wall carbon nanotube nanofluid. Fu et al. (Citation2021) introduced a PV-T collector that utilises two types of coolant fluids: water and MPCM slurry. The use of MPCM slurry as a coolant fluid results in a more considerable decrease in the temperature of PV-T cells, leading to 13.5% and 0.8% increase in the maximum thermal efficiency and average electrical efficiency.
Reviewing the literature highlights that many efforts have been made to develop effective thermal management systems for high-performance PV-T systems. However, despite recent advances, further research and development are required to gain sufficient insight into the combined heat transfer enhancement techniques to boost the performance of PV-T systems. While the use of twisted tapes in other heat transfer applications are emerging in recent years (e.g. Ghalambaz et al., Citation2020b; Mashayekhi et al., Citation2020), the increase of convective heat transfer coefficients of PV-T systems still remains not fully understood. Therefore, to address this research gap, the present study aims to investigate the use of twisted tape inserts in wavy tubes to evaluate the performance of PV-T systems from an energy and exergy standpoint. The present work examines the effect of twisted tape/wavy tube geometrical parameters and different types of coolant fluid on the system performance. Unlike previous studies that only considered solar radiation intensity as a constant variable, this research employs an isotropic sky module to calculate location-specific solar radiation intensity for a tilted PV-T system, allowing for a more accurate monthly performance assessment of the proposed configuration.
2. Model description
This study investigates a PV-T system incorporating wavy cooling tubes equipped with twisted tape inserts. Figure shows the proposed PV-T system together with the PV panel, the absorber plate, cooling tubes, and the boundary conditions. In the PV-T collector, the PV module is located on the upper surface, the absorber plate is in the middle, and the cooling tubes are placed at the bottom. As illustrated in Figure , the boundary condition of the upper surface is considered to be the incoming solar irradiance and heat losses through convection and radiation mechanisms.
The schematic of the present module is shown in Figure . As seen in Figure (a), the PV system benefits from five wavy tubes under the panel for cooling purposes. All surfaces are considered insulated except the upper layer, which absorbs solar energy from the Sun. Due to the symmetrical condition, only 1/5 of the whole system is considered for the computational domain (Ekramian et al., Citation2014). The length of the absorber plate is 2000 mm, from which 1640 mm of the absorber plate is enclosed by the PV module (Eisapour et al., Citation2020). The absorber plate absorbs the solar thermal energy depending on the absorber’s solar radiation intensity and absorptivity.
Figure shows the characteristics of the proposed twisted tape inserts. In this figure, Lt is the pitch of the twisted tape, Wt is the width of the twisted tape, and Ht is the height. Table presents the characteristics of various proposed cases considered in this research. Case 1 is the system with a straight tube, which is assumed to be the base case in this study. Cases 2 and 3 are the system with wavy tubes without twisted tape inserts. Adding twisted tapes to cases 1, 2, and 3 forms cases 4, 5, and 6, respectively.
Table 1. Characteristics of different cases considered in this research.
3. Governing equation
3.1. Assumptions
In order to make the PV-T numerical modelling more straightforward, the following hypotheses are applied:
The fluids are incompressible.
The problem is assumed to be steady-state.
The flow regimes in cases 1–3 and cases 4–6 are laminar and turbulent, respectively. The latter is due to the presence of twisted tube inserts.
The external surface of the cooling tube and the absorber plate is well insulated.
Nanofluid and MPCM slurries are in thermal equilibrium and single-phase in the calculations (Demir et al., Citation2011).
Convection and radiation heat losses are considered.
No thermal resistance between the PV panel and absorber plate, leading to identical temperatures at the interface (Fontenault & Gutierrez-Miravete, Citation2012).
3.2. Laminar model
The continuity, momentum, and energy equations for a laminar flow are written as follows (Liaw et al., Citation2021):
(1)
(1)
(2)
(2)
(3)
(3)
The energy equation for the solid part is given as follows:
(4)
(4)
3.3. Turbulence model
Using twisted tape inserts inside the tube generates vortices, resulting in the transition from laminar to turbulent flow (Liaw et al., Citation2021). To accommodate this, the Realisable k-ϵ enhanced wall treatment model is used as below (Liaw et al., Citation2021):
(5)
(5)
(6)
(6)
(7)
(7)
(8)
(8)
(9)
(9) where k is the turbulent kinetic energy, ϵ is the turbulence dissipation rate, Gk is the kinetic enhancement due to buoyancy, YM is the fluctuation dilation in the compressible turbulence regime, and Sk is a source term specified by the user. The values of
,
,
are constants in these equations (Liaw et al., Citation2021). Further information on performance of different two-equation turbulence models can be found in several publications including those by the present authors (Keshmiri et al., Citation2008, Citation2015, Citation2016)
3.4. Boundary conditions
The upper surface of the system absorbs solar energy from the Sun. A fraction of this energy is absorbed, transferred to the HTF, and a fraction is dissipated due to convection and radiation losses. The net heat absorbed where the plate is covered by the PV cell is calculated as (Du et al., Citation2016):
(10)
(10) where
and
and
(Kumar & Mullick, Citation2010). The net heat absorbed by the sections of the absorber plate not being covered by PV cells is obtained by:
(11)
(11)
The ambient conditions are considered for Shiraz, a southern city in Iran, located at 29.59 N and 52.58 E. Table illustrates Shiraz’s irradiance intensity and atmospheric temperature in 2020 according to the weather data file. Further information is provided in Appendix A.
Table 2. The irradiance intensity and atmospheric temperature for Shiraz city in 2020.
The surfaces in contact are entirely adiabatic. The shared surfaces between the absorber plate and the PV module are symmetrical. Velocity inlet and pressure outlet boundary conditions are assumed at the inlet and outlet of the fluid. The inlet temperature of the HTF is considered ambient for all cases (298.15 K). On the tube walls, a no-slip boundary condition is employed. Table displays the properties of the PV panel and absorber plate. The electrical reference efficiency of the PV cell, , is evaluated when the PV temperature approaches the reference temperature.
Table 3. Absorber plate and PV panel properties (Bhattarai et al., Citation2012).
3.5. Properties of different working fluids
The working fluids in this study include (i) pure water, (ii) Ag-water nanofluid, (iii) MPCM slurry, and (iv) MPCM nano-slurry. For Ag-water nanofluid and MPCM nano-slurry, a user-defined function (UDF) is developed to calculate the thermal conductivity of the nanofluid and the specific heat transfer coefficient of MPCM slurry as a function of temperature.
3.5.1. Properties Ag-water nanofluid
The properties of the nanofluids are calculated as follows (Kakaç & Pramuanjaroenkij, Citation2009):
(12)
(12)
(13)
(13) For
, the viscosity of nanofluid is calculated as (Mishra et al., Citation2014):
(14)
(14) The effective thermal conductivity can calculate as (Xuan et al., Citation2003):
(15)
(15)
The thermophysical properties of Ag nanoparticles are listed in Table (Öğüt, Citation2009). The properties of water are considered at 298.15 K.
Table 4. The properties of Ag nanoparticles (M. Eisapour et al., Citation2020).
3.5.2. MPCM slurry
The PCM material in this study is hydrocarbon n-octadecane. Table presents the properties of the MPCM slurry, the PCM and water composite, and the base fluid. According to the theoretical homogenous models and experimental studies, MPCM slurry properties are given as follows (Alquaity et al., Citation2012):
(16)
(16) The MPCM slurry viscosity is obtained from (Vand, Citation1945):
(17)
(17)
Table 5. Properties of n-octedane microencapsulate in this research (Hasan, Citation2011).
The effective thermal conductivity is calculated from the Maxwell model (Alquaity et al., Citation2012):
(18)
(18) The bulk-specific heat is calculated as (Alquaity et al., Citation2012):
(19)
(19)
It should be mentioned that melting occurs over the temperature range of 297–302 K.
3.5.3. MPCM nano-slurry
In this case, the Ag-water nanofluid is considered a carrier fluid combined with MPCM – containing 5% Ag-water nanofluid and 15% MPCM. Eqs (12–15) and Eqs. (16–19) estimates the average properties of nanofluid and MPCM nano-slurry, respectively. The property of fluids is listed in Table . It is to be noted that the properties such as thermal conductivity for nanofluid and MPCM nanofluid and specific heat for MPCM slurry/nano-slurry are not known at the beginning since these parameters are the function of fluid temperature. To address this, a UDF is employed to calculate these properties.
Table 6. Properties of considered HTFs at 298.15 K.
3.6. Energy analysis
The electrical and thermal efficiencies of the PV panel are defined as (Yu et al., Citation2019):
(20)
(20)
(21)
(21) where
is the packing factor which is considered equal to 0.82.
and
are the panel efficiency and the first law efficiency calculated as (Ji et al., Citation2007):
(22)
(22)
(23)
(23)
3.7. Exergy efficiency
The second thermodynamic law is adopted for the exergy analysis. The energy analysis shows the quantity of energy, while the exergy analysis shows the quality of energy. The exergy balance for the PV-T system can be calculated as (Demirel, Citation2013):
(24)
(24)
(25)
(25) Eq. (24) can be written as:
(26)
(26) The term in the bracket is useful exergy. The exergy efficiency is then given as follows (Khanjari et al.):
(27)
(27)
The electrical, thermal, and solar exergy are calculated as follows (Wu et al., Citation2015):
(28)
(28)
(29)
(29) where
(30)
(30) The solar exergy absorbed by the PV panel is calculated as (Khanjari et al., Citation2016):
(31)
(31) where
is set to 5774 K.
4. Numerical method and validation
After creating the geometry using Ansys Design Modeller, Ansys Meshing software is used to generate the mesh. The SIMPLE algorithm is used to solve the pressure field, and the QUICK scheme is used to discretize the momentum and energy equations. The residual values for the convergence of continuity and momentum are , while for the energy equation, it is
.
The geometry is divided into blocks to achieve the structured mesh, and then the geometry is meshed using the swipe element method. Figure shows the generated mesh for case 3 as an example. This figure shows that mesh size is refined in the vicinity of solid-fluid interfaces due to larger velocity/temperature gradients. The results of grid independency for case 3 are shown in Figure . The total number of mesh elements is varied between 830,000 and 4,560,000. The outlet and PV temperatures are chosen for the purpose of mesh independency checks. As seen in this figure, there is a marginal difference in the temperature by further refining the mesh beyond 3,418,000 grids. As such, this number of grids is chosen for all subsequent simulations.
For model validation, the results are compared with the data published in the literature for both laminar and turbulent flow. To validate the laminar flow model (cases 1–3), the numerical results of Yu et al. (Citation2019) are used, who used straight tubes under the PV module to cool down the panel and increase the electrical/thermal efficiency of the PV cell. For this purpose, they used a microencapsulated slurry with water-based fluid. The length and width of the module considered in their research were 2000 mm and 1000 mm, of which 1640 mm was covered by the panel. The length of the cooling tube is 2000 mm, while its diameter is 8 mm. The temperature of the cooling fluid at the inlet is 298.15°C, and the solar radiation intensity is 1000 W/m2. Figure shows the variation in PV temperature relative to the water inlet velocity for the present study and Yu et al. (Citation2019) model. As shown, the results follow a similar trend with acceptable accuracy.
Figure 6. The validation study of the PV-T system compared with Yu et al. (Citation2019).

The validation of the turbulent flow (cases 4–6) is performed with the numerical results of Liaw et al. (Liaw et al., Citation2021). In their research, twisted tape inserts in straight and helical tubes were studied using the k-ϵ model to solve the flow equations. The tube length was 1.258 m for the straight tube, and in the case of the helical tube, the coil pitch was 0.02 m. In addition, the width and height of the twisted tape were considered 0.4 and 10 mm, respectively. The operational conditions, including inlet temperature, wall temperature, and outlet pressure, were 298, 353 K, and zero relative pressure, respectively. Figure illustrates the variation of the heat transfer coefficient with the Reynolds number for the straight pipe, where good agreement is obtained between the results.
Figure 7. The twisted tape and turbulence model validation study compared with Liaw et al. (Citation2021).

The accuracy of the model in terms of nanofluid flow is assessed with the numerical study of Khanjari et al. (Citation2016). The proposed PV-T model consisted of a glass cover, a set of PV cells, a thermal sheet, and a tube collector in a header and riser configuration. To reduce the computational time, the model is simplified to include one riser tube just. In addition, the boundary condition and geometry configuration are adjusted according to Yu et al. (Citation2019) study. Figure shows the outlet temperature as a function of inlet velocity. The results indicate an acceptable agreement with those reported by Khanjari et al. (Citation2016).
Figure 8. The validation study of using Nanofluids in PV-T system compared with Khanjari et al. (Citation2016).

The MPCM slurry fluid is validated with the experimental data of Chen et al. (Citation2008). The authors employed the MPCM slurry passing through a circular stainless-steel tube for cooling the PV module with constant heat flux. The same physical domain is used for the model validation. The MPCM concentration and particle diameters are 15.8% and 8.2 (Chen et al., Citation2008). Figure shows the Nusselt number as a function of cylinder dimensionless length for the present work and those obtained by Chen et al. (Citation2008) under the constant heat flux of
. The comparison of the results shows acceptable accuracy of the present model.
Figure 9. Validation of MPCM slurry thermal performance in a cylindrical cavity with Chen et al. (Citation2008).

5. Results and discussions
The panel temperature and heat absorbed by the HTF for case 4 are compared against case 1 to assess the advantages of using twisted tape to enhance the cooling performance of a PV panel in a straight tube. This is followed by a detailed sensitivity analysis of geometrical parameters of twisted tape/wavy tube, types of coolant fluid, and monthly solar irradiation intensity on the overall exergy and exergy efficiencies of the PV-T systems.
5.1. Effect of twisted tape inserts in a straight tube
Figure shows that the panel temperature is higher when there is no twisted tape inside the tube, reaching the maximum temperature of 326 K compared to 320 K with twisted tape. This is attributed to the twisted tape creating a rotational motion in the flow near the wall, which generates secondary flow, enhances flow mixing, and disturbs the boundary layer (Mashayekhi et al., Citation2022). This, in turn, leads to a reduction in the PV panel temperature and an increase in the electrical efficiency of the PV panel.
Figure 10. Contours of the temperature distribution in the PV-T system with (a) a straight tube and (b) a straight tube with a twisted tape.

Figure shows the velocity contours at different cross-sections of a straight tube with/without twisted tape at 0.1 m/s inlet velocity (cases 4 and 1). The minimum and maximum velocities are obtained near the tube wall and centre of the tube in the case without twisted tape. However, when the twisted tape is inserted inside the tube, the boundary layer is disturbed, and the thickness of the boundary layer is reduced due to enhanced mixing effects (Ghalambaz et al., Citation2020b).
Figure 11. Cross-sectional velocity contours for straight tube (case 1) and straight tube with twisted tape insert (case 4) at u = 0.1 m/s.

Table lists the values of the average PV temperature as well as the total heat absorption by the cooling fluid with and without twisted tape in a straight tube at an intake velocity of 0.1 m/s (cases 4 and 1). The solar radiation intensity is assumed to be 778 W/m2 from the weather data file for Shiraz in October. The panel temperature decreases by 4.1 K, while the net heat absorbed by HTF increases by 36.3 W for a case with twisted tape compared to the base case.
Table 7. Values of the average PV temperature as well as the total heat absorption by the cooling fluid for the PV-T system with straight with and without twisted tape.
Table 8. The average PV temperature and the total heat absorption by the cooling fluid for the PV-T system with straight with and without twisted tape for different inlet velocities.
Figure (a) shows the energetic and exergetic efficiencies of the PV module for cases 4 and 1. According to Eq. (10), reducing the PV panel temperature is accompanied by a reduction in the temperature difference between the panel and the environment and, as a result, lower heat loss due to convection and radiation. The energy and exergy efficiencies increase when the twisted tape is inserted inside the straight tube. The thermal, electrical, and overall energy efficiencies increase by 6.8%, 4.1%, and 6.2%, while the thermal, electrical, and overall exergy efficiencies increase by 28%, 2%, and 6.3% in the case of twisted tape as compared to the base case, respectively.
Figure 12. The thermal, electrical, and overall efficiencies of the straight tube with and without twisted tape (cases 4 and 1) (a) energy efficiency and (b) exergy efficiency.

The results of this study suggest that the use of a twisted tape inside a straight tube under a PV panel is an effective method for enhancing the cooling performance of the panel and increasing the overall energy and exergy efficiencies of the system.
5.2. Effect of fluid velocity
The effect of inlet velocity ranging from 0.05 m/s to 0.25 m/s is investigated in this section. Figure shows the fluid temperature distribution for cases 4 and 1 under various values of inlet velocity. Inserting twisted tapes reduces the cross-sectional area of the cooling channel, increasing the flow velocity and centrifugal force – leading to a better redirection of the core flow toward the heated wall and a reduction in the fluid flow temperature. At a lower inlet velocity of 0.05 m/s, the generated centrifugal force is not strong enough to lower the PV panel temperature. However, moving to higher Reynolds numbers increases the flow velocity and momentum of the fluid – assisting the fluid in energy absorption from the heated wall and reduction in the PV panel temperature.
Figure 13. Temperature distribution of the PV panel at different inlet fluid velocities for the straight tube with and without twisted tape (cases 4 and 1).

Table lists the average PV temperature as well as the total heat absorption by the cooling fluid for different fluid velocities considering the mean solar radiation in October. It can be noticed that at higher inlet velocities, the panel temperature decreases, and the heat absorbed by the cooling fluid increases. Due to the secondary flow formation and the vortex generation, heat transfer in the twisted tape case is more significant. As a case in point, the change in the inlet velocity from 0.05 to 0.1 m/s is associated with the increase in the heat absorbed rate by 21.71% in case 4 and by 8.21% in case 1.
Figure 14. Effect of inlet velocity on (a) thermal and electrical energy efficiencies, (b) thermal and electrical exergy efficiencies, and (c) overall energetic and exergetic efficiencies of the PV module.

Figure (a) shows the PV-T system thermal and electrical energy efficiencies for different inlet velocities of the fluid. Increasing the inlet velocity increases both the thermal and electrical efficiencies. At 0.1 m/s, the thermal and electrical efficiencies of a straight tube with a twisted tape are 6.9% and 2% higher than that for the base case because of the higher reduction in the PV module temperature as a result of the enhancement in the heat transfer coefficient.
Figure (b) shows the PV-T system thermal and electrical exergy efficiencies for different velocities. Increasing the inlet velocity decreases the thermal exergy efficiency and increases the electrical exergy efficiency. The electrical exergy efficiency of a PV module with twisted tape is higher than that of a PV module without twisted tape. As velocity increases from 0.05 to 25
, the thermal exergy decreases as a result of higher heat transfer to the HTF. According to Eq. (30), the electrical exergy of the system increases due to a decrease in the PV panel temperature. Over this range of velocity, the thermal and electrical efficiencies in the case with a twisted tape drop by 44.36% and increase by 6.2%, respectively. For the same case without a twisted tube, the thermal and electrical efficiencies decrease by 52.4% and increase by 4.2%, respectively.
Figure (c) shows the overall energy and exergy efficiencies of the PV-T system. Based on Eqs. (23) and (27), higher energy and exergy efficiencies are obtained for the PV system equipped with a twisted tape than for the base case. The increase in the inlet velocity from 0.05 to 0.25 m/s is associated with an increase in the energy efficiency by 20.3% and a decrease in the exergy efficiency by 5.7% for the system with a twisted tape. In contrast, for the straight tube case, the energetic and exergetic efficiencies increased by 14.1% and reduced by 8.6%, respectively.
5.3. Different types of configurations
Figure shows the temperature distribution of the PV panel for different geometries at an inlet velocity of 0.1 m/s for the mean solar radiation in October. As seen in this figure, the straight tube suffers from effective cooling in the corners of the PV panel, which reduces the system performance. This can be resolved by employing wavy tubes with twisted tape. It is evident that using the wavy tube – with 200 mm wavelength and 50 mm amplitude – and twisted tape (case 6) results in a better temperature distribution than other cases due to higher heat transfer characteristics and lower stagnation regions in the fluid flow.
Figure 15. Temperature distribution in PV-T system for different types of configurations at the inlet velocity of 0.1 m/s.

Figure (a and b) show the overall energy efficiency for different geometries. As seen in this figure, the energy efficiency for cases 2–6 increases by 5.1%, 7.8%, 6.2%, 11.5%, and 12.4% compared to the base case (case 1), respectively. The exergy efficiency in wavy-shaped tubes is higher than that of the straight tube because of the more significant heat transfer area and, consequently, more reduction in the temperature of the PV panel. Using a twisted tape in the tube also increases the exergy efficiency compared to the base case. The exergy efficiencies of cases 2–6 increased by 6.9%, 7.4%, 6.3%, 15.8%, and 16.8% compared to the base case (case 1). This is attributed to the amounts of average PV temperature and total heat absorption by the HTF for these geometries, listed in Table for different fluid velocities. As seen in this table, the panel temperature decreases as the inlet velocity increases, which is more noticeable at lower velocities. Moreover, the panel temperature in cases 2–6 is 3.3, 5.2, 4.1, 7.8, and 8.3 K, cooler than the base case at the inlet velocity of 0.1 m/s.
Figure 16. Effect of PV system configuration on the (a) energy efficiency and (b) exergy efficiency at the inlet velocity of 0.1 m/s.

Table 9. The average panel temperature and heat absorbed by HTF for the PV-T system with different cases for different velocity inlets.
Figure (a) shows the thermal and electrical energy efficiencies at different velocities. As the velocity increases, the thermal and electrical efficiencies increase. By changing the inlet velocity from 0.05 to 0.25
for cases 1–6, the thermal efficiency increases by 20%, 18.3%, 15.5%, 22.5%, 11.5%, and 10%, and the electrical efficiency increases by 4.1%, 5%. 4.5%, 6.3%,3.4% and 3.3%, respectively. Figure (b) illustrates the thermal and electrical exergy efficiencies for different geometries at different velocities. As the efficiency increases, the thermal exergy efficiency decreases, and the electrical exergy increases. In cases 2, 3, 4, 5, and 6 at the inlet velocity of 0.05 m/s, the thermal exergy efficiency increases by 5.1%, 25.3%, 6.3%, 26.2%, and 30.5% compared to the base case. The electrical efficiency increases by 8.8%, 2.3%, 9.2%, 3.9%, and 4.4% for cases 2–5 compared with the base case. In case 6, with increasing the inlet velocity, the thermal exergy efficiency decreases from 3.3% to 1.4%, and the electrical exergy increases from 9% to 9.3%. Figure (c) shows the overall energetic and exergetic efficiencies for different geometries at different velocities – higher velocity results in higher energy and lower exergy efficiency. Case 6 has the best performance from energy and exergy, in which a twisted tape is inserted inside a wavy tube with a wavelength of 200 mm and an amplitude of 50 mm. The overall energy efficiency for cases 2, 3, 4, 5, and 6 increases by 2.6%, 7.1%, 2. 9%, 12.8%, and 14.8% compared to the base case, while these numbers are 1.8%, 7.4%, 2%, 9% and 10.3% for exergy efficiency at the inlet velocity of 0.5 m/s. Increasing the inlet velocity from 0.05 m/s to 0.25 m/s increases the energy efficiency from 76.1% to 83.13% and decreases the exergy efficiency from 12.3% to 10.7%. Figure (d) shows the amount of thermal and electrical exergy. The results show that the amount of thermal exergy decreases for a higher velocity while the electrical exergy increases. In the best case (case 6), the thermal exergy decreases from 11.2 W to 4.7 W with increasing velocity, and the electrical exergy increases from 30.6 W to 31.6 W.
5.4. Different types of coolant fluid
To study the effect of fluid type on the performance of the PV-T system, eight different fluids have been used, including Ag-water nanofluid with 1%, 3%, and 5% volume fractions, n-octedane microcapsules with 5%, 10%, and 15% volume concentrations, and MPCM Nano slurry with 5% nanoparticles and 15% microcapsule. It should be noted that there is a limit for the volumetric concentration of MPCM because of the non-Newtonian behaviour of MPCM at high concentrations of MPCM; fluid behaviour becomes non-Newtonian – which dictates the upper limit of pumping. As such, the maximum concentration of 15% is considered for the MPCM.
Figure (a) presents the energy efficiency of a PV-T system at an inlet velocity of 0.1 m/s for case 5, considering the mean solar radiation in October. The addition of nanoparticles increases the overall heat transfer coefficient and the performance of a PV-T system. Using microencapsulation also increases the fluid heat capacity, and, as a result, the fluid absorbs more heat. The highest thermal and electrical energy efficiencies are found in the case of water with 15% slurry due to more decrease in the temperature of an absorber plate. Figure (b) shows the exergy efficiency for different cooling fluids. The thermal exergy efficiency decreases by increasing the nanofluid volume fraction due to higher viscosity and thermal conductivity – leading to higher entropy generation and lower exergy efficiency of the PV cell. The opposite trend is observed in the case of the volume concentration of MPCM. The increase in the volume concentration of MPCM is accompanied by an increase in thermal exergy efficiency due to an enhancement in heat capacity. Electrical exergy efficiency in both nanoparticle and MPCM increases with the enhancement of the volume fraction of fluids due to a reduction in PV temperature. Increasing the volume fraction of nanofluid and MPCM reduces and increases the exergy efficiency of the PV module compared to pure water. As such, the MPCM microcapsule with a 15% volume concentration performs best based on the overall exergy efficiency. Table shows the PV-T system average panel temperature, heat absorbed, and pressure drop in the tube for different fluids. According to this table, the panel temperature decreases with increasing nanofluid concentration. In the best case, using nanoparticles with a volume concentration of 5% reduces the panel temperature to 305.71 K. When using an MPCM slurry with a concentration of 15%, the panel temperature decreases by almost 0.91 K compared to the base fluid. The heat absorbed by the fluid declines by increasing the nanofluid concentration. The highest heat-absorbed rate of 49.17 W is achieved in the case of MPCM slurry with a 15% volume concentration. The maximum pressure drop obtained in different cases is 153.8 Pa.
Figure 18. Effect of coolant fluid on the (a) energy efficiency and (b) exergy efficiency at the inlet velocity of 0.1 m/s.

Table 10. Average panel temperature, heat absorbed, and pressure drop for different types of coolant fluid at the inlet velocity of 0.1 m/s.
5.5. Seasonal effects
Due to the difference in the solar radiation intensity in a typical year, the performance of a PV panel varies with the geographical location and throughout the year. Figure (a) shows the monthly energy efficiency of the PV panel at the inlet fluid velocity of 0.1 m/s. The highest and lowest thermal energy efficiency occurred in July and January. According to Eq. (10) and Table , absorber heat increases due to solar radiation intensity and ambient temperature enhancement. As a result, the thermal energy efficiency rises in July and decreases in January.
Figure 19. Monthly performance of the PV system in terms of (a) energy efficiency and (b) exergy efficiency for Shiraz city at the inlet velocity of 0.1 m/s.

In contrast to the previous case, the highest and lowest electrical energy efficiency occurred in January and July due to the ambient temperature reduction presented in Table . The highest and lowest overall energy efficiency is in July and January. Figure (b) illustrates the monthly exergy efficiency of the PV panel at the inlet fluid velocity of 0.1 m/s. According to Eq. (28), an increase in solar radiation intensity and a reduction in ambient temperature enhance the exergy efficiency. The best thermal exergy is related to October, while the lowest is related to January. The best electrical exergy is in January, and the lowest is in October. The highest and lowest overall exergy efficiency is in October and January. The proposed system has the best and worst performance in October and January, respectively.
Table shows the monthly average panel temperature and heat absorbed by the fluid. The highest panel temperature is in October (i.e. 306.53 K), and the lowest is in January (i.e. 302.08 K). The highest and lowest heat absorption occurs in October and January – equivalent to 255.26 and 108.25 W, respectively.
Table 11. Monthly average PV temperature and the total heat absorption by HTF for the PV-T system located in Shiraz at the inlet fluid velocity of 0.1 m/s.
5.6. Comparison against the existing data
This section compares the results obtained from the present study with those found in the literature. Table shows the energy and exergy efficiency improvement based on the proposed method in the literature and present work relative to the base case with no design improvement. As seen in this table, the geometrical configuration of the PV-T module plays a crucial role in the energy and exergy performance of the system. This study indicated that changing the geometry of the tubes from a straight to a wavy shape with a twisted tape inserted under the PV panel resulted in significant improvements in energy and exergy efficiencies, corresponding to 12.4% and 16.8% increase compared to the base case, respectively. The results showed that increasing the volume concentration of nanoparticles in the coolant fluid led to an increase in energy efficiency and a decrease in exergy efficiency. Similarly, increasing the volume concentration of the MPCM coolant fluid also led to an increase in energy and exergy efficiencies. MPCM slurry with a 15% volume concentration exhibited the best energy and exergy efficiencies among the coolant fluids considered. Compared to other papers published in the literature, the co-implementation of a wavy tube and twisted tape provides a more uniform temperature distribution along the tube wall due to the secondary flow induced in the core and near the wall regions. These dual mixing effects prohibit the formation of hot zones and boundary layers, leading to better cooling performance. This is the main reason for higher energy and exergy efficiencies obtained from this work relative to other papers published in the literature.
Table 12. comparison between the current study and other methods in terms of energy and exergy efficiency.
6. Conclusion
Maintaining the temperature of a photovoltaic (PV) system in the range of 35°C to 45°C requires an effective cooling system in place. The present study aimed to improve the cooling performance of a photovoltaic-thermal (PV-T) system by employing twisted tape inserts in wavy tubes. Having the model validated first, a parametric study was subsequently conducted using CFD to obtain the effect of various parameters, including the inlet velocity, geometry design, different types of coolant fluid, and monthly/seasonal effects on the energy/exergy efficiencies.
It is found that increasing the cooling fluid velocity from 0.05 m/s to 0.25 m/s increased the energy efficiency and decreased the exergy efficiency of the PV-T system, suggesting that there is an optimal velocity to achieve the best balance between energy and exergy efficiencies. The implementation of the wavy tube with twisted tape showed to increase the energy and exergy efficiencies by 12.4% and 16.8%, respectively, due to the dual mixing effects. These, in turn, prohibited the formation of hot zones and boundary layer, leading to enhanced performance. Increasing the volume concentration of the microencapsulated phase change material (MPCM) coolant fluid led to an increase in energy efficiency from 79.4% to 80.3% and an increase in exergy efficiency from 11.3% to 11.6%. Among the coolant fluids considered, MPCM slurry with 15% volume concentration exhibited the best energy and exergy efficiencies. The best monthly performance of the PV panel (assumed to be installed in Shiraz, Iran) in terms of energy and exergy efficiencies was obtained in July and October, corresponding to 90% and 11.3%, respectively.
Based on the conclusions drawn from this study, some suggestions for future work include (i) investigating the interaction between several passive and active cooling to improve the PV-T performance, (ii) experimental testing of the proposed configuration, (iii) optimising the design parameters to further increase energy and exergy efficiencies, (iv) techno-economic assessment of the proposed technology, (v) examining the lifetime and stability of the PV-T system under harsh environment, and (vi) investigating the scalability of the proposed cooling system for large-scale PV-T systems.
Disclosure statement
No potential conflict of interest was reported by the author(s).
Additional information
Funding
References
- Abdallah, S. R., Saidani-Scott, H., & Abdellatif, O. E. (2019). Performance analysis for hybrid PV/T system using low concentration MWCNT (water-based) nanofluid. Solar Energy, 181, 108–115. https://doi.org/10.1016/j.solener.2019.01.088
- Ahmadinejad, M., & Moosavi R. (2023). Energy and exergy evaluation of a baffled-nanofluid-based photovoltaic thermal system (PVT). International Journal of Heat and Mass Transfer, 203, 123775. https://doi.org/10.1016/j.ijheatmasstransfer.2022.123775
- Akbar, A., Najafi, G., Gorjian, S., Kasaeian, A., & Mazlan, M. (2021). Performance enhancement of a hybrid photovoltaic-thermal-thermoelectric (PVT-TE) module using nanofluid-based cooling: Indoor experimental tests and multi-objective optimization. Sustainable Energy Technologies and Assessments, 46, 101276. https://doi.org/10.1016/j.seta.2021.101276
- Alktranee, M., Shehab, M. A., Németh, Z., Bencs, P., Hernadi, K., & Koós, T. (2022). Energy and exergy assessment of photovoltaic-thermal system using tungsten trioxide nanofluid: An experimental study. International Journal of Thermofluids, 16, 100228. https://doi.org/10.1016/j.ijft.2022.100228
- Alquaity, A. B. S., Al-Dini, S. A., Wang, E. N., & Yilbas, B. S. (2012). Numerical investigation of liquid flow with phase change nanoparticles in microchannels. International Journal of Heat and Fluid Flow, 38, 159–167. https://doi.org/10.1016/j.ijheatfluidflow.2012.10.001
- Arasteh, H., Rahbari, A., Mashayekhi, R., Keshmiri, A., Mahani, R. B., & Talebizadehsardari, P. (2021). Effect of pitch distance of rotational twisted tape on the heat transfer and fluid flow characteristics. International Journal of Thermal Sciences, 170, 106966. https://doi.org/10.1016/j.ijthermalsci.2021.106966
- Aridi, R., Ali, S., Lemenand, T., Faraj, J., & Khaled, M. (2022). CFD analysis on the spatial effect of vortex generators in concentric tube heat exchangers – A comparative study. International Journal of Thermofluids, 16, 100247. https://doi.org/10.1016/j.ijft.2022.100247
- Bhattarai, S., Oh, J.-H., Euh, S.-H., Kafle, G. K., & Kim, D. H. (2012). Simulation and model validation of sheet and tube type photovoltaic thermal solar system and conventional solar collecting system in transient states. Solar Energy Materials and Solar Cells, 103, 184–193. https://doi.org/10.1016/j.solmat.2012.04.017
- Carpio, J., & Valencia, A. (2021). Heat transfer enhancement through longitudinal vortex generators in compact heat exchangers with flat tubes. International Communications in Heat and Mass Transfer, 120, 105035. https://doi.org/10.1016/j.icheatmasstransfer.2020.105035
- Chen, B., Wang, X., Zeng, R., Zhang, Y., Wang, X., Niu, J., Li, Y., & Di, H. (2008). An experimental study of convective heat transfer with microencapsulated phase change material suspension: Laminar flow in a circular tube under constant heat flux. Experimental Thermal and Fluid Science, 32(8), 1638–1646. https://doi.org/10.1016/j.expthermflusci.2008.05.008
- Collares-Pereira, M., & Rabl, A. (1979). The average distribution of solar radiation-correlations between diffuse and hemispherical and between daily and hourly insolation values. Solar Energy, 22(2), 155–164. https://doi.org/10.1016/0038-092X(79)90100-2
- Cooper, P. I. (1969). The absorption of radiation in solar stills. Solar Energy, 12(3), 333–346. https://doi.org/10.1016/0038-092X(69)90047-4
- Darbari, B., Rashidi, S., & Keshmiri, A. (2020). Nanofluid heat transfer and entropy generation inside a triangular duct equipped with delta winglet vortex generators. Journal of Thermal Analysis and Calorimetry, 140(3), 1045–1055. https://doi.org/10.1007/s10973-019-08382-7
- Demir, H., Dalkilic, A. S., Kürekci, N. A., Duangthongsuk, W., & Wongwises, S. (2011). Numerical investigation on the single phase forced convection heat transfer characteristics of TiO2 nanofluids in a double-tube counter flow heat exchanger. International Communications in Heat and Mass Transfer, 38(2), 218–228. https://doi.org/10.1016/j.icheatmasstransfer.2010.12.009
- Demirel, Y. (2013). Thermodynamic analysis. Arabian Journal for Science and Engineering, 38(2), 221–249. https://doi.org/10.1007/s13369-012-0450-8
- Du, Y., Fell, C. J., Duck, B., Chen, D., Liffman, K., Zhang, Y., Gu, M., & Zhu, Y. (2016). Evaluation of photovoltaic panel temperature in realistic scenarios. Energy Conversion and Management, 108, 60–67. https://doi.org/10.1016/j.enconman.2015.10.065
- Duffie, J. A., Beckman, W. A., & Blair, N. (2020). Solar engineering of thermal processes, photovoltaics and wind. John Wiley & Sons.
- Eisapour, A. H., Eisapour, M., Hosseini, M. J., Shafaghat, A. H., Sardari, P. T., & Ranjbar, A. A. (2021). Toward a highly efficient photovoltaic thermal module: Energy and exergy analysis. Renewable Energy, 169, 1351–1372. https://doi.org/10.1016/j.renene.2021.01.110
- Eisapour, M., Eisapour, A. H., Hosseini, M. J., & Talebizadehsardari, P. (2020). Exergy and energy analysis of wavy tubes photovoltaic-thermal systems using microencapsulated PCM nano-slurry coolant fluid. Applied Energy, 266, 114849. https://doi.org/10.1016/j.apenergy.2020.114849
- Ekramian, E., Etemad, S. G., & Haghshenasfard, M. (2014). Numerical analysis of heat transfer performance of flat plate solar collectors. Journal of Fluid Flow, Heat and Mass Transfer (JFFHMT), 1, 38–42. https://doi.org/10.11159/jffhmt.2014.006
- Essam, Y., Ali Najah, A., Ramli, R., Chau, K.-W., Idris Ibrahim, M. S., Sherif, M., Sefelnasr, A., & El-Shafie, A. (2022). Investigating photovoltaic solar power output forecasting using machine learning algorithms. Engineering Applications of Computational Fluid Mechanics, 16(1), 2002–2034. https://doi.org/10.1080/19942060.2022.2126528
- Fontenault, B. J., & Gutierrez-Miravete, E. (2012). Modeling a combined photovoltaic-thermal solar panel. Proceedings 2012 COMSOL Conference, Boston.
- Fu, Z., Yongwei, L., Liang, X., Lou, S., Qiu, Z., Cheng, Z., & Zhu, Q. (2021). Experimental investigation on the enhanced performance of a solar PVT system using micro-encapsulated PCMs. Energy, 228, 120509. https://doi.org/10.1016/j.energy.2021.120509
- Ghalambaz, M., Doostani, A., Izadpanahi, E., & Chamkha, A. J. (2020a). Conjugate natural convection flow of Ag–MgO/water hybrid nanofluid in a square cavity. Journal of Thermal Analysis and Calorimetry, 139(3), 2321–2336. https://doi.org/10.1007/s10973-019-08617-7
- Ghalambaz, M., Groşan, T., & Pop, I. (2019). Mixed convection boundary layer flow and heat transfer over a vertical plate embedded in a porous medium filled with a suspension of nano-encapsulated phase change materials. Journal of Molecular Liquids, 293, 111432. https://doi.org/10.1016/j.molliq.2019.111432
- Ghalambaz, M., Mashayekhi, R., Arasteh, H., Ali, H. M., Talebizadehsardari, P., & Yaïci, W. (2020b). Thermo-hydraulic performance analysis on the effects of truncated twisted tape inserts in a tube heat exchanger. Symmetry, 12(10), 1652. https://doi.org/10.3390/sym12101652
- Hasan, M. I. (2011). Numerical investigation of counter flow microchannel heat exchanger with MEPCM suspension. Applied Thermal Engineering, 31(6–7), 1068–1075. https://doi.org/10.1016/j.applthermaleng.2010.11.032
- Ho, C. J., Liu, Y.-C., Ghalambaz, M., & Yan, W.-M. (2020). Forced convection heat transfer of nano-encapsulated phase change material (NEPCM) suspension in a mini-channel heatsink. International Journal of Heat and Mass Transfer, 155, 119858. https://doi.org/10.1016/j.ijheatmasstransfer.2020.119858
- Hossain, M. S., Pandey, A. K., Selvaraj, J., Rahim, N. A., Rivai, A., & Tyagi, V. V. (2019). Thermal performance analysis of parallel serpentine flow based photovoltaic/thermal (PV/T) system under composite climate of Malaysia. Applied Thermal Engineering, 153, 861–871. https://doi.org/10.1016/j.applthermaleng.2019.01.007
- Huang, G., Riera Curt, S., Wang, K., & Markides, C. N. (2020). Challenges and opportunities for nanomaterials in spectral splitting for high-performance hybrid solar photovoltaic-thermal applications: A review. Nano Materials Science, 2(3), 183–203. https://doi.org/10.1016/j.nanoms.2020.03.008
- Ji, J., Lu, J.-P., Chow, T.-T., He, W., & Pei, G. (2007). A sensitivity study of a hybrid photovoltaic/thermal water-heating system with natural circulation. Applied Energy, 84(2), 222–237. https://doi.org/10.1016/j.apenergy.2006.04.009
- Kakaç, S., & Pramuanjaroenkij, A. (2009). Review of convective heat transfer enhancement with nanofluids. International Journal of Heat and Mass Transfer, 52(13-14), 3187–3196. https://doi.org/10.1016/j.ijheatmasstransfer.2009.02.006
- Keshmiri, A., Cotton, M. A. M. A., Addad, Y., Rolfo, S., & Billard, F. (2008). RANS and LES investigations of vertical flows in the fuel passages of gas-cooled nuclear reactors. Proceedings 16th ASME International Conference on Nuclear Engineering ‘ICONE16’, Pap ICONE16-48372, pp. 297–306.
- Keshmiri, A., Revell, A., & Darabkhani, H. G. (2016). Assessment of a common nonlinear eddy-viscosity turbulence model in capturing laminarization in mixed convection flows. Numerical Heat Transfer, Part A: Applications, 69(2), 146–165. https://doi.org/10.1080/10407782.2015.1069672
- Keshmiri, A., Uribe, J., & Shokri, N. (2015). Benchmarking of three different CFD codes in simulating natural, forced and mixed convection flows. Numerical Heat Transfer, Part A: Applications, 67(12). https://doi.org/10.1080/10407782.2014.965115
- Khanjari, Y., Pourfayaz, F., & Kasaeian, A. B. (2016). Numerical investigation on using of nanofluid in a water-cooled photovoltaic thermal system. Energy Conversion and Management, 122, 263–278. https://doi.org/10.1016/j.enconman.2016.05.083
- Kim, W. H., & Tae, S. P. (2022). Effect of multihole baffle-induced lobe flow structures on a high efficiency micro-thermophotovoltaic system. Engineering Applications of Computational Fluid Mechanics, 16(1), 2074–2099. https://doi.org/10.1080/19942060.2022.2130990
- Kumar, S., & Mullick, S. C. (2010). Wind heat transfer coefficient in solar collectors in outdoor conditions. Solar Energy, 84(6), 956–963. https://doi.org/10.1016/j.solener.2010.03.003
- Li, J., Zhang, W., Lingzhi, X., Zihao, L., Xin, W., Oufan, Z., Jianmei, Z., & Xiding, Z. (2022). A hybrid photovoltaic and water/air based thermal (PVT) solar energy collector with integrated PCM for building application. Renewable Energy, 199, 662–671. https://doi.org/10.1016/j.renene.2022.09.015
- Liaw, K. L., Kurnia, J. C., & Sasmito, A. P. (2021). Turbulent convective heat transfer in helical tube with twisted tape insert. International Journal of Heat and Mass Transfer, 169, 120918. https://doi.org/10.1016/j.ijheatmasstransfer.2021.120918
- Liu, B. Y. H., & Jordan, R. C. (1963). The long-term average performance of flat-plate solar-energy collectors: With design data for the US, its outlying possessions and Canada. Solar Energy, 7(2), 53–74. https://doi.org/10.1016/0038-092X(63)90006-9
- Mashayekhi, R., Arasteh, H., Talebizadehsardari, P., Kumar, A., Hangi, M., & Rahbari, A. (2021). Heat transfer enhancement of nanofluid flow in a tube equipped with rotating twisted tape inserts: A two-phase approach. Heat Transfer Engineering, 1–18. https://doi.org/10.1080/01457632.2021.1896835
- Mashayekhi, R., Arasteh, H., Toghraie, D., Motaharpour, S. H., Keshmiri, A., & Afrand, M. (2020). Heat transfer enhancement of Water-Al2O3 nanofluid in an oval channel equipped with two rows of twisted conical strip inserts in various directions: A two-phase approach. Computers & Mathematics with Applications, 79(8), 2203–2215. https://doi.org/10.1016/j.camwa.2019.10.024
- Mashayekhi, R., Eisapour, A. H., Eisapour, M., Talebizadehsardari, P., & Rahbari, A. (2022). Hydrothermal performance of twisted elliptical tube equipped with twisted tape insert. International Journal of Thermal Sciences, 172, 107233. https://doi.org/10.1016/j.ijthermalsci.2021.107233
- Mehryan, S. A. M., Ghalambaz, M., Chamkha, A. J., & Izadi, M. (2020). Numerical study on natural convection of Ag–MgO hybrid/water nanofluid inside a porous enclosure: A local thermal non-equilibrium model. Powder Technology, 367, 443–455. https://doi.org/10.1016/j.powtec.2020.04.005
- Menon, G. S., Murali, S., Elias, J., Aniesrani Delfiya, D. S., Alfiya, P. V., & Samuel, M. P. (2022). Experimental investigations on unglazed photovoltaic-thermal (PVT) system using water and nanofluid cooling medium. Renewable Energy, 188, 986–996. https://doi.org/10.1016/j.renene.2022.02.080
- Mishra, P. C., Mukherjee, S., Nayak, S. K., & Panda, A. (2014). A brief review on viscosity of nanofluids. International Nano Letters, 4(4), 109–120. https://doi.org/10.1007/s40089-014-0126-3
- Moore, K., & Wei, W. (2021). Applications of carbon nanomaterials in perovskite solar cells for solar energy conversion. Nano Materials Science, 3(3), 276–290. https://doi.org/10.1016/j.nanoms.2021.03.005
- Öğüt, E. B. (2009). Natural convection of water-based nanofluids in an inclined enclosure with a heat source. International Journal of Thermal Sciences, 48(11), 2063–2073. https://doi.org/10.1016/j.ijthermalsci.2009.03.014
- Özakin, A. N., & Kaya, F. (2019). Effect on the exergy of the PVT system of fins added to an air-cooled channel: A study on temperature and air velocity with ANSYS Fluent. Solar Energy, 184, 561–569. https://doi.org/10.1016/j.solener.2019.03.100
- Saleem, A., Akhtar, S., Nadeem, S., Alharbi, F. M., Ghalambaz, M., & Issakhov, A. (2020). Mathematical computations for peristaltic flow of heated non-Newtonian fluid inside a sinusoidal elliptic duct. Physica Scripta, 95(10), 105009. https://doi.org/10.1088/1402-4896/abbaa3
- Saleem, S., Akhtar, S., Nadeem, S., Saleem, A., Ghalambaz, M., & Issakhov, A. (2021). Mathematical study of electroosmotically driven peristaltic flow of Casson fluid inside a tube having systematically contracting and relaxing sinusoidal heated walls. Chinese Journal of Physics, 71, 300–311. https://doi.org/10.1016/j.cjph.2021.02.015
- Santos, J. M., Pinazo, J. M., & Cañada, J. (2003). Methodology for generating daily clearness index index values Kt starting from the monthly average daily value K¯t. Determining the daily sequence using stochastic models. Renewable Energy, 28(10), 1523–1544. https://doi.org/10.1016/S0960-1481(02)00217-3
- Shahsavar, A., Eisapour, M., & Talebizadehsardari, P. (2020). Experimental evaluation of novel photovoltaic/thermal systems using serpentine cooling tubes with different cross-sections of circular, triangular and rectangular. Energy, 208, 118409. https://doi.org/10.1016/j.energy.2020.118409
- Tian, M.-W., Khorasani, S., Moria, H., Pourhedayat, S., & Dizaji, H. S. (2020). Profit and efficiency boost of triangular vortex-generators by novel techniques. International Journal of Heat and Mass Transfer, 156, 119842. https://doi.org/10.1016/j.ijheatmasstransfer.2020.119842
- Tomar, V., Norton, B., & Tiwari, G. N. (2019). A novel approach towards investigating the performance of different PVT configurations integrated on test cells: An experimental study. Renewable Energy, 137, 93–108. https://doi.org/10.1016/j.renene.2017.11.020
- Vand, V. (1945). Theory of viscosity of concentrated suspensions. Nature, 155(3934), 364–365. https://doi.org/10.1038/155364b0
- Wu, S.-Y., Guo, F.-H., & Xiao, L. (2015). A review on the methodology for calculating heat and exergy losses of a conventional solar PV/T system. International Journal of Green Energy, 12(4), 379–397. https://doi.org/10.1080/15435075.2013.840833
- Xuan, Y., Li, Q., & Hu, W. (2003). Aggregation structure and thermal conductivity of nanofluids. AIChE Journal, 49(4), 1038–1043. https://doi.org/10.1002/aic.690490420
- Yaghoubi, M. A., & Sabzevari, A. (1996). Further data on solar radiation in Shiraz, Iran. Renewable Energy, 7(4), 393–399. https://doi.org/10.1016/0960-1481(96)00010-9
- Yu, Q., Romagnoli, A., Yang, R., Xie, D., Liu, C., Ding, Y., & Li, Y. (2019). Numerical study on energy and exergy performances of a microencapsulated phase change material slurry based photovoltaic/thermal module. Energy Conversion and Management, 183, 708–720. https://doi.org/10.1016/j.enconman.2019.01.029
Appendix A
Solar radiation intensity
The isotropic diffuse model proposed by Liu and Jordan (B. Y. H. Liu & Jordan, Citation1963) was used to obtain the solar radiation intensity. In this method, solar radiation consists of direct, isotropic diffuse, and solar radiation diffusely reflected from the ground. The total solar radiation on the tilted plate for an hour is obtained from the following equation:
(32)
(32)
is ground reflected which is considered 0.2 for all of the months in this study.
is the slop of the collector and in this study considered
.
is the ratio of beam radiation calculated by (Duffie et al., Citation2020):
(33)
(33) where
(34)
(34)
(35)
(35)
is the latitude which is 29.59 for Shiraz city.
is the hour angle.
is the surface azimuth angle and set zero due south in this study.
is declination and calculation by (Cooper, Citation1969):
(36)
(36) which n is the day of the year. The ratio of hourly total to total daily radiation is calculated by (Collares-Pereira & Rabl, Citation1979):
(37)
(37) The coefficients a and b are given by (Duffie et al., Citation2020):
(38)
(38)
(39)
(39)
is sunset hour angle is given by (Duffie et al., Citation2020):
(40)
(40) To obtain the daily clearance index, KT is defined as follows (Santos et al., Citation2003):
(41)
(41) where
, is the day of the month,
is the number of days of the month and
is a dimensionless parameter given by:
(42)
(42)
is a dimensionless parameter given by:
(43)
(43) The value of
and
defined as follow:
(44)
(44)
(45)
(45) The solar irradiation and monthly means of the daily clear index for different months in Shiraz city of Iran are presented in Table .
Table A1. Irradiation and monthly means of the daily clear index for different months in Shiraz city (Yaghoubi & Sabzevari, Citation1996).
For
(46)
(46) And for
(47)
(47) The hourly diffuse radiation
is obtained with the ratio of hourly diffuse to daily diffuse radiation
as (Duffie et al., Citation2020):
(48)
(48) Hourly beam radiation
is calculated by subtracting
from I. Finally, the solar intensity for the average day for all of the months for the city of Shiraz is presented in . Then the moderate solar radiation intensity from 10 am to 2 pm is calculated and shown in Table .
Figure A1. Solar radiation intensity for different months of Shiraz, Iran.
Ambient temperature
To obtain the average ambient temperature for different months, the minimum temperature and maximum temperature for days of the month based on weather data for 2020 in Shiraz are given in . Then, after passing the curve of the lowest temperature and the highest temperature of the two curves obtained in each graph, the average of the lowest temperature and the highest temperature for the days are averaged, and the results are presented in Table .
Figure A2. Average low and high-temperature distribution for Shiraz city according to AccuWeather report for the year 2020.