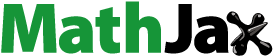
Abstract
A numerical model incorporating the phase field method, the arbitrary Lagrangian–Eulerian (ALE) method, the effective heat capacity model and the Navier–Stokes equations is established to solve the complex force–mass–heat coupling transport during the process of a particle impacting a high-temperature melt pool. Then, a study of the particle–melt pool interaction mechanism is carried out, the dominant factors that influence the particle capture behaviour explored, and the effects of contact angle and particle velocity on particle melting further analysed. Results show that a decaying particle oscillation phenomenon and a periodical surface meniscus structure appear during the process of a particle impacting a melt pool. As for particle melting, it is far delayed behind particle oscillation and mainly takes place at the static heat transfer stage; its heat accumulation is dominated by the particle’s mushy degree, and becomes gentle after the particle becomes totally mushy. However, the particle’s melting speed is determined by surface heating; the dominant melting factor converts to laser irradiation with increasing laser energy density; it changes to melt pool heating as the particle velocity increases and the contact angle decreases. Furthermore, when the contact angle decreases, the optimized initial velocity region broadens, and the best initial velocity gradually reduces. Highlights of the article are as follows: a single particle impacting a high-temperature melt pool model incorporating the coupling of the phase field and ALE methods is developed. Further, a dominant particle melting comparison map under various laser parameters is made. Dominant particle melting converts to melt pool heating as the initial velocity increases and the equilibrium contact angle decreases. The optimization of the initial particle velocity region broadens and the best initial velocity reduces to zero as the equilibrium contact angle decreases.
1. Introduction
The powder particle deposition process is an important feature of direct laser deposition (DLD) additive manufacturing technology, which is also a basic guarantee for realizing the rapid manufacturing of functionally graded materials and complex structures. During the manufacturing procedure, thousands of micro-particles fly into the millimetre melt pool (formed as the substrate is melted by a high heat flux laser), and are captured and melted in order to establish the layer (Thompson et al., Citation2015). In most studies, such as by Qi et al. (Citation2006) and Wen and Shin (Citation2011), particles are considered to be captured directly after falling into the melt pool. However, the experiments carried out by Haley et al. (Citation2018) and Volpp et al. (Citation2018) showed that the particles appeared to exhibit three kinds of behaviour as they contacted and interacted with the surface of the melt pool, i.e. rebounding, oscillation and submergence. Those different behaviours make the capture process change sharply: rebounding particles would not be captured; submerged particles would be captured directly; and oscillating particles would gradually melt onto the melt pool surface. A totally melted particle could be well-captured eventually, but a partially melted particle would finally stay on the surface and solidify as a defect, making a rough and low precision layer surface. Obviously, the interaction between deposited particles and the melt pool directly determines the particle capture efficiency and surface roughness, which has an important impact on moulding quality, speed and manufacturing stability. Knowing how to control the manufacturing process in order to encourage more particles onto the melt pool surface and to show submerged or fully melted behaviour (oscillating particles) would be significantly helpful for improving the DLD process. Therefore, clarifying the interaction mechanism between particles and the melt pool and understanding the dominant factors that influence particle motion behaviour are the primary priorities.
The study of solid–fluid interaction can be traced back to the nineteenth century, when as early as Citation1897 Worthington and Cole studied the cavitation phenomenon caused by the impact of a particle ball on a liquid surface through an auxiliary camera. In the following century, a large number of solid particles impacting a liquid surface were continuously studied. Viswanathan (Citation1999) found that, for particles above the millimetre level, their behaviour was mainly affected by surface tension, particle inertia and the drag force, while motion on the surface presented submergence, oscillation and rebound. There are many factors that affect the impact of particles on a liquid surface. Vella et al. (Citation2006) studied the influence of different wetting angles on a static floating cylinder and a sphere on a water surface, respectively. Their results showed that a change of wetting angle had no effect on the equilibrium of a small radius cylinder, but had great influence on the ball. Lee and Kim (Citation2011) combined potential flow theory and the Young–Laplace equation to study the moving state of a hydrophobic spherical particle in the initial stage of submergence, and the radius and velocity ranges for different impact behaviours were preliminarily predicted using the Weber (We) and Bond (Bo) numbers. The impact behaviour and influence mechanism of submicron particles are slightly different. Kintea et al. (Citation2016) numerically studied the impact behaviour of rotating and non-rotating micro-particles on a water surface under different attack angles and We numbers. It was found that the wetting angle plays an important role in cavitation formation, especially for smaller We numbers and particle radii, and the attack angle has almost no effect. Wang et al. (Citation2015) introduced a dynamic wetting angle into the numerical model during their research on the impact behaviour of submicron particles, and the results were more consistent with the experimental results. Ji et al. (Citation2017) further improved the model based on that of Wang et al. to analyse the energy conversion mechanism of the particle impact process, and determined the quantitative interaction of hydrodynamic force and surface tension in particle impact. All of the above enable us to understand the particle motion and capture characteristics clearly during the particle−fluid interaction; moreover, the basic force transport process is also carefully analysed. Their studies are widely used in guiding industrial production, such as water spraying in micro-particle pollutants removing, wet scrubbing for industrial particles, and even biomimetic floating organisms. However, these results are not well suited to the process of particle capture by a melt pool, since the high-temperature difference influence and particle melting were not considered.
In fact, the particle melting can change the particle capture process remarkably on a liquid surface; additionally, when the temperature gradient is high, the surface tension of a liquid metal will change greatly, which affects the behaviour of particles as well. Haley et al. (Citation2018) investigated the impact behaviour experimentally of particles on the surface of a high-temperature melt pool, and demonstrated that the particles landing district directly affects the duration time of particles on the melt pool surface, which has the largest effect on its behaviour; in contrast, the effect of inertial kinetic energy is slight. Prasad et al. (Citation2019) and Volpp et al. (Citation2018) also observed those phenomena in experiments. In addition, it was revealed that the particles tend to move towards the melt pool centre, which greatly reduces the melting time of the particles. In order to explore the particle behaviour formation mechanism in the above further, they also conducted numerical and analytical studies. Haley et al. (Citation2019) developed a numerical model of a single particle impacting the surface of a melt pool based on a three-phase flow model, and explored the changing trend of particle melting time under different wetting angles, impact velocities and particle sizes. In addition, the effects of heat transfer and laser irradiation of the melting of particles were also discussed. Volpp (Citation2019) established a simplified analytical model of particle melting and motion based on the surface tension model and the Young equation, and revealed that the wetting effect is the main driving force of particle motion; additionally, they showed that the temperature difference between the particles and the melt pool has a significant effect on the particles’ behaviour—too large a temperature difference tends to induce particle rebound. An investigation of the effect of temperature based surface tension on particle motion during the particle capture process has also been carried out by Lin (Citation1999) based on the elastic impact theory model. They found that the higher the temperature and the larger the radius of the particle, the more easily the particles rebound from the melt pool surface—results very similar to those of Volpp (Citation2019). Gatzen et al. (Citation2014) found that the floating of particles on the melt pool surface is also affected by liquid viscosity—the larger is the viscosity, the weaker is particles’ moving ability. Dong et al. (Citation2022) numerically investigated the melting process of a particle completely immersed in a melt pool, focusing on the influence of the wetting effect and verifying the effectiveness of the effective heat capacity method in tracking latent enthalpy release/absorption. Gadeschi et al. (Citation2021) also studied the motion and heat transfer process of particles completely immersed in fluid, but the temperature range was small in their research and no phase change occurred. The above research can provide better methods and ideas for subsequent study, but there are still some problems.
The process of a particle impacting a melt pool and being heated, melted and captured is a complex physical problem involving force–mass–heat coupling transfer, solid–liquid phase change, gas–liquid free interface tracking, and fluid–solid interaction (FSI). However, in analytical studies, the particle’s motion and the development of gas–liquid interface morphology are obtained through idealized formulae combined with plane geometry principles, which cannot take into account the real evolution process during the FSI process. For numerical studies, either the model does not involve the gas–liquid free interface and only the phase change problem of a completely immersed particle is studied, or the solid phase is simplified as a third viscous fluid, which cannot clearly describe the force–mass–heat transfer process that occurs on the moving boundary of the FSI process. Obviously, an innovative particle–melt pool interplay model needs to be developed that not only can track the melt pool free surface deformation and describe the FSI process, but also can take into account the solid–liquid phase change introduced by particle melting. To address the above issues, a phase field (PF) method incorporated with the arbitrary Lagrangian–Eulerian (ALE) model is first introduced. As is well known, the PF method based on the fourth-order Cahn–Hilliard equation has excellent conservation and efficiency in tracking the free interface under complex multi-physical fields. For example Mondal et al. have applied the PF method to study electric-field-driven (Mondal et al., Citation2013), pulsating-flow-driven (Mondal et al., Citation2014) and rheology-modulated (Mondal et al., Citation2015) two-phase flow in a micro-channel, respectively, and the free surface behaviour was well captured. Additionally, the PF method also obtained appreciable results when used in studies of magnetic-field-driven two-phase flow (Gorthi et al., Citation2017), thermo-capillary filling dynamics (DasGupta et al., Citation2014) and droplet breakup under a non-uniform force field (Shyam et al., Citation2022). The ALE method based on moving mesh technology is widely used in dealing with FSI problems, especially for solving large domain deformations, and shows great applicability, such as swing tracking of a fixed elastic rod under fluid action (Jacob et al., Citation2021) and high-velocity impact welding (Nassiri & Kinsey, Citation2016). Zhang et al. (Citation2020) introduced this method in the study of particles and gas–liquid interface interaction as well, and their results showed that their model had good accuracy and robustness. Obviously, the combination of the PF and ALE methods can directly describe the gas–liquid–solid coupling problem involved in the process of a particle impacting a melt pool, and the clear existence of solid boundaries makes it easier to handle the force and heat transfer problems during the FSI. Moreover, the introduction of moving mesh technology can also reduce the computational divergence caused by the continuous mesh deterioration induced by the particle movement. In addition, in order to simulate clearly the particle melting process generated by melt pool heating, the effective heat capacity method based on the mushy zone assumption is further applied. This method integrates the latent heat release/absorption into the apparent heat capacity (Yang & He, Citation2010), which not only accurately describes the position of the solid–liquid interface, but also greatly reduces the computational complexity of the simulation process. It is widely used in solid–liquid phase change tracking currently (Cui et al., Citation2022; Dong et al., Citation2022).
In conclusion, a numerical model incorporating the PF model, the ALE model, the effective heat capacity method and the Navier–Stokes (N-S) equations is established in this article in order to solve the complex force–mass–heat coupling transport during the process of a particle impacting a high-temperature melt pool. Based on this model, the movement and melting evolution behaviour of a single metal particle on the surface of a high-temperature melt pool are studied and the influence of temperature on particle capture is explored. In addition, the influence of the particle velocity and equilibrium wetting angle regime on particle impact is studied in order to provide a reference for optimizing the DLD manufacturing procedure. It should be noted that the equilibrium wetting angle used here concentrates on the more than 90° case, since micro metal particles show hydrophobic features and Eustatopoulos (Citation2015) showed that the contact angle is almost always more than 90° in the DLD process. Tsigaridis et al. (Citation2006) also revealed that most micron size particles are hydrophobic, especially iron and ferroalloys, and the contact angle is about 120° (Schönecker et al., Citation2015). Furthermore, as a pilot study, the velocity range investigated in this article is concentrated in the range 0∼4 m/s (low speed particle deposition), and research on particle capture under medium- and high-speed and hydrophilic conditions will be given in a follow-up study.
2. Model formulation
As particles impact the surface of a high-temperature melt pool, owing to the influence of momentum and surface wetting caused by particle impact, the liquid–gas interface deforms and intensifies gradually to form a meniscus structure; in turn, the meniscus structure acts on particles and changes the movement state of the particles; simultaneously, the particle temperature increases continuously owing to heat transfer from the melt pool, and finally the particles melt and are captured. The process of particles impacting a high-temperature melt pool is a liquid/gas two-phase flow and a fluid–solid interaction process with complex heat, mass and force transfer phenomena, which are shown in Figure (a). Figure (b) shows the geometry used in this article, where the height of the gas domain is h3, the particle diameter is dp, the particle initial velocity is v0 and at first the particle is located in the gas region. Since the particle diameter (10∼100 µm) is extremely smaller compared with the melt pool surface (about 1∼3 mm), the impact zone on the deformed surface of the melt pool (caused by the Marangoni force and the vapour recoil force − Bian, Ling, et al., Citation2022) can be approximated as a flat surface here. Additionally, the particle impact is assumed to be vertically impinging, and the distance between particle centroid and melt pool surface is h1. Furthermore, as the melt pool evolution enters a quasi steady state (the melt pool is in this state most of the time), the temperature of the melt pool remains unchanged (Bian, Tan, et al., Citation2022). In this article for simplification, only the region near the the surface of the melt pool is taken as the liquid research domain (the height is h2 and the initial temperature is T0). The detailed geometry parameters and the case arrangement used here are shown in Tables and , respectively.
Figure 1. Particle−melt pool interaction: (a) physics phenomena; (b) geometry model (for the axis-symmetric geometry, only half the cross section is built to save computing resource).

Table 1. The main model parameters.
Table 2. Cases arrangement in this study.
2.1 Basic assumptions
On the basis of accuracy in simulation, some other assumptions are made as follows to make the model simplified and tractable: (1) the fluid flow is laminar and incompressible, owing to its velocity being far smaller than the speed of sound; (2) the particle volume expansion induced by the temperature increase is ignored; (3) the particle shape is assumed to be unchanged during impact even though part of the particle is melted, since the time scale is milliseconds and the liquid part does not separate owing to inertia (the same simplification can also be found in the studies of Haley et al. (Citation2019) and Volpp (Citation2019); and (4) heat radiation from the melt pool surface is neglected. Laser irradiation of the particle is not considered, but is taken as a comparison parameter for the study of clarifying the main controlling factor in particle heating.
2.2 Mathematic model
2.2.1 Fluid flow and heat transfer
For incompressible laminar fluid flow (Re < 800 in this study), the N-S equations are shown as follows:
(1)
(1)
(2)
(2)
(3)
(3) where ρ is fluid density, u is fluid velocity, t is time, µ is fluid viscosity, p is pressure, g is gravity acceleration, h is enthalpy and λ is thermal conductivity. The PF method based Cahn−Hillard equations are applied to track gas–liquid two-phase flow (Akinyemi et al., Citation2020):
(4)
(4) where ϕ is the PF variable, the value of which is −1 or 1 (gas or liquid) except for the transition region (between −1 and 1), ψ is the auxiliary variable, ε is the interface thickness parameter (taking minimum grid length), σ is the surface tension coefficient,
is the mixing energy density,
is the mobility of the interface, and χ is the mobility adjustment parameter (maximum fluid velocity on the interface in this article).
All properties used in the equations are material mixing properties, which can be computed as follows:
(5)
(5) where η represents density ρ, viscosity µ or thermal conductivity λ, respectively. The subscripts l and g indicate liquid and gas, respectively. For Equation (2), the fourth term ( fs) and fifth term ( fw) on right-hand side are volumetric surface tension and wetting force, respectively.
(6)
(6) where ni is the vector normal at the liquid–gas–solid three-phase contact point, θw is the dynamic equilibrium contact angle, which is gradually changing during particle motion and can be computed according to the method recommended by Kistler (Citation1993).
2.2.2 Particle motion and melt
Since the particle is assumed to impact vertically on the melt pool, only the vertical particle motion has been considered. During impact, four main forces are exerted on the particle, i.e. surface tension and surface pressure imposed by the meniscus structure, fluid viscous force and gravity (Ji et al., Citation2017; Kintea et al., Citation2016), respectively. The momentum equation is shown as follows:
(7)
(7) where v is the particle velocity and mp is the particle’s mass. The first term on the right-hand side of Equation (7) is the surface tension imposed by the meniscus structure, which is expressed as
(8)
(8) where
is the unit tangential vector on the liquid–gas interface. The second and third terms on the right-hand side of Equation (7) are the surface pressure and viscous forces, respectively (hydrodynamic force).
(9)
(9) where
is the unit vector normal to the liquid–gas interface. The energy conversation equation for the particle is shown as follows:
(10)
(10) In this study, the particle experiences a phase change under heating by the melt pool. To track and consider this phenomenon, the effective heat capacity method is introduced, which is defined as follows:
(11)
(11) where L is the melting latent heat of the particle, e is half the transition temperature range of the liquid–solid phase change, Tm is the temperature point of the liquid–solid phase change, Cps and Cpl are the specific heat capacities of the solid phase and liquid phase of the particle, respectively, and h(x, y) is the second-order smooth Heaviside function. The material properties used in this study are listed in Table .
Table 3. Material properties.
2.3 Model validation
Since the experiment on a single micro-particle impacting a high-temperature melt pool is hard to perform, to validate the model established in the article, a comparison has been carried out with the results of models from the literature under the same conditions. The validation divides to two parts: the first is a validation of the hydrophobic micro-particle impact on a water surface at room temperature, the purpose of which is to test the correct incorporation of the wetting effect, two-phase flow, ALE and FSI; the second part is validating the implementation of liquid solid coupling heat transfer and phase change mass transfer, which is a solidification process of a fixed liquid droplet on a cold surface. A comparison of the results is shown as Figures (a) and (b). For the first part, two conditions, i.e. submergence and oscillation, have been chosen here, and it can be seen that the numerical result obtained by this model is almost the same as that obtained by Ji et al. (Citation2017). The maximum and average errors are 17.3% and 7.2%, respectively, under the oscillation condition, and the maximum and average errors are 10.7% and 5.4%, respectively, under the submergence condition.
Figure 2. Model validation: (a) hydrophobic micro-particle impact on water surface; (b) solidification process of a fixed liquid droplet on a cold surface.

For the second part, the dimensionless velocity of the solid–liquid interface during solidification is taken as the validation parameter (by Xiao et al., Citation2017), and from Figure (b) it can be shown that our model can track the advanced interface accurately. It should be pointed out that, owing to the fact that the model used in this article does not consider the influence of droplet condensation expansion, and as condensation progresses, this factor will cause the droplet top to rise continuously. Therefore, after a certain stage, the error between the experimental and simulation results will increase (about 12 s in this article). However, this error does not affect the reliability of the model used in this article since, even at the final time of 18 s (the solidification degree is about 70%), the maximum error between the simulation and experiment is still below 10%.
The above two problem validations combine to reveal the correct implement of those methods in our built model; therefore, this model is accurate and convincing for application in future studies on micro-particle impact on high-temperature melt pools.
3. Results and discussion
3.1 Particle thermodynamic behaviour
The first stage. After impact by a spherical particle (6.6 ms), the liquid–gas interface deforms and quickly forms a ‘meniscus structure’. As the particle contacts the liquid–gas interface, a solid–gas–liquid three-phase contact angle appears and increases rapidly from 0°, and before it reaches the dynamic equilibrium contact angle (computed from the static equilibrium contact angle, i.e. 115°), a capillary wetting force induced by the non-equilibrium contact angle is produced at the contact point. Under the capillary wetting force effect, the liquid–gas interface climbs on the solid particle surface, and a ‘convex hump and concave waist’ meniscus structure is formed first, as shown in Figure (only a half cross section is shown because of its axisymmetric geometry). The surface tension on the particle presents in the negative z-direction and increases to −0.23 mN from zero as shown in Figure , which displays the force variation trend on the particle. The particle dropping velocity is accelerated and rises to −0.65 m/s from −0.05 m/s (the origin of free dropping in air), and the particle displacement increases to −270 µm, as shown in Figure . However, at this stage the surface pressure and viscous force exerted by the meniscus structure present in the positive z-direction, and both of them increase with an increase in the particle dropping velocity.
Once the equilibrium contact angle is reached, the wetting effect disappears, climbing of the liquid–gas interface on the particle surface stops, and furthermore, under the downward particle motion, the deformation of the ‘convex hump and concave waist’ structure declines, transports to the far field and gradually vanishes (Figure (d)). The surface tension decreases, but at this moment it is still bigger than the sum of the positive surface pressure and viscous force, hence the particle’s remaining acceleration drops (the velocity continues increasing to −0.75 m/s), and only the acceleration continuously decreases to zero. After that, the sum of the positive forces is bigger than the negative surface tension and the particle begins slowing down (it decreases to −0.4 m/s); however, the velocity-dependent viscous force and surface pressure also decrease.
The second stage. Induced by the continuous particle dropping, the deformation of the liquid–gas interface changes to a ‘concave hollow and convex waist’ meniscus structure, the surface tension changes to the positive z-direction as well and increases to 0.15 mN. In this period, the particle dropping velocity decreases quickly to zero (owing to the increasing positive z-acceleration) and the particle reaches its lowest location (the displacement is −300 µm, as shown in Figure ). Subsequently, the particle begins to move upwards and its velocity gradually rises to 0.2 m/s; the liquid–gas interface rises and the deformation of the ‘concave hollow and convex waist’ meniscus structure reduces. Additionally, the surface tension also gradually decreases to zero, but the negative z surface pressure and viscous force become larger owing to the increasing positive z particle velocity.
The third stage. As the total force changes to the negative z-direction, the particle begins to decelerate and moves to a displacement of −275 µm when the velocity is zero. A new ‘convex hump and concave waist’ structure on the liquid–gas interface is again formed near the particle.
The fourth stage. In the following process, the motion of and force on the particle and liquid–gas interface periodically repeat the above stage, but the amplitude reduces greatly. The particle gradually tends to steady and becomes static after 7.2 ms.
During the process of the particle impacting the melt pool surface, the heat continuously transfers to the particle from the melt pool. In Figure , the colour of the particle represents its temperature and the black line indicates the melting line. It can be observed that, until the particle becomes static, the maximum temperature is about 1600 K and only a small part of the particle is melted. Figure further illustrates the quantitative evolution of the contact area, melt volume (>1500 K), mushy volume (>1450 K) and heat accumulation of the particle. The variation trend of the contact area is similar to that of the particle motion, and the ratios of the maximum and final contact areas to total surface area are 0.37 (6.8 ms) and 0.292 (7.2 ms), respectively. All of the melt and mushy volumes and heat accumulation increase continuously; at the static time, the ratio of melt and mushy volumes to particle volume are 0.05 and 0.012, respectively, and the heat accumulation is about 0.02 J. The mushy volume increases more quickly than the melt volume, and the particle becomes total mushy at 8.85 ms, but it does not become totally melted until 10.7 ms. The heat accumulation is mainly driven by the mushy evolution; before becoming totally mushy, it increases sharply (increase >0.17 J in about 1.6 ms), but after that it increases more and more slowly and only increases by 0.01 J in about 1.8 ms.
Figure 6 Evolution of contact area, melt volume, mushy volume and heat accumulation of the particle.

A comparison between the effect of heat transfer from the pure melt pool and laser irradiation of the particle melting has also been performed. For a uniform laser beam with radius r and power P, its effect on particle melting can be estimated as follows: the heat flow density is
If the surface absorptivity of the particle is ε and the heated area is A, the heat transfer in unit time is qs = εqA; hence, the particle melting time under pure laser irradiation is trad = Q/qs, where Q is the total energy required by particle melting and can be extracted from simulation results. A is half the sphere’s superficial area and ε is 0.35 (Haley et al. Citation2019). Figure shows the various melting times versus laser power under different particle radius conditions; it can be seen that the particle melting time increases quickly with the increase of laser spot radius and the decrease of laser power. Under some conditions, the melting time is far larger than the melt pool solidification time (5∼10 ms), which will induce defects on the layer surface when manufacturing.
Figure 7. Melting time versus laser power under different particle radius conditions with pure laser irradiation.

Clarifying the dominant control factor of particle melting is of important significance in order to improve the manufacturing process further (to change the main factor to accelerate particle melting). Here, further, a particle melting parameter comparison map under various laser parameters (P: 100∼1500 W; rlaser: 0.02∼5 mm) is made, as shown in Figure Here, tcod represents the residual time (which indicates the time from the beginning of particle motion to total particle melting here) of the particle obtained by pure melt pool heat transfer, trad represents the time obtained by pure laser irradiation. The ratio trad/tcod indicates the influence degree of melt pool heating or laser irradiation compared with each other, the bigger the value the greater the influence of melt pool heating, and vice versa. Additionally, when trad/tcod = 1, the heat transfer from the two is balanced. Under common laser working conditions (P: 100∼1500 W; r: 0.1∼1 mm), the dominant factor in particle melting gradually changes to melt pool heating from laser irradiation, with decreasing laser power and increasing diameter.
3.2 Influence of particle initial velocity on particle behaviour
An investigation on particle impact behaviour under different velocity conditions (0∼4 m/s) has been carried out, as shown in Figure , which displays the evolution of surface tension, velocity and displacement of the particle. Since the variation trend of surface pressure and viscous force is similar with velocity, they are not shown detailed here. Additionally, the times of the beginning of oscillation for different velocity conditions vary significantly; thus, a time after contact (relative time) is using to replace the true particle motion time. It can be seen that the influence of the wetting effect induced by non-equilibrium continuously decreases with increasing particle initial velocity; the ‘convex hump and concave waist’ meniscus structure of the first stage gradually becomes smaller and the initial acceleration weakens. Especially under the 4 m/s condition, there is no liquid climb on the particle surface and the ‘concave hollow and convex waist’ meniscus structure is formed on the surface directly after impact (the second stage). Additionally, both the velocity and displacement at each stage are continuously increasing (such that the maximum displacement increases to −420 µm at the second stage, and the maximum velocity increases to1.5 m/s at the third stage), and the surface deformation and surface tension become bigger as well. However, the various initial particle velocities have no effect on the final particle displacement and contact area ratio, which are all −285 µm and 0.292, respectively.
The force proportion and evolution at different stages is significantly important in controlling the particle motion behaviour; thus, a detailed relative force ratio (to surface tension) evolution study based at each stage under different velocity conditions is carried out. Since the effect of those time dependent forces on the particle cannot be compared directly, a statistical integral ratio analysis on the particle work is introduced as a replacement, where ∫(Fp·Δs)/ ∫(Fs·Δs) and ∫(Fv·Δs)/ ∫(Fs·Δs) represent the work ratio of surface pressure and viscous force to surface tension, respectively.
As shown in Figure , for the different stages under the same velocity condition, as the initial velocity is zero, the surface tension ratio first exhibits an increasing trend and then decreases with particle impact, and the viscous force ratio varies slightly. However, for other velocities, the surface pressure ratio first tends to decrease, then increases to a steady state, and the viscous force gradually decreases to a steady state; the smaller the initial velocity, the earlier the stage that the force enters a steady state. When it comes to various velocities in the same stage, with the velocity increasing, the surface pressure ratio first increases and then decreases in the first stage, continuously decreases in the second stage, and changes little in the following stages. As for the viscous force ratio, it changes obviously only in the first stage, which gradually increases (by almost three times) with the velocity increasing, and in other stages it changes only slightly. On the whole, the effect of surface pressure increases first, then decreases with increasing velocity, and the viscous force effect always increases, as shown in Figure .
The particle melting process is also significantly affected by variation of the particle initial velocity, as shown in Figure . With increasing initial velocity, the particle residual time reduces sharply (∼10 ms decreasing to ∼5 ms), and it gradually tends to be consistent with the melting time (saving the time wasted during the particle’s move to the melt pool surface). On the whole, both the residual time and the melting time of the particle tend to decrease first and then increase, and the melting time is minimum (3.2 ms) when the initial velocity is 1 m/s. In order to clarify the reason for these evolving phenomena, an investigation of the heat flux at the particle’s surface and the contact area during particle oscillation and the static process have been performed. For the various velocity conditions, the final contact area and heat boundary are almost the same, thus the melting difference is mainly shaped by the oscillation process. As shown in Figure , during the oscillation period the average contact area increases gradually as the initial velocity increases, but the amplitude becomes smaller. Additionally, the heat flux at the particle surface tends to increase at first, the maximum heat flux being obtained under the 1 m/s condition; after that, the heat flux decreases sharply, but the amplitude also becomes smaller as the velocity continues to increase. Combining those two factors, the minimum melting time is obtained when the initial velocity is 1 m/s, which has the biggest a total heat flux during oscillation period. Furthermore, as the initial velocity increases, the particle residual time decreases almost 50%, the trad/tcod contour line moving towards two, meaning an enhanced melt pool heating under common laser parameters. Thus, control of improved melt pool transfer should be considered first in order to reduce the residual and melting time of the particle when manufacturing with a higher particle initial velocity.
3.3 Influence of equilibrium contact angle on particle behaviour
Figure illustrates the evolution of surface tension and the velocity and displacement of the particle under various steady equilibrium contact angle conditions with initial velocity zero. It is obvious that the influence of the wetting effect rises in the first stage. With decreasing equilibrium contact angle, the deformation of the ‘convex hump and concave waist’ meniscus structure intensifies, but its duration reduces (∼0.11 ms at 145° decreasing to ∼0.05 ms at 90°). In this stage, the maximum dropping velocity of the particle remains increases to −1.15 m/s (from 0.4 m/s) owing to the increasing surface tension and the driven acceleration. The maximum displacement increases to −340 µm from −250 µm as well. Additionally, the variations of those phenomena gradually sharpen between conditions as the equilibrium contact angle decreases. Similar to the first stage, in the second and third stages the surface tension, velocity and displacement of the particle also tend to increase as the equilibrium contact angle decreases, but their amplitudes periodically reduce, especially after the third stage, and the particle enters an almost static state. In addition, the final particle displacement is strongly affected by the equilibrium contact angle, which increases to about −320 µm from −240 µm when the contact angle reduces to 90° from 145°.
The variation of equilibrium contact angle also has a significant affect on the evolution of the force proportion under each stage. As shown in Figure , for the same equilibrium contact angle, all of the surface pressure ratios tend to increase first and then decrease as the particle continuously oscillates under the four conditions, the maximum ratios are all obtained in the third stage, and the amplitude variation gradually increases as the equilibrium contact angle decreases. Unlike the surface pressure ratio, the viscous force ratio changes only slightly during the whole particle oscillation period for all four conditions, and its variation amplitude also sees almost no change as the contact angle varies. Considering the same stage under various contact angle conditions, both the surface pressure and viscous force ratios tend to increase as the contact angle reduces in all stages, especially in the third stage, and the surface pressure rises beyond half the surface tension (its ratio is 0.52) when the equilibrium contact angle is 90°. On the whole, both the effects of surface tension and viscous force gradually decrease with increasing equilibrium contact angle, as shown in Figure .
Figure depicts the variations of average heat flux, contact area ratio and melting and residual time of the particle under various equilibrium contact angle conditions. During particle impact, with decreasing contact angle, both the final contact area and the average contact area during oscillation continuously increase, and their best values basically equal each other. Although the average heat flux of the particle slightly reduces, the total heat transfer from the melt pool increases comprehensively with decreasing contact angle. Thus, the particle melting time is smallest under the 90° condition, which is about 3.3 ms (5.9 ms for 145°). Similar to the decreasing melting time, the residual time also decreases when the contact angle decreases (the time reduces about 25% from 145° to 90°); therefore, as the trad/tcod contour line moves towards 1.25, the heat transfer from the melt pool is also enhanced.
So as to consider the effect of the initial velocity and equilibrium contact angle comprehensively, an investigation of many different combinations of velocity and contact angle have been carried out, and the results for melting time are illustrated in Figure . When the equilibrium angle is 100°, when the velocity is above 4 m/s, the particle submerges quickly; when the equilibrium decreases to 90°, the critical value reduces to 3 m/s. However, when the contact angle increases to 145°, when the velocity is above 3.5 m/s, particle rebound occurs. For other conditions, whose contact angle is between 90° and 145° and velocity is less than 4 m/s, particles will stand statically on the surface after oscillation. The particle behaviour in the oscillation zone directly influences the quality of the formed layer, which should be further analysed. For the conditions located in the oscillation zone, the best initial velocity of particle melting exists under each contact angle condition, the best initial velocity gradually decreases to 0 from 1 m/s as the contact angle decreases to 90° from 145°, as shown in Figure . Furthermore, it also can be observed that the particle melting (capture) ability becomes weaker if the initial velocity is too big or too small, the optimization velocity region is located nearby the best parameter line, and that region broadens with decreasing equilibrium contact angle. For a DLD manufacturing process, if the particle is deposited at low velocity, the weaker the hydrophobia of the particle, the faster the melting of the particle and the more easily it is captured by the melt pool; however, if the particle is strongly hydrophobic, its deposition should be performed near the best velocity parameter line. This has important significance for improving the particle deposition and capture process.
4. Conclusions
In this article, a numerical model of a single particle impacting a high-temperature melt pool, considering the interaction of force, and the mass and heat of the particle and melt pool, has been established based on the coupling of the PF and ALE methods and the N-S equations. An investigation of the particle’s motion and melting behaviour during impact are carried out, and the influence regime of particle velocity and equilibrium contact angle on particle impact is studied as well. The main conclusions are listed as follows.
As a low velocity and hydrophobic particle impacts a melt pool surface, particle oscillation and surface meniscus structure quickly form under the coupled effects of non-equilibrium contact angle, surface tension, surface pressure and viscous force. The surface structure presents a periodical variation from ‘convex hump and concave waist’ to ‘concave hollow and convex waist’ as the particle oscillates, and the amplitudes for both gradually weaken with decreasing driving forces. These phenomena commonly vanish about 2 ms after impact.
Only a small part of the particle has been melted when the particle oscillation is vanishing. Most of the heat in the particle is accumulated from the static heat transfer of the melt pool, and the heat accumulation is dominated by the mushy degree of the particle, the variation of which becomes gentle before becoming total mushy. In addition, under common conditions, the dominant factor of melt pool melting converts to laser irradiation from melt pool heating with the increase of laser power and the decrease of laser spot diameter.
Along with the increasing initial velocity, the wetting effect gradually weakens, the viscous force effect continuously increases, and the surface pressure effect tends to increase first and then decrease. The particle velocity and displacement in each stage gradually increases, but the final displacement and contact area change very little. The particle melting time decreases first and then increases, and a minimum time is obtained at the 1 m/s condition when the equilibrium contact angle is 115°.
As the equilibrium contact angle decreases, the wetting effect gradually increases and the effect of surface pressure and viscous force also rises; furthermore, the velocity, displacement and final contact area increase as well. The particle melting time significantly reduces. Additionally, the optimization initial velocity region for particle melting broadens and the best velocity gradually decreases to 0 from 1 m/s as the equilibrium contact angle decreases.
Furthermore, it can be seen that, for a DLD process under low-speed hydrophobic powder delivery conditions, the vast majority of particles will be in the oscillation state when they impact the melt pool, and the total melting time of those particles is about 3∼5 ms. In this case, during an actual process, to ensure manufacturing quality, the first task is to guarantee that the melt pool exists long enough, which advances a requirement for the scanning speed of the laser beam. Secondly, minimizing the oscillation time as much as possible is beneficial for the rapid balance of particles and reducing the heat loss caused by oscillation. Obviously, reducing the equilibrium contact angle and reducing the particle impact velocity are undoubtedly two effective methods. In addition, since particle melting is far delayed compared with its oscillation process, how to maximize the melting speed during the particle’s stationary state in order to promote manufacturing speed and improve quality is the top priority for actual manufacturing. At present, preheating particles to reduce the heat transfer temperature difference and applying external forces to increase the final heat transfer area are indeed two better options.
The limitations of this study. Firstly, the model established is currently only applicable to single particle capture research, and cannot be applied well to the research involving multi-particle coupling and capture. Secondly, once the influence of melt pool convection is considered, the assumption adopted in this article that the particle shape is unchanged during impact even though part of particle is melting will gradually affect the calculation accuracy along with the increased melt volume. Thirdly, this article does not consider the effect of temperature on physical properties, but instead uses constant properties. In fact, for temperature sensitive materials, this assumption is not suitable. In addition, this article does not investigate the effect of temperature on particle capture, which makes the research conclusions somewhat incomplete for guiding actual manufacturing processes.
Disclosure statement
No potential conflict of interest was reported by the authors.
Additional information
Funding
References
- Akinyemi, L., Iyiola, O., & Akpan, U. (2020). Iterative methods for solving fourth- and sixth-order time-fractional Cahn-Hillard equation. Mathematical Methods in the Applied Sciences, 43(7), 4050–4074. https://doi.org/10.1002/mma.6173
- Bian, Q., Ling, K., Dai, R., & Zeng, M. (2022). Numerical study on heat and mass transport phenomena during a multilayer cladding process for direct laser deposition. Numerical Heat Transfer, Part A: Applications, 82(7), 356–375. https://doi.org/10.1080/10407782.2022.2078631
- Bian, Q., Tan, K., Ling, K., Chen, Y., Zeng, M., & Wang, Q. W. (2022). Transport phenomena and evolution mechanism of the melt pool during a laser-based metal melting process. Journal of Thermal Science and Engineering Applications, 14(12), 120901. https://doi.org/10.1115/1.4053226
- Cui, M., Zhang, C., Zhang, B., Xu, B., Peng, H., & Gao, X. (2022). Numerical solution of phase change heat transfer problems by effective heat capacity model and element differential method. Journal of Computational Science, 60, 101593. https://doi.org/10.1016/j.jocs.2022.101593
- DasGupta, D., Mondal, P. K., & Chakraborty, S., (2014), Thermocapillary-actuated contact-line motion of immiscible binary fluids over substrates with patterned wettability in narrow confinement. Physical Review E, 90(2), 023011. https://doi.org/10.1103/PhysRevE.90.023011
- Dong, X., Hao, G., & Yu, R. (2022). Two-dimensional smoothed particle hydrodynamics (SPH) simulation of multiphase melting flows and associated interface behavior. Engineering Applications of Computational Fluid Mechanics, 16(1), 588–629. https://doi.org/10.1080/19942060.2022.2026820
- Eustatopoulos, N. (2015). Wetting by liquid metals—Application in materials processing: The contribution of the Grenoble group. Metals, 5(1), 350–370. https://doi.org/10.3390/met5010350
- Gadeschi, G. B., Schilden, T., Albers, M., Vorspohl, J., Meinke, M., & Schröder, W. (2021). Direct particle–fluid simulation of flushing flow in electrical discharge machining. Engineering Applications of Computational Fluid Mechanics, 15(1), 328–343. https://doi.org/10.1080/19942060.2021.1877198
- Gatzen, M., Radel, T., Thomy, C., & Vollertsen, F. (2014). Wetting behavior of eutectic Al–Si droplets on zinc coated steel substrates. Journal of Materials Processing Technology, 214(1), 123–131. https://doi.org/10.1016/j.jmatprotec.2013.08.005
- Gorthi, S. R., Mondal, P. K., & Biswas, G. (2017). Magnetic-field-driven alteration in capillary filling dynamics in a narrow fluidic channel. Physical Review E, 96(1), 013113. https://doi.org/10.1103/PhysRevE.96.013113
- Haley, J. C., Schoenung, J. M., & Lavernia, E. J. (2018). Observations of particle-melt pool impact events in directed energy deposition. Additive Manufacturing, 22, 368–374. https://doi.org/10.1016/j.addma.2018.04.028
- Haley, J. C., Schoenung, J. M., & Lavernia, E. J. (2019). Modelling particle impact on the melt pool and wettability effects in laser directed energy deposition additive manufacturing. Materials Science and Engineering: A, 761, 138052. https://doi.org/10.1016/j.msea.2019.138052
- Jacob, B., Drawert, B., Yi, T., & Petzold, L. (2021). An arbitrary Lagrangian Eulerian smoothed particle hydrodynamics (ALE-SPH) method with a boundary volume fraction formulation for fluid-structure interaction. Engineering Analysis with Boundary Elements, 128, 274–289. https://doi.org/10.1016/j.enganabound.2021.04.006
- Ji, B., Song, Q., & Yao, Q. (2017), Numerical study of hydrophobic micron particle’s impaction on liquid surface. Physics of Fluids, 29(7), 0077102. https://doi.org/10.1063/1.4991915
- Kintea, D. M., Breitenbach, J., Thammanna Gurumurthy, V., Roisman, V., & Tropea, C. (2016). On the influence of surface tension during the impact of particles on a liquid-gaseous interface. Physics of Fluids, 28(1), 0012108. https://doi.org/10.1063/1.4939285
- Kistler, S. F., (1993). Wettability: Hydrodynamics of wetting (pp. 327–446). Marcel Dekker.
- Lee, D., & Kim, H. (2011). Sinking of small sphere at low Reynolds number through interface. Physics of Fluids, 23(7), 0072104. https://doi.org/10.1063/1.3614536
- Lin, J. (1999). A simple model of powder catchment in coaxial laser cladding. Optics & Laser Technology, 31(3), 233–238. https://doi.org/10.1016/S0030-3992(99)00046-8
- Mondal, P. K., DasGupta, D., Bandopadhyay, A., & Chakraborty, S. (2014), Pulsating flow driven alteration in moving contact-line dynamics on surfaces with patterned wettability gradients. Journal of Applied Physics, 116(8), 0084302. https://doi.org/10.1063/1.4893705
- Mondal, P. K., DasGupta, D., & Chakraborty, S. (2015). Rheology-modulated contact line dynamics of an immiscible binary system under electrical double layer phenomena. Soft Matter, 11(33), 6692–6702. https://doi.org/10.1039/C5SM01175B
- Mondal, P. K., Ghosh, U., Bandopadhyay, A., DasGupta, D., & Chakraborty, S. (2013), Electric-field-driven contact-line dynamics of two immiscible fluids over chemically patterned surfaces in narrow confinements. Physical Review E, 88(2), 023022. https://doi.org/10.1103/PhysRevE.88.023022
- Nassiri, A., & Kinsey, B. (2016). Numerical studies on high-velocity impact welding: Smoothed particle hydrodynamics (SPH) and arbitrary Lagrangian–Eulerian (ALE). Journal of Manufacturing Processes, 24, 376–381. https://doi.org/10.1016/j.jmapro.2016.06.017
- Prasad, H. S., Brueckner, F.; & Kaplan A. F. H., (2019), Powder catchment in laser metal deposition. Journal of Laser Applications, 31(2), 0022308. https://doi.org/10.2351/1.509613031.022308
- Qi, H., Mazumder, B., & Ki, H., (2006), Numerical simulation of heat transfer and fluid flow in coaxial laser cladding process for direct metal deposition. Journal of Applied Physics, 100(2), 0024903. https://doi.org/10.1063/1.2209807
- Schönecker, S., Li, X., Börje, J., Se, K. K., & Levente, V. (2015). Thermal surface free energy and stress of iron. Scientific Reports, 5(1), 14860. https://doi.org/10.1038/srep14860
- Shyam, S., Dhapola, B., & Mondal, P. K. (2022). Magnetofluidic-based controlled droplet breakup: Effect of non-uniform force field. Journal of Fluid Mechanics, 944, A51. https://doi.org/10.1017/jfm.2022.504
- Thompson, S. M., Bian, L., Shamsaei, N., & Yadollahi, A. (2015). An overview of direct laser deposition for additive manufacturing; part I: Transport phenomena, modeling and diagnostics. Additive Manufacturing, 8, 36–62. https://doi.org/10.1016/j.addma.2015.07.001
- Tsigaridis, K., Krol, M., Dentener, F., Balkanski, Y., Lathière, J., Metzger, S., Hauglustaine, D. A., & Kanakidou, M. (2006). Change in global aerosol composition since preindustrial times. Atmospheric Chemistry and Physics, 6(12), 5143–5162. https://doi.org/10.5194/acp-6-5143-2006
- Vella, D., Lee, D., & Kim, H. (2006). The load supported by small floating objects. Langmuir, 22(14), 5979–5981. https://doi.org/10.1021/la060606m
- Viswanathan, S. (1999). Numerical study of particle collection by single water droplets. Industrial & Engineering Chemistry Research, 38(11), 4433–4442. https://doi.org/10.1021/ie990199s
- Volpp, J. (2019). Behavior of powder particles on melt pool surfaces. The International Journal of Advanced Manufacturing Technology, 102(5-8), 2201–2210. https://doi.org/10.1007/s00170-018-03261-1
- Volpp, J., Prasad, H. S., Riede, M., Brueckner, F., & Kaplan, A. F. H. (2018). Powder particle attachment mechanisms onto liquid material. Procedia CIRP, 74, 140–143. https://doi.org/10.1016/j.procir.2018.08.064
- Wang, A., Song, Q., & Yao, Q. (2015), Behavior of hydrophobic micron particles impacting on droplet surface. Atmospheric Environment, 115, 1-8. https://doi.org/10.1016/j.atmosenv.2015.05.053
- Wen, S., & Shin, Y. C., (2011), Modeling of the off-axis high power diode laser cladding process. Journal of Heat Transfer, 133(3), 031007. https://doi.org/10.1115/1.4002447
- Worthington A. M., & Cole, R. S. (1897). Impact with a liquid surface, studied by the aid of instantaneous photography. Philosophical Transactions of the Royal Society of London. Series A, Containing Papers of a Mathematical or Physical Character, 189, 137–148. https://doi.org/10.1098/rsta.1897.0005
- Xiao, G., Du, Y., Wang, Q., Guo, L., & Wang, M. (2017). Freezing characteristics of super cooled water droplet in consideration of non-equilibrium effect (Chinese). Acta Aeronautica et Astronautica Sinica, 38(2), 520703. https://doi.org/10.7527/S1000-6893.2016.0309
- Yang, H., & He, Y. (2010). Solving heat transfer problems with phase change via smoothed effective heat capacity and element-free Galerkin methods. International Communications in Heat and Mass Transfer, 37(4), 385–392. https://doi.org/10.1016/j.icheatmasstransfer.2009.12.002
- Zhang, F., Wang, K., Zhao, H., Qiu, Y., Wang, G., Zhang, J., & Tan, H. (2020). Interaction between powder particle and gas-liquid interface of the melt pool during laser solid forming process. Optics and Lasers in Engineering, 129, 106065. https://doi.org/10.1016/j.optlaseng.2020.106065