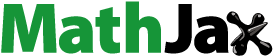
Abstract
Towards the development of emission-free hydrogen gas turbines with wider operability limits, the combined effects of lean premixed combustion, dual combustion (combustion staging), hydrogen enrichment, and oxy-fuel combustion on the combustion and emission characteristics of CH4/H2/O2/CO2 flames are investigated in a dual annular counter-rotating swirl burner. The central stream is of a higher equivalence ratio (∅) of 0.9 for stable flame ignition, whereas the annular stream is of a lower ∅ of 0.65 to maintain the burner’s environmental performance. The stratified flame reactions are modelled using the partially premixed combustion model, which has been validated using the available experimental data. The inner recirculation zone becomes smaller as the hydrogen fraction (HF) becomes higher until it vanishes and there exists only an outer recirculation zone when the secondary hydrogen fraction approaches 100%. For 100% HF in both primary and secondary flow, the maximum combustion temperature within the combustor was observed to be as high as 2350 K. Increasing HF makes the flame more compact and anchored to the burner centre body with faster kinetics, less ignition delay time, and, accordingly, with increased potential for flashback. The product formation rate was found to increase in the inner shear layer at higher HF, implying a higher flame intensity and a more compact flame. The hydrogen fraction of the primary stream (maintained at higher equivalence ratio) is observed to be dominant in regulating CO mole fractions. Stable well-anchored flames are obtained over the considered fuel mixtures, indicating the role of stratified combustion in widening the operability of gas turbine combustors under stratified combustion conditions.
1. Introduction
Increasing greenhouse gas (GHG) emission with growing energy demand necessitates significant advancement in the combustion technologies used for power generation. Over the last few decades, gas turbine combustion has become one of the preferred energy generation processes from fossil fuel owing to its inherent low emission over the coal-fuelled combustion. However, the relativity cleaner gas turbine combustion still produces harmful emissions. About 21% of global CO2 emission is caused by the utilization of gaseous fuels (Ritchie & Roser, Citation2020). For this reason, gas turbines (GTs) are continuously subjected to stricter emission regulations and are upgraded with various low emission combustion techniques such as steam injection, lean premixed combustion, and hydrogen combustion (Kim, Citation2019).
Lean premixed combustion (LPM) combined with hydrogen addition (Alhuyi et al., Citation2022) has been among the most focused areas in gas turbine combustion in the last decade. Although having high potential, this combustion configuration suffers from several issues, such as elevated NOx emission (Boyce, Citation2011, Citation2012) and flame instabilities (Ali et al., Citation2020; Mousavi et al., Citation2020; Roy & Gupta, Citation2022). Adopting oxy-fuel combustion with recirculation of exhaust flue gases is an effective approach to eliminate NOx emission, as this technique deals with pure oxygen as oxidizer rather than air (Aliyu et al., Citation2022; Nemitallah et al., Citation2022). Exhaust gas or CO2 recirculation in oxy-combustion helps to regulate the excess temperature resulting from combustion in pure oxygen (Haque et al., Citation2020). Furthermore, the instabilities, both static and dynamic, in LPM−hydrogen combustion can be minimized through stratification of the inlet stream (Nemitallah et al., Citation2021; Peng et al., Citation2016).
The dual annular counter-rotating swirl (DACRS) burner is one of the promising technologies for improving the stability of LPM flames and for extending the blowout limit to much leaner conditions in order to maintain combustor environmental performance and increase turbine turndown ratio. In a DACRS burner, the flow of reactants is separated into two streams: (a) a central fuel-rich stream that generates a stable flame in order to sustain ignition for (b) an outer stream of much lower equivalence ratio to sustain environmental performance. This DACRS technology helps to elevate the shear between the two flames, which eventually supports the transfer of active radicals from the central fuel-rich flame to the outer leaner flame (Joshi et al., Citation1994) leading to a more stable overall flame with wider operability and lower emissions of the combustor. It has been reported that the stratified combustion technique has larger operational limits and greater flame speed compared with non-stratified counterparts (Galizzi & Escudié, Citation2006; Kang & Kyritsis, Citation2005; Kim et al., Citation2016). Another way to reduce pollutant emissions and to control heat release from chemical reactions during gas turbine combustion is to conduct the combustion in stages. Air staging, fuel staging, and combined air–fuel staging are the main methods by which staging techniques can be applied. In air staging, three zones are used: primary, secondary, and dilution zones. Fuel is injected into the primary air within the primary zone and the mixture is ignited to generate most of the heat release within this zone. This zone keeps the equivalence ratio near stoichiometry in order to sustain the reaction’s stability and to avoid blowout. The other portion of heat release happens within the secondary zone, where fuel is completely burned. Finally, a supply of air is utilized to sustain the exhaust temperature in a range that does not affect the turbine blades (Borchert & Szymczyk, Citation2011; Samuelsen & Burn, Citation2006). A similar approach is adopted in the fuel staging inside the combustor, where the fuel is supplied in more than one region to have different zones with different air–fuel compositions (Kiameh, Citation2003). Fuel staging helps to mitigate instabilities during combustion in industrial hardware (Bulat et al., Citation2007; Cohen et al., Citation2011). Finally, for combined air–fuel staging, the fuel–oxidizer mixtures in the flow paths of the burner have different equivalence ratios. Kim and Hochgreb (Citation2011) reported that the splitting of equivalence ratios has a major impact on the flame dynamics and flame shape. The heat release rate was found to increase when stratification was applied in fuel-lean conditions, owing to the differential burning velocities of lean and rich zones that produced more flame surface area (Pasquier et al., Citation2007). Lean blowout limits are extended in stratification to a much leaner range. Fuel distribution has greater influence on the flame stabilization and structure mechanisms in stratified swirl flames compared with the corresponding homogeneous ones (Kang & Kyritsis, Citation2009). Despite covering a wide range of stratified combustion challenges, none of these investigations examined oxy-combustion, hydrogen addition or hydrogen combustion with stratified combustion.
Another method for improving further the flame stability and speed in gas turbine combustors is hydrogen enrichment (Najafi et al., Citation2021; Sui et al., Citation2021). For the same equivalence ratio, hydrogen addition produces higher flame temperatures and widens lean blowout operational limits (Nogenmyr et al., Citation2011). Hydrogen addition was found to boost the flame speed of hydrocarbons (Boushaki et al., Citation2012; Guo et al., Citation2005; Kim et al., Citation2009). The stability limits shift towards leaner conditions with the increase of hydrogen addition. Kim et al. (Citation2009) analysed the hydrogen-enrichment effect on methane premixed swirling air flames. They found that the temperature of the reaction region increased, and the flow swirl decreased, owing to the higher combustibility of hydrogen that induces faster reactions and higher combustion temperatures, which accelerates the flow axially resulting in reduced levels of flow swirl. Gersen et al. (Citation2008) studied the ignition delay time of hydrogen−methane mixtures of various concentrations in a rapid compression machine. They reported a decrease in the ignition delay time with the increase of hydrogen enrichment level, which resulted in faster reactions with high potential for flame flashback. Although hydrogen addition helps in lowering the lean blowout limit (Gersen et al., Citation2008), it increases the chances for flashback owing the increased flame speed (Strakey et al., Citation2007). However, these studies were on air-combustion only; no characteristics of oxy-combustion were investigated. On the other hand, Ali et al. (Citation2020) and Imteyaz et al. (Citation2020) studied the operability in terms of flashback−blowout and emission in hydrogen-enriched oxy-methane combustion, but flame stratification for CH4/H2/O2/CO2 was not covered in their study.
The implementation of the concepts of flame stratification and oxy-fuel combustion together with hydrogen enrichment in gas turbine combustion has not been reported in the open literature. Based on the foregoing discussion, the goal of the current study is to investigate the effects of combining the concepts of LPM combustion, combustion staging, hydrogen enrichment, and oxy-fuel combustion to examine their effects on the combustion and emission characteristics in a DACRS burner. This is necessary given the widespread interest in clean and effective power generation in combustion systems. Oxy-fuel combustion eliminates NOx emissions, but it also results in less stable flames and restricted combustor operation, especially close to the blowout limit. By introducing the concepts of flame stratification and hydrogen enrichment, it is possible to maintain environmental performance while also expanding a combustor’s operability limit close to the blowout limit and allowing for part-load operation. Hence, the present research examines the effect of hydrogen enrichment on combustion and emission parameters in a DACRS combustor with oxy-fuel combustion for wider operability and cleaner power generation in gas turbines. Also, the study targets the representation of data on pure hydrogen combustion for the sake of the development of hydrogen gas turbines.
2. Numerical model setup
2.1. Combustor description
Oxy-combustion in a model DACRS burner is investigated for CH4−H2 fuel under an LPM scheme in different stratification conditions. The burner and combustor components are presented in Figures (a) and (b), respectively, with all dimensions in millimetres. Figure (a) describes the dual DACRS burner. The burner encompasses two annular inlets: the primary stream (inner) and the secondary stream (outer). The primary inlet has inner and outer diameters of 0.4 and 5 mm, respectively, while those for the secondary stream are 7 and 29 mm, respectively. Each stream of fuel−oxidizer mixture is directed through one of two swirlers before reaching the combustion chamber. The swirlers rotate the flow in opposite directions, with each of them having the same swirl blade inclination angle of 45° from the central axis, to induce a counter-rotating effect between the primary and the secondary streams. A steel centre body placed at the centre of the burner has a cone angle of 20° and a diameter of 9.5 mm. The inlet sections are surrounded by a 10 mm thick steel base plate. Lastly, a quartz tube of 300 mm length, 38.1 mm inner diameter, and 6 mm thickness surrounds the combustion chamber, as shown in Figure (b). The combustor is allowed to have a high aspect ratio with an expansion angle of 45° at the burner outlet to allow for a stronger outer recirculation zone and better flame stability.
Fuel−oxidizer mixtures with fully premixed conditions enter the combustor at bulk throat velocities of 6 and 3 m/s for the primary and secondary streams, respectively. The burner has a power output range of 4.1 MW/m3/atm at a fuel volume flow rate of 6 L/min to 6.2 MW/m3/atm at a fuel volume flow rate of 10 L/min. The majority of industrial gas turbines operate between 3.5 and 20 MW/m3/atm; therefore, these circumstances are equivalent. It is a very common approach to design gas turbine combustors with fixed inlet flow velocity to achieve the design limitation of fixed pressure drop across the combustor. Based on such conditions, the inlet velocities are fixed at values that achieve such operational ranges at realistic inlet flow Reynolds numbers. Exhaust gas recirculation (EGR) is considered to limit the flame temperature in oxy-combustion by adding CO2 to the fuel−oxygen mixture. The addition of CO2 in the oxidizer stream is controlled and varied using oxygen fractions (OFs), as expressed in Equation (1). The oxygen fraction in all cases in this study is maintained at a fixed value of 35% for both inlets with fixed equivalence ratios (∅) for both primary and secondary streams of 0.9 and 0.65, respectively. The simulations are performed while varying the hydrogen fractions (HFs) in the fuel mixture, as presented in Equation (2), to examine the effects of hydrogen addition and flame stratification on combustion, operability, and emission characteristics of oxy-methane flames in a DACRS burner for gas turbine combustion applications.
(1)
(1)
(2)
(2)
2.2. Governing equations
2.2.1. Turbulence model
The two-dimensional oxy-combustion turbulence model (i.e. the standard k−ϵ model) of the Reynolds-averaged Navier−Stokes equations is implemented using the commercial code ANSYS® Fluent® 19R3 (ANSYS Fluent, Citation2011), where the dissipation rate (ϵ) and kinetic energy (k) are calculated using Equations (3) and (4), respectively. The quantities and
denote turbulence kinetic energy generation due to velocity gradients, while
represents the impact of the fluctuating dilatation in the compressible turbulence with regard to the overall rate of dissipation. The terms
,
, and
are constants, and the quantities
and
correspond to Prandtl numbers for both k and ϵ, respectively. Ultimately, as seen in Equation (5) (ANSYS Fluent, Citation2011), the evaluation of the turbulent viscosity
is done through combining k and ϵ:
(3)
(3)
(4)
(4)
(5)
(5)
Radiative heat transfer has been evaluated using discrete ordinates (DOs) modelling. This model is effective and recommended for simulating a wide-range spectrum of optical thickness for oxy-fuel flames (Porter et al., Citation2010). The weighted sum grey gas model (WSGGM) is used to get the gas mixture’s absorption coefficient, which is validated over a large range of the operating conditions (Johansson et al., Citation2011). The emissivity weighting factor for a grey gas is estimated based on the reported experimental data in the literature (Coppalle & Vervisch, Citation1983; Smith et al., Citation1982).
2.2.2. Reaction model
Implementing CO2-dilution in oxy-fuel combustion is done using the partially premixed combustion model. The variation of the inlet mixture fraction is fixed at zero to sustain constant equivalence ratio at the inlet. In ANSYS Fluent, the partially premixed model is a combination of the diffusion and premixed combustion models, having the benefits of both predecessor models simultaneously, which allows for more control of reactions and species (ANSYS Fluent, Citation2011). Equations (6) and (7) are the transport equations for the progress variable (PV) and mean mixture fraction, respectively. For evaluating and
, Equations (8) and (9) are used, where
correspond to the turbulent Prandtl number (0.85), the progress term, and the turbulent Schmidt number (0.70), respectively (Zimont et al., Citation1997). To determine the mean reaction rate, the Zimont method (Zimont et al., Citation1997) is used as presented in Equation (10). Such model has been well-validated in the literature and has shown suitability for modelling highly reactive/turbulent hydrogen flames (Zimont, Citation1977; Zimont & Lipatnikov, Citation1992). The definition of the different terms in Equation (10) can be found in our previous publication (Haque et al., Citation2022).
(6)
(6)
(7)
(7)
(8)
(8)
(9)
(9)
(10)
(10)
PV and the probability density function (PDF) are used to calculate the species fractions and temperature, as presented in Equation (11). More details about the different parameters can be found in Haque et al. (Citation2022).
(11)
(11) The PFR represents the product generation rate for all reactions, including intermediate and final products, and can be calculated using Equation (12):
(12)
(12)
(13)
(13) where T0 stands for layer temperature, Tb for burned temperature, Tu for unburned temperature, and
for the fraction of unburned fuel by mass. The inner layer temperature in the temperature profile of premixed flames signifies the change from the inert preheat zone to the reaction zone, and as a result, it is the location where the second derivative vanishes, and the slope is greatest (Muller et al., Citation1997). SL was calculated initially considering the overall values of the operating parameters for both streams, i.e. at overall equivalence ratio, overall hydrogen fraction, and overall oxygen fraction. As the calculations proceeded, the composite chemical kinetic model used to simulate the laminar burning velocity of premixed flames was utilized to determine the mixture characteristics, Tb and T0, which were used to estimate the thickness of the flame (Okafor et al., Citation2016). The studies by Göttgens et al. (Citation1992) and Salem (Citation2018) can be read for more details on SL computation.
The thermal power (TP) delivered at a combustor’s inlet, which is the power density (PD), can be evaluated using Equation (14). The terms and
are the mass flow rate and the fuel calorific value, and the terms
and
are the combustor volume and combustor volume, respectively. Here, the calorific values of the CH4, CO, and H2 are 55.5, 10.1, and 141.8 MJ/kg, respectively (Ali et al., Citation2021; Haque et al., Citation2022).
(14)
(14)
2.3. Boundary conditions
Two-dimensional (2D) axisymmetric geometry is considered to model the stratified oxy-combustion, as shown in Figure (b) above, in the axi-symmetric swirl mode. This mode is a pre-existing simulation scheme in ANSYS Fluent software for modelling swirling flows in axi-symmetric geometries. The gravitational effect along with a steady-state pressure-based solver is selected. The SIMPLE scheme is used for the iterative solution of the pressure–velocity coupling. For the numerical simulation, partial differential equations from previous sections are discretized, where a second-order upwind scheme is used for spatial discretization of all the parameters. Convergence is checked by setting the residuals at 10−8 and through monitoring the parameters.
A velocity inlet boundary condition is selected for both inlets, and the pressure outlet boundary is considered for the combustor outlet. An inlet velocity boundary condition is considered at the burner inlet with a flow direction to produce a swirl effect; and the temperature and fuel mass fractions (i.e. mean mixture fractions) are identified as well. For the centre body and steel base, they are specified as no-slip adiabatic walls. A semitransparent wall is defined to simulate the quartz with a mixed (convection and radiation) heat transfer boundary condition. The ambient temperature is set to 300 K, while 20 W/m2 K is considered for the external convective heat transfer coefficient. The model parameters and boundary conditions are presented in Table .
Table 1. Summary of model and boundary conditions employed in the DACRS burner simulation.
2.4. Meshing and experimental comparison
The two-dimensional computational geometry is divided into numerous tetrahedral meshing elements, for each of which the governing equations are solved. A grid independence study is performed through the generation of three meshes of 24,641, 50,000, and 75,000 finite volume cells. Using the three meshes, the model is built up to solve for the flow field in scenario 1 of non-reacting flow circumstances. In comparison to the mesh of 24,641 cells, the distributions of the axial velocity profiles for the meshes of 50,000 and 75,000 cells show no appreciable differences in the profiles, less than a 3% variation. Based on that, all numerical computations are carried out using the 24,641-cell mesh shown in Figure . The selected mesh for the study contains elements with minimum face area, 2D volume, minimum orthogonal quality, and maximum aspect ratio of 1.66 × 10−4 m2, 4.03 × 10−8 m3, 0.83 and 2.49, respectively.
The developed model for hydrogen enriched methane combustion is validated against the authors’ earlier experimental work (Nemitallah et al., Citation2019) at ∅ = 1.0, OF = 30%, and varying HF, as illustrated in Figure . The temperature distribution shows a slight overprediction of temperature with the numerical model in the axial direction. This can be related to the cooling effect in the burner port, which is not addressed in the numerical model, quenching the reaction resulting in a small decrease in the combustion temperature (Nemitallah et al., Citation2019). Temperature levels are observed to be increasing with the increase in HF, which might be attributed to the increase in the reactivity and fuel diffusion, or a slight increase in the adiabatic temperature due to an increase in HF. Temperatures are close to one another while varying HF in the axial direction, whereas the temperature decreases in the radial direction from the centre of the combustor to the combustor wall owing to the impact of the outer recirculation zone (ORZ) and heat transfer through the wall. The applied partially premixed combustion model has been validated several times in several publications by the authors (Aliyu et al., Citation2022; Nemitallah et al., Citation2019, Citation2021, Citation2022), and proved to be of very high accuracy.
Figure 3 Comparison of experimental (dots) and numerical (lines) results in terms of axial temperature profiles at ∅ = 1.0, OF = 30%, and varying HFs (Nemitallah et al., Citation2019).

3. Results and discussion
Stratified oxy-combustion flames are studied for methane−hydrogen fuel mixtures. The overall oxygen fractions are kept constant at 35%, while the Ø and HF are varied in both primary and secondary inlets. The cases selected for numerical analysis are listed in Table , where the bulk throat velocities are 6 and 3 m/s for the primary and secondary inlets, respectively.
Table 2. Feed fuel−oxidizer composition for the selected cases.
3.1 Effects of H2-level on laminar flame speed (LFS) and power density
Flame behaviour is initially compared with respect to LFS and combustor PD in this section. The variations in LFS with different stratification and hydrogen fractions are depicted in Figure , in which LFS increases with an increase in HF, where a sharp increase can be observed in the 100% HFp cases. This may be attributed to the reaction between H radicals and oxygen molecules (i.e. ). LFS is affected by a range of intermediate active radicals; however, increasing the HF results in a significant and certain increase in the number of H radicals. As the HF increases, the number of H radicals also increases in the reactant mixture, which react with O2, causing the forward reaction, which consequently promotes the LFS (Li et al., Citation2017; Miao et al., Citation2014; Wu et al., Citation2011; Yu et al., Citation1986). LFS is calculated using CHEMKIN software considering the overall values of the operating parameters for both streams, i.e. the overall equivalence ratio, overall HF, and overall oxygen fraction. On the other hand, PD slightly decreases with an increase in HF, where the decrease is significant in the 100% HFp cases. The PD values depend on the mass flow rates
and calorific values
o tfhe fuel mixture for fixed pressure and combustor volume (see Equation 14). Even though hydrogen has a higher calorific value per unit mass, the overall mass flow rate of the hydrogen reduces (since hydrogen has considerably lower density than methane) when increasing HF at constant flow velocities, which causes the PD to go down with the rise in HF.
3.2 Effect of H2-level on flame macrostructure
Since in actual practice OH radicals are utilized for heat release and flame visualization, the flames are presented in terms of their corresponding OH contours. Figure shows the flame structure in terms of OH contours for the selected cases. As the HF values increase in both inlets, the flame becomes more compact, and the effect of the inner recirculation zone (IRZ) increases (the velocity streamlines along with the flow vorticity are presented in Figures and , respectively). Hydrogen addition increases the reactivity between the reactants, which in turn makes the flame stronger. In addition, the size of the zone occupied by OH radicals decreases with increasing HF. However, the concentrations of OH radicals increase with hydrogen addition. Furthermore, aside from the laminar flame speed, hydrogen addition increases temperature, turbulent flame speed, and varies Lewis number (the ratio of thermal to mass diffusivities) as the hydrogen has higher diffusivity (Yuan et al., Citation2007). All these factors contribute to more compact flames with increased hydrogen. For the cases of HFs of 100%, as we considered fixed inlet flow velocity, replacement of CH4 with H2 in the secondary stream (where most of the flow is introduced to the combustor) results in significant reductions in the fuel mass flow rate and combustor power, and both swirl and reaction intensities are reduced resulting in lower concentrations of intermediate OH radicals and the flame elongates in the axial direction. The amount of diluent is also reduced significantly with increasing HFs, which may result in a local increase in the flame temperature with increasing HFs, although the total fuel mass flow rate is reduced.
3.3 Flame−flow interactions when varying H2-levels
Figure shows the axial temperature distribution of the combustor. In all cases, the temperature of the combustor increases from the inlet up to Z/D = 0.1, then starts decreasing. The decrease in temperature is gradual in the HFs = 100% cases, while the temperature reduction curve is steeper for 25% and 50% HFs. Pure hydrogen flames are characterized by very small size near the combustor inlet, especially with the present combustor design of the DACRS burner, which enhances flow mixing and even results in shorter flames. The higher temperatures for the other flames are due to the extended flame length at lower hydrogen content. The amount of oxygen and diluent are reduced significantly with increasing HFs, as the total fuel mass flow rate is significantly reduced when replacing CH4 with H2 in the fuel mixture at fixed inlet flow velocity. This may justify the increase in combustion temperature with increasing HFs, although the total fuel mass flow rate is significantly reduced. As shown in Figure , the IRZ in the 25% and 50% HFs cases produces convective heat circulation, which contributes to the sharp decrease in axial temperature. IRZ shifting downstream with elevated hydrogen has also been observed for single swirling flow in the authors’ earlier study on hydrogen-enriched oxy-methane combustion (Nemitallah et al., Citation2019). For the 100% HFs cases, the absence of IRZ causes lower heat recirculation from the centre axis of the combustor resulting in gradual reduction of temperature in the axial direction. With the increase in HF, the IRZ downstream makes the flame compact and stronger, until the HFs reaches 100% where the IRZ is not present, and the outer recirculation zone (ORZ) is stretched along the axial direction as a result. Furthermore, the temperature also increases with the HF owing to increased reactivity. For 100% HF in both primary and secondary flow (i.e. case 9), temperatures as high as 2350 K are observed. Increasing HF makes the flame more compact and anchored to the burner centre body with faster kinetics, less ignition delay time, and, accordingly, with increased potential for flashback, which should be avoided in gas turbines.
Figure 6. Velocity streamlines mapped over temperature contours for cases 1–9 (the colour code is specified based on specific ranges from MIN to MAX values for each case).

Figure 7. Vorticity magnitude contours for cases 1–9 (the colour code is specified based on specific ranges from MIN to MAX values for each case).

Figure 8. Temperature distribution in the axial direction through the combustor centreline for cases 1–9.

Similar to temperature, the vorticity level also rises with the HF in Figure . Vorticity magnitude in the secondary flow increases as compared to the primary counterpart with HFs. The vorticity, however, is higher in the primary stream when the HF in both inlets reaches 100%. Increased vorticity and flame speed aide in the shifting of IRZ and axial stretching of ORZ when the HFs is elevated, as the secondary flow has a higher flow rate compared to that of the primary flow (more than 10 times as compared to the primary mass flow rate). However, it can also be observed that the ORZ is comparatively shorter in case 9 (HFp = 100%, HFs = 100%) than in cases 3 (HFp = 25%, HFs = 100%) and case 6 (HFp = 50%, HFs = 100%).
3.4 Effects of H2-levels on the main combustion characteristics
In this section, the combustion characteristics of the flames are studied based on the combustion progress variable (PV) and Damköhler number (Da). Figure illustrates the PV contours at the combustion inlet for different conditions. The flame is mainly stabilized on the inner shear layer (ISL); however, the outer shear layer (OSL) is slightly visible with increased HF. For 100% HFs, OSLs are observed on the flame surface. Similar distributions of the progress variable are shown for HFp lower than 100% with stretched flames toward the combustor wall due to the effect of both IRZ and ORZ. The transition length from unburnt to burnt mixture, i.e. the length of the zone represented by high intensity of the progress variable, is significantly lower in the 100% HFs cases (i.e. cases 3, 6, and 9), which is in-line with the results from Tang et al. (Citation2015). Figure shows the characteristics of the flame in terms of Da. Reaction rates are expressed in terms of Da, where higher values indicate fast reaction rates and lower values mean the reactions are kinetically controlled. The Damköhler number, assessed from the Equation (15) below, indicates to the proportion of rate of reaction to rate of diffusion. The terms and
depict the scale root mean square (RMS) of the fluctuation of velocity and the integral length.
(15)
(15) The values of the Da number presented in Figure are domain averaged values for both the primary and the secondary flames in the overall operating conditions, i.e. at overall equivalence ratio, overall hydrogen fraction, and overall oxygen fraction. The case of 100% HFs has the highest Da number in all three primary HFs, which indicates fast reaction rates. This may be attributed to the natural behaviour of hydrogen as being more reactive and diffusive than methane. For HF = 100% in the primary flow, the Da number is reduced. This can be attributed to the fact that the primary mass flow rate is much lower than the secondary flow rate. Even increasing hydrogen content reduces the total mass flow rate as the inlet flow velocity is fixed. That’s why the hydrogen concentration in the secondary stream has the dominating effect over that in the primary flow.
3.5 Emission attributes
Ideally for oxy-combustion, the exhaust stream consists of CO2 and H2O. However, in reality some CO might exist in the exhaust stream, which is toxic and considered as one of the major reasons for air pollution problems. In this section, the contours for CO mole fraction distributions inside the combustor are examined. Figure shows the CO contour on the centre plane of the combustor for the selected cases. The CO mole fractions are observed near the primary inlet and are seen to be reducing with the rise in HFp. A reduction in methane mole fraction is one of the reasons behind this drop in CO, as the C atoms are reduced with elevated hydrogen concentrations. However, some CO concentrations are still visible even with 100% hydrogen fractions. This is due to the dissociation of CO2 in the oxidizer mixer at higher combustor temperatures. The concentration of CO reduces as the produced CO again reacts with available oxygen atoms as the flow progresses downstream. In fact, CO concentrations near the combustor outlet are very close to zero for all flames with the increase of hydrogen content which reduces the carbon sources in the fuel. We present such CO contours to reflect the flame core where CO is highly generated when methane fuel is present, in addition to reflecting the CO2 dissociation (as the oxidizer component) at higher combustion temperatures.
4. Conclusions
The combustion characteristics and behaviour of premixed oxy-flames in different methane−hydrogen mixtures are investigated numerically in a dual annular counter-rotating swirl (DACRS) combustor. Primary and secondary velocities have been maintained at 6 and 3 m/s, at 35% overall oxygen fraction (OF). The hydrogen fraction in both the inlet mixtures varied from 25 to 100% to determine the influence of hydrogen addition on flame, flow, and emission characteristics. The following concluding remarks are made based on the results from the numerical simulations.
Hydrogen addition increases laminar flame speed and vorticity, which is attributed to the shifting of the inner recirculation zone (IRZ) downstream, making the flame stronger and more stable.
The inner recirculation zone (IRZ) vanishes at 100% hydrogen fraction (HF) in the secondary inlet, while the outer recirculation zone (ORZ) is stretched in the axial direction.
For fixed inlet velocities, increased hydrogen levels reduce the combustor power density (PD).
Flames are mainly stabilized on the inner shear layer (ISL). Increasing the hydrogen content in the fuel results in a faster increase of the progress variable in the axial direction and to a shorter flame.
For 100% HF in both the primary and secondary flows, the combustion temperature is observed to be as high as 2350���K. Increasing HF makes the flame more compact and anchored to the burner centre body with faster kinetics, less ignition delay time, and, accordingly, with increased potential for flashback.
Concentrations of OH and CO decrease within the combustor and occupy smaller areas at higher HFs. The hydrogen fraction of the primary stream (maintained at higher equivalence ratio) dominates the spatial CO distribution in the combustor.
Stable well-anchored flames are obtained over the considered ranges of fuel mixtures of the primary and secondary streams indicating the role of stratified combustion in widening the operability of gas turbine combustors.
The topic of hydrogen combustion is hot and needs more investigation to characterize the hydrogen flames in more detail. There follows a list of points recommended for future research work.
Determination of required flame length to fully oxidize hydrogen along gas turbine engines operating envelope. This needs to be a comprehensive study that includes preheat, pressures, and temperatures that gas turbines operate at. It also includes the impact of pressure drop and mixer configuration to be included.
Enabling combustion systems to run on natural gas and 100% hydrogen via axial fuel staging. Requires multiple configurations and geometrical variations to optimize the results along with operating conditions.
Modelling hydrogen blended fuels emissions, NOx, and CO, and comparing to experimental data. The study needs to include different mixer configurations, pressure drops, and operating conditions.
The authors would like to acknowledge the support received from King Fahd University of Petroleum & Minerals (KFUPM) through the KFUPM Consortium for Hydrogen Future [project number H2FC2309]; also, the support received from King Abdullah City for Atomic and Renewable Energy (K.A. CARE) is highly appreciated.
Disclosure statement
No potential conflict of interest was reported by the authors.
References
- Alhuyi, M., Alavi, M., Salem, M., & Assad, M. (2022). Utilization of hydrogen in gas turbines: A comprehensive review. International Journal of Low-Carbon Technologies, 17, 513–519. https://doi.org/10.1093/ijlct/ctac025
- Ali, A., Nemitallah, M. A., Abdelhafez, A., Hussain, M., Kamal, M. M., & Habib, M. A. (2021). Comparative analysis of the stability and structure of premixed C3H8/O2/CO2 and C3H8/O2/N2 flames for clean flexible energy production. Energy, 214, 1–10. https://doi.org/10.1016/j.energy.2020.118887
- Ali, A., Nemitallah, M. A., Abdelhafez, A., Imteyaz, B., Kamal, M. M., & Habib, M. A. (2020). Numerical and experimental study of swirl premixed CH4/H2/O2/CO2 flames for controlled-emissions gas turbines. International Journal of Hydrogen Energy, 45(53), 29616–29629. https://doi.org/10.1016/j.ijhydene.2020.07.210
- Aliyu, M., Nemitallah, M., Said, S., Abdelhafez, A., Mansir, I., & Habib, M. (2022). Role of adiabatic flame temperature on controlling operability of a micromixer-based Gas turbine combustor holding premixed Oxy-flames for carbon capture. Journal of Energy Resources Technology, 144(10), 102307. https://doi.org/10.1115/1.4053983
- Borchert, U., & Szymczyk, J. A. (2011). Fluidic analyses of a model Gas turbine combustion chamber with and without combustion under actual operating conditions. XX. International Conference Research and Practice Didact. Mod. Mech. Eng., Stralsund, NETL, Morgantown, WV.
- Boushaki, T., Dhué, Y., Selle, L., Ferret, B., & Poinsot, T. (2012). Effects of hydrogen and steam addition on laminar burning velocity of methane–air premixed flame: Experimental and numerical analysis. International Journal of Hydrogen Energy, 37(11), 9412–9422. https://doi.org/10.1016/j.ijhydene.2012.03.037
- Boyce, M. (2011). Gas turbine engineering (Handbook-4th Edition). Butterworth-Heinemann.
- Boyce, M. P. (2012). Combustors. In Gas turbine Eng. Handb (4th ed., pp. 427–490). Elsevier.
- Bulat, G., Skipper, D., McMillan, R., & Syed, K. (2007). Active control of fuel splits in gas turbine DLE Combustion systems. Vol. 2 Turbo Expo 2007, ASMEDC; 2007, p. 135–44.
- Cohen, J., Hagen, G., Banaszuk, A., Becz, S., & Mehta, P.. (2011). Attenuation of gas turbine combustor pressure oscillations using symmetry breaking. In 49th AIAA Aerospace Sciences Meeting including the New Horizons Forum and Aerospace Exposition (pp. 60).
- Coppalle, A., & Vervisch, P. (1983). The total emissivities of high-temperature flames. Combustion and Flame, 49(1–3), 101–108. https://doi.org/10.1016/0010-2180(83)90154-2
- ANSYS Fluent (2011). Theory guide. ANSYS Inc.
- Galizzi, C., & Escudié, D. (2006). Experimental analysis of an oblique laminar flame front propagating in a stratified flow. Combustion and Flame, 145(3), 621–634. https://doi.org/10.1016/j.combustflame.2005.12.001
- Gersen, S., Anikin, N. B., Mokhov, A. V., & Levinsky, H. B. (2008). Ignition properties of methane/hydrogen mixtures in a rapid compression machine. International Journal of Hydrogen Energy, 33(7), 1957–1964. https://doi.org/10.1016/j.ijhydene.2008.01.017
- Göttgens, J., Mauss, F., & Peters, N. (1992). Analytic approximations of burning velocities and flame thicknesses of lean hydrogen, methane, ethylene, ethane, acetylene, and propane flames. Symp Combust, 24, 129–135. https://doi.org/10.1016/S0082-0784(06)80020-2
- Guo, H., Smallwood, G. J., Liu, F., Ju, Y., & Gülder, ÖL. (2005). The effect of hydrogen addition on flammability limit and NO emission in ultra-lean counterflow CH4/air premixed flames. Proceedings of the Combustion Institute, 30(1), 303–311. https://doi.org/10.1016/j.proci.2004.08.177
- Haque, M. A., Nemitallah, M. A., Abdelhafez, A., Mansir, I. B., & Habib, M. A. (2020). Review of fuel/oxidizer-flexible combustion in gas turbines. Energy & Fuels, 34(9), 10459–10485. https://doi.org/10.1021/acs.energyfuels.0c02097
- Haque, M. A., Nemitallah, M. A., Abdelhafez, A., Mokheimer, E., & Habib, M. A. (2022). Analysis of methane, propane, and syngas oxy-flames in a fuel-flex gas turbine combustor for carbon capture. International Journal of Energy Research, 46(7), 8657–8675. https://doi.org/10.1002/er.7745
- Imteyaz, B., Habib, M., Nemitallah, M., Abdelhafez, A., & Ben-Mansour, R. (2020). Operability of a premixed combustor holding hydrogen-enriched oxy-methane flames: An experimental and numerical study. International Journal of Energy Research, 2020, 1–15. https://doi.org/10.1002/er.5998
- Johansson, R., Leckner, B., Andersson, K., & Johnsson, F. (2011). Account for variations in the H2O to CO2 molar ratio when modelling gaseous radiative heat transfer with the weighted-sum-of-grey-gases model. Combustion and Flame, 158(5), 893–901. https://doi.org/10.1016/j.combustflame.2011.02.001
- Joshi, N. D., Epstein, M. J., Durlak, S., Marakovits, S., & Sabla, P. E. (1994). Development of a fuel air premixer for aeroderivative dry low emissions combustors. Proc ASME Turbo Expo, 3. https://doi.org/10.1115/94-GT-253
- Kang, T., & Kyritsis, D. C. (2005). Methane flame propagation in compositionally stratified gases. Combustion Science and Technology, 177(11), 2191–2210. https://doi.org/10.1080/00102200500240836
- Kang, T., & Kyritsis, D. C. (2009). Phenomenology of methane flame propagation into compositionally stratified, gradually richer mixtures. Proceedings of the Combustion Institute, 32(1), 979–985. https://doi.org/10.1016/j.proci.2008.06.007
- Kiameh, P. (2003). Power generation handbook: Selection, applications, operation, and maintenance. McGraw-Hill Handbooks.
- Kim, D. (2019). Review on the development trend of hydrogen Gas turbine combustion technology. J Korean Soc Combust, 24, 1–10.
- Kim, H. S., Arghode, V. K., & Gupta, A. K. (2009). Flame characteristics of hydrogen-enriched methane-air premixed swirling flames. International Journal of Hydrogen Energy, 34(2), 1063–1073. https://doi.org/10.1016/j.ijhydene.2008.10.035
- Kim, K. T., & Hochgreb, S. (2011). The nonlinear heat release response of stratified lean-premixed flames to acoustic velocity oscillations. Combustion and Flame, 158(12), 2482–2499. https://doi.org/10.1016/j.combustflame.2011.05.016
- Kim, T. Y., Park, C., Oh, S., & Cho, G. (2016). The effects of stratified lean combustion and exhaust gas recirculation on combustion and emission characteristics of an LPG direct injection engine. Energy, 115, 386–396. https://doi.org/10.1016/j.energy.2016.09.025
- Li, Z., Cheng, X., Wei, W., Qiu, L., & Wu, H. (2017). Effects of hydrogen addition on laminar flame speeds of methane, ethane and propane: Experimental and numerical analysis. International Journal of Hydrogen Energy, 42(38), 24055–24066. https://doi.org/10.1016/j.ijhydene.2017.07.190
- Miao, J., Leung, C. W., Huang, Z., Cheung, C. S., Yu, H., & Xie, Y. (2014). Laminar burning velocities, Markstein lengths, and flame thickness of liquefied petroleum gas with hydrogen enrichment. International Journal of Hydrogen Energy, 39(24), 13020–13030. https://doi.org/10.1016/j.ijhydene.2014.06.087
- Mousavi, S., Kamali, R., Sotoudeh, F., Karimi, N., & Jeung, S. (2020). Numerical investigation of the effects of swirling Hot Co-flow on MILD combustion of a hydrogen–methane blend. Journal of Energy Resources Technology, 142(11), 112301. https://doi.org/10.1115/1.4047251
- Muller, U., Bollig, M., & Peters, N. (1997). Approximations for burning velocities and Markstein numbers for lean hydrocarbon and methanol flames. Combustion and Flame, 108(3), 349–356. https://doi.org/10.1016/S0010-2180(96)00110-1
- Najafi, B., Haghighatshoar, F., Ardabili, S., S. Band, S., Chau, K. w., & Mosavi, A. (2021). Effects of low-level hydroxy as a gaseous additive on performance and emission characteristics of a dual fuel diesel engine fueled by diesel/biodiesel blends. Engineering Applications of Computational Fluid Mechanics, 15(1), 236–250. https://doi.org/10.1080/19942060.2021.1871960
- Nemitallah, M., Haque, A., Hussain, M., Abdelhafez, A., & Habib, M. (2022). Stratified and hydrogen combustion techniques for higher turndown and lower emissions in Gas turbines. Journal of Energy Resources Technology, 144(2), 020801. https://doi.org/10.1115/1.4052541
- Nemitallah, M. A., Haque, M. A., Hussain, M., Abdelhafez, A., & Habib, M. A. (2021). Stratified and hydrogen combustion techniques for higher turndown and lower emissions in gas turbines. Journal of Energy Resources Technology, 2021, 1–42. https://doi.org/10.1115/1.4052541
- Nemitallah, M. A., Imteyaz, B., Abdelhafez, A., & Habib, M. A. (2019). Experimental and computational study on stability characteristics of hydrogen-enriched oxy-methane premixed flames. Applied Energy, 250, 433–443. https://doi.org/10.1016/j.apenergy.2019.05.087
- Nogenmyr, K.-J., Petersson, P., Bai, X. S., Fureby, C., Collin, R., Lantz, A., Linne, M., & Aldén, M. (2011). Structure and stabilization mechanism of a stratified premixed low swirl flame. Proceedings of the Combustion Institute, 33(1), 1567–1574. https://doi.org/10.1016/j.proci.2010.06.011
- Okafor, E. C., Nagano, Y., & Kitagawa, T. (2016). Experimental and theoretical analysis of cellular instability in lean H 2 -CH 4 -air flames at elevated pressures. international Journal of Hydrogen Energy, 41(15), 6581–6592. https://doi.org/10.1016/j.ijhydene.2016.02.151
- Pasquier, N., Lecordier, B., Trinité, M., & Cessou, A. (2007). An experimental investigation of flame propagation through a turbulent stratified mixture. Proceedings of the Combustion Institute, 31, 1567–1574. https://doi.org/10.1016/j.proci.2006.07.118
- Peng, C., Tong, L., & Cao, X. (2016). Numerical analysis on hydrogen stratification and post-inerting of hydrogen risk. Annals of Nuclear Energy, 94, 451–460. https://doi.org/10.1016/j.anucene.2016.04.029
- Porter, R., Liu, F., Pourkashanian, M., Williams, A., & Smith, D. (2010). Evaluation of solution methods for radiative heat transfer in gaseous oxy-fuel combustion environments. Journal of Quantitative Spectroscopy and Radiative Transfer, 111(14), 2084–2094. https://doi.org/10.1016/j.jqsrt.2010.04.028
- Ritchie, H., & Roser, M. (2020). CO2 and GHG emissions. Our World Data. https://ourworldindata.org/emissions-by-fuel.
- Roy, R., & Gupta, A. (2022). Measurement of lean blowoff limits in swirl-stabilized distributed combustion with varying heat release intensities. Journal of Energy Resources Technology, 144(8), 082301. https://doi.org/10.1115/1.4052795
- Salem, E. K. I. J. (2018). Numerical simulations of premixed flames of multi component fuels/air mixture and their applications. University of Kentucky. https://doi.org/10.13023/etd.2019.113.
- Samuelsen, S., & Burn, R. (2006). Quick- Mix, lean burn (RQL) combustor. Gas Turbine Handb., n.d., pp. 227–33.
- Smith, T. F., Shen, Z. F., & Friedman, J. N. (1982). Evaluation of coefficients for the weighted Sum of gray gases model. Journal of Heat Transfer, 104(4), 602–608. https://doi.org/10.1115/1.3245174
- Strakey, P., Sidwell, T., & Ontko, J. (2007). Investigation of the effects of hydrogen addition on lean extinction in a swirl stabilized combustor. Proceedings of the Combustion Institute, 31(2), 3173–3180. https://doi.org/10.1016/j.proci.2006.07.077
- Sui, C., Zhang, J., Zhang, L., Hu, X., & Zhang, B. (2021). Large eddy simulation of premixed hydrogen-rich gas turbine combustion based on reduced reaction mechanisms. Engineering Applications of Computational Fluid Mechanics, 15(1), 798–814. https://doi.org/10.1080/19942060.2021.1918581
- Tang, A., Xu, Y., Pan, J., Yang, W., Jiang, D., & Lu, Q. (2015). Combustion characteristics and performance evaluation of premixed methane/air with hydrogen addition in a micro-planar combustor. Chemical Engineering Science, 131, 235–242. https://doi.org/10.1016/j.ces.2015.03.030
- Wu, F., Kelley, A. P., Zhu, D., & Law, C. K. (2011). Further study on effects of hydrogen addition on laminar flame speeds of fuel-air mixtures US National Combustion Meeting Atlanta Ga.
- Yu, G., Law, C. K., & Wu, C. K. (1986). Laminar flame speeds of hydrocarbon + air mixtures with hydrogen addition. Combustion and Flame, 63(3), 339–347. https://doi.org/10.1016/0010-2180(86)90003-9
- Yuan, J., Ju, Y., & Law, C. K. (2007). On flame-front instability at elevated pressures. Proceedings of the Combustion Institute, 31(1), 1267–1274. https://doi.org/10.1016/j.proci.2006.07.180
- Zimont, V., Polifke, W., Bettelini, M., & Weisenstein, W. (1997). An efficient computational model for premixed turbulent combustion at high reynolds numbers based on a turbulent flame speed closure. Vol. 2 Coal, biomass altern. Fuels; combust. Fuels; Oil Gas appl. Cycle innov. American Society of Mechanical Engineers.
- Zimont, V. L. (1977). To computations of turbulent combustion of partially premixed gases, chemical physics of combustion and explosion processes. In Combustion of multi-phase and gas systems (pp. 77–80). OIKhF.
- Zimont, V. L., & Lipatnikov, A. N. (1992). A model of heat release in premixed turbulent combustion. In T. V. Ondranin (Ed.), Applied problems of aeromechanics and geospase physics (pp. 48–58). MIPT.