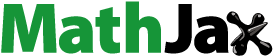
Abstract
Efficient energy conversion can improve Pelton turbine unit efficiency and bring higher economic benefits. However, the reasons that influence the energy conversion characteristics of the Pelton turbine have not been effectively revealed. The main reason is the lack of systematic research on the change process of flow patterns in the bucket and its relationship with the energy conversion characteristics. Experimental and numerical simulation studies of a Pelton turbine were conducted. The change process of the flow patterns from when the jet enters the bucket to when it is completely discharged is analyzed and three main flow patterns in the buckets are summarized. Through energy characteristic analysis, it was found that during the power change process of the bucket, the flow rate entering the bucket and the velocity moment at the inlet of the bucket shows a trend of first increasing and then decreasing, while the velocity moment at the outlet of the bucket and flow loss show an opposite trend, resulting in the most sufficient energy conversion when the jet is perpendicular to the bucket. The basic topology of the bucket energy conversion process is constructed to effectively understand the Pelton turbine's energy conversion characteristics.
Nomenclature
Rα | = | volume fraction |
Vm | = | velocity of the mixture |
Μm | = | dynamic viscosity of the mixture |
Μt | = | turbulent viscosity |
F1 | = | weighted function |
Q | = | flow rate |
Pr | = | power |
= | evaluation parameter | |
= | grid convergence index | |
Wd | = | bucket width |
Q11 | = | unit flow rate |
Ηopt | = | optimal efficiency |
T | = | time |
R | = | radial vector |
N | = | wall-normal phase vector direction |
= | rotational angular speed of the runner | |
Eout | = | total energy at the outlet of the bucket |
Hf | = | flow loss |
Ep | = | effective energy |
Cu | = | circumferential component of absolute velocity |
Sa | = | average entropy production rate |
Tw | = | temperature |
W1 | = | relative velocity of bucket inlet |
Cm1 | = | axial velocity of bucket inlet |
Β2 | = | relative flow Angle of bucket outlet |
Α2 | = | absolute flow Angle of bucket outlet |
V2 | = | absolute velocity of bucket outlet |
U2 | = | circumferential velocity of bucket outlet |
Pd | = | density of power |
Ρm | = | density of the mixture |
Pm | = | static pressure of the mixture |
Aαβ | = | interfacial area density |
Gk | = | generating term of turbulent kinetic energy |
A1 | = | constant coefficient |
= | rigid vorticity | |
= | specific dissipation rate | |
= | relative error of the extrapolated value | |
Sn | = | nozzle opening |
H | = | head |
Η | = | efficiency |
Ns | = | specific speed |
A | = | bucket area |
Τ | = | wall stress |
K | = | rotation axis direction |
Ein | = | total energy entering the bucket |
= | unit mass flow rate | |
Cur | = | velocity moment |
= | absolute flow angle | |
Sf | = | local entropy production rate |
QT | = | total water mass flow |
Γ | = | angle of incidence |
W2 | = | relative velocity of bucket outlet |
Cm2 | = | axial velocity of bucket outlet |
Β1 | = | relative flow Angle of bucket inlet |
Α1 | = | absolute flow Angle of bucket inlet |
V1 | = | absolute velocity of bucket inlet |
U1 | = | circumferential velocity of bucket inlet |
Abbreviations
VOF | = | volume of fluid |
IEC | = | international electrotechnical commission |
GCI | = | grid convergence index |
SST | = | shear stress transport |
1. Introduction
Global industrialization has led to a dramatic increase in the demand for energy in developing countries (Rejeb et al., Citation2022). This resulted in an approximate 5% rise in carbon dioxide emissions in 2021, challenging global climate security (Mitali et al., Citation2022). Renewable green energy is pollution-free and the first choice to alleviate the increasingly severe global climatic problems (Qu et al., Citation2023; Zhao et al., Citation2022). Hence, it is necessary to develop green and clean energy. Hydropower is a widely used form of green energy. In 2021, the global installed hydropower capacity reached 1330 GW, and by 2050, it should be approximately 60% to meet the growing energy demand (Quaranta & Trivedi, Citation2021). Water turbine power generation is the most widely used form of hydropower generation because the load can be easily adjusted by changing the water flow through the turbine. Turbine power generation systems can reduce the demand for power supply from slow response coal-fired and nuclear power systems (Bhattarai et al., Citation2019).
The Pelton turbine is a type of widely used hydraulic turbine in which the runner of the turbine unit works under atmospheric pressure. Therefore, the unit flow rate and load can be adjusted by adjusting the number of operating nozzles and nozzle openings. Compared with a reaction turbine, it has a high application head, a wide applicable discharge range, and a high-efficiency load range (Sengpanich et al., Citation2019). When Pelton turbines are used to develop ultra-high water head resources, they can significantly save the power station investment. At present, the design head of Switzerland's Bieudron Power Station is 1869 m, and the rated output of a single unit is 423 MW, whereas that of Sichuan Subagu Hydropower Station, with the highest applied head in China, is 1175 m, and the rated output of a single unit is only 26 MW (Zeng, Citation2018). Pelton turbines account for 16% of the turbines currently installed in the European Union. In Europe, 31% of hydropower stations with more than 50 MW capacity are Pelton turbines because they have comprehensive applications and development prospects (Quaranta et al., Citation2020).
Unlike the reaction-type hydroelectric generating unit, the Pelton turbine mainly uses a high-speed water jet to drive the runner to rotate (Panagiotopoulos et al., Citation2015). They face many complex engineering problems (Ge et al., Citation2021; Kumar & Saini, Citation2010; Messa et al., Citation2019; Stamatelos et al., Citation2011), including sediment wear, cavitation caused by high-speed jets, flow-induced vibration, and energy conversion characteristics. Among them, energy conversion characteristics are the focus of the current research because efficient energy conversion can improve unit efficiency (Vessaz et al., Citation2017), which brings higher economic benefits. At present, several studies have been carried out on the energy evolution characteristics of Pelton turbines. Zeng et al. (Citation2017) researched the flow characteristics of these turbines and found that the bucket's energy conversion changes periodically, and different buckets’ energy characteristic curves show a specific phase difference, leading to periodic changes in the efficiency of their units. Nigussie et al. (Citation2017) analyzed that the regular shift in the bucket's energy characteristic curve is due to the change of jet flow entering the bucket. Xiao (Citation2006) conducted numerical calculations of the Pelton turbine and discovered the impact of the jet on the diffusion and development of water film in the bucket. This leads to changes in its energy conversion efficiency, which is because of the different development rules of the water film that happens when the relative position of the jet and the bucket changes. Kubota (Citation1989) was the first to analyze the bucket's flow interference phenomenon through experimental visualization research. The jet interference at the inlet and outlet of the bucket sharply declines the Pelton turbine model efficiency. Zeng (Citation2018) pointed out that when the bucket cuts the jet, it reduces the bucket's working capacity and hinders its rotation, thus reducing the energy conversion efficiency. Perrig et al. (Citation2006) conducted numerical simulation and experimental research on the Pelton turbine and found that the bucket area near the cutout most significantly contributes power to the runner. These areas had longer interactions with the jet and received high-momentum water particles. Xiao et al. (Citation2014) analyzed the bucket's flow characteristics at different moments. They found gradual changes in the torque of the runner with a shift in the bucket's position and different torque curves at different radial positions. Based on theoretical derivation, Zhang (Citation2007) discovered changes in centrifugal and Coriolis forces as important reasons for the change in the working capacity of the bucket. Petley (Citation2018) preliminarily carried out a velocity triangle analysis of the bucket's flow characteristics and found the water hitting the bucket to deviate from the original flow direction. It moved along the internal curvature of the bucket in a forced trajectory and inevitably led to the deflection of the outflow angle, resulting in energy characteristic changes.
In general, research on the Pelton turbine bucket's flow and energy characteristics has revealed many valuable results; however, these are still insufficient to clearly understand the energy conversion mechanism of the Pelton turbine. The above research independently analyzes the change in the bucket's flow and energy conversion characteristics. The correlation between the bucket's flow patterns and energy conversion characteristics has not been systematically summarized; therefore, the conversion process of energy performance is unrecognized. In addition, the reasons behind this correlation have not been effectively revealed, which is not conducive to understanding the essence behind the bucket's flow phenomenon. The purpose of this study is to achieve the following two sub-objectives: (1) Summarize the relationship between the bucket's flow patterns and energy conversion. (2) Reveal the reasons behind the relationship between the bucket's flow patterns and energy conversion.
2. Mathematical models and methods
2.1. Multiphase flow models
Unlike the reaction turbine runner, which is completely submerged in water, the Pelton turbine runner is connected to the atmosphere, as shown in Figure (Chitrakar et al., Citation2020; Petley, Citation2018). That is to say, under the action of the jet from the nozzle, there are both water and air phases in the runner, as shown in Figure (Avellan et al., Citation1998). Therefore, to consider the impact of air – water two phases flow, the current flow simulation in the Pelton turbines is based on water air two-phase flow (Bhattarai et al., Citation2019).
Figure 1. Structural diagram of Pelton turbine (Petley, Citation2018).

Figure 2. Air-water two phases flow in the Pelton turbine (Avellan et al., Citation1998): (a)nozzle jet (b)air-water two phases flow.

The homogeneous model under the Euler – Euler framework is a standard method to deal with such flow problems. In the homogeneous model, all fluids share the same velocity field, pressure field, and turbulence characteristics where the inter-phase velocity slip is not considered (Wang et al., Citation2022). Compared with the inhomogeneous model, the homogeneous model deals with the multiphase flow of the Pelton turbine more robustly (Židonis & Aggidis, Citation2016). A relatively lower calculation cost helps the homogeneous model to obtain calculation accuracy close to the experiment. The volume fraction control equation and the mass and momentum conservation equation under the Euler – Euler framework are shown in Equations. (1) and (2).
(1)
(1)
(2)
(2) where, for air–water two-phase flow, Np = 2, rα is the volume fraction, α = 1 is the water phase, α = 2 is the air phase, ρm is the density of the mixture, t is the time coordinate, Vm is the velocity vector of the mixture, pm is the static pressure of the mixture, and μm is the dynamic viscosity of the mixture.
In addition, the internal flow in the turbine is a typical free surface flow (Guo et al., Citation2020; Janßen & Krafczyk, Citation2011). The free surface model is recommended by CFX software to simulate the behavior of air–water two-phase with an obvious interface, which is similar to the VOF (volume of fluid) method (ANSYS Inc, Citation2021b). The interface data transfer of momentum and mass in the free surface model directly depends on the contact area between the two phases in flow simulation. It is characterized by the interface area per unit volume between phases, which is known as the interface area density. If the simulation has just two phases, then Equation (3) is used for interfacial area density.
(3)
(3)
2.2. Turbulence models and governing equations
Flow simulation of the Pelton turbine was conducted using ANSYS CFX software. The SST (shear stress transport) k-ω model was selected as the turbulence model for the subsequent flow field calculation, considering that the Pelton turbine bucket's flow field has natural flow separation characteristics (Benzon et al., Citation2015; Hahn et al., Citation2022; Shadab et al., Citation2022; Sun et al., Citation2023). This method shows excellent prediction accuracy on the flow simulation research of related Pelton turbines, and the transport equations of the selected model are shown in Equation (4). In addition, under some special operating conditions, cavitation may exist in the Pelton turbine. When considering cavitation, the flow simulation of Pelton turbines will become more complex, involving the interactions between multiphase systems such as continuous phase water and air, as well as discrete phase bubbles. In this case, the energy conversion characteristics of the jet to the bucket may be more complex. However, this manuscript did not consider cavitation. Because, the purpose and scope of this study are to investigate the impact of jet flow on the energy conversion characteristics of the bucket under conventional operating conditions (without cavitation).
(4)
(4) where Vj is the velocity component, μt is turbulent viscosity, Gk is the generating term of turbulent kinetic energy, F1 is a weighted function, a1 is a constant coefficient, and σk, σω, σω2, α’, β1, and β’ are the closure parameters (Menter, Citation1994).
3. Verification and validation
3.1. Computing domain and boundary conditions
The real view of the Pelton turbine is shown in Figure (a), mainly including the nozzle and runner. Enlarged figures of the nozzle and bucket are shown in Figure (b) and (c) for a clearer understanding of the geometric characteristics of each component. The high computational cost caused by complex multiphase flow calculation and the enormous computational grid in the Pelton turbine is an issue in the construction of the numerical simulation calculation domain. Due to the symmetry of the internal flow of the Pelton turbine, the calculation domain is usually simplified to reduce the calculation cost.
Figure 3. Real views of Pelton turbine and of zoom figures each component: (a) Pelton turbine (b) zoom figure of nozzle (c) zoom figure of bucket.

In accordance with the research of Zeng et al. (Citation2017) this study chooses half of the Pelton turbine area (symmetry boundary) for subsequent flow calculation. The numerical simulation calculation domain is shown in Figure , including the intake, nozzle, bucket, and outlet extension domains. The radial and spanwise extensions of the outlet extension domain stabilize the flow and converge the calculation results. A combination of the inlet total pressure (normal to boundary condition) and outlet opening boundary (normal to boundary condition) is selected for boundary conditions. The turbulence intensity at both the inlet and outlet is 5% (medium intensity). This combination restores the difference between upstream and downstream water levels in actual projects. Under the initial conditions, the water phase volume fraction at the inlet of the calculation domain is one, and the air phase volume fraction is zero. The volume fraction of the air phase at the outlet of the calculation domain is one, and the water phase volume fraction is zero. Židonis (Citation2015) discovered that gravity and surface tension have little influence on the internal flow of the Pelton turbine (about 0.03% error); hence, the impact of these two factors is not considered in this study. Since the relative position of the jet and the bucket changes with the rotation of the runner, the bucket's flow patterns also change at all times. Therefore, a transient calculation is required for the numerical calculation of the Pelton turbine. The time step selected is a 0.25 degree rotation of the runner and a root mean square value of the Courant number which is less than 3; this satisfies the requirement of time step independence (Židonis, Citation2015). The transient rotor-stator method is adopted between rotating and stationary parts No slip condition is used on the wall and the residual convergence standard is 1.0 × 10−4.
3.2. Grid generation and independence verification
Mesh discretization is an essential part of numerical simulation. Due to the complex geometry of the bucket and outlet extension, a tetrahedral grid is used to discretize the calculational domain. The intake and nozzle domains are discretized by a hexahedral grid. The GCI (grid convergence index) method is used for grid independence analysis. According to the requirements of the grid convergence index method (Celik et al., Citation2008; Hahn et al., Citation2023; Zhao et al., Citation2021), three groups of grids are determined. The number of grids is 15112588 (case 1), 6807479 (case 2), and 3080295 (case 3), respectively. The average rigid vorticity ωR (Chen et al., Citation2022; Liu et al., Citation2018) of the bucket domain, the average specific dissipation rate ωs of the outlet and power Pr, and the flow rate Q are selected as indices of the grid independence test. Table shows the results of the grid independence analysis. φ1∼φ3 is the numerical simulation results of the evaluation parameters. The relative error of the extrapolated value ranges from 0.061% to 2.69% and
ranges from 0.40% to 6.73%. The grid convergence index
ranges from 0.076% to 3.46% and
ranges from 0.5% to 10.13%. Therefore, the above grid generation scheme meets the requirements of grid convergence (Celik et al., Citation2008; Zhao et al., Citation2021). After considering the calculation cost and accuracy, case 2 grid is finally selected for subsequent flow simulation. The mesh discretization results of the main components of case 2 and zoom figures to the critical mesh parts of the nozzle and bucket are shown in Figures (a)(b), respectively. The average cell size is 1.2mm2. In addition, the distribution of the bucket boundary layer grids (10 layers) is shown in Figure (c) and the distribution of bucket y + at the moment (most areas of bucket y + are below 10) when the jet is perpendicular to the bucket are shown in Figure (d). The numerical discretization schemes of each flow passage component are shown in Table .
Figure 5. Mesh discretization results in the computational domain: (a) nozzle grid discrete (b) bucket grid discrete (c) bucket boundary layer grids (d) bucket y+ distribution.

Table 1. Spatial discrete error evaluation results.
Table 2. Grid type and final number of grids for each domain.
3.3. Experimental validation
The Pelton turbine unit performance test was carried out on a hydraulic machinery test bench conforming to the IEC (International Electrotechnical Commission)−60193 standard. As shown in Figure (a), the test was conducted on the high-precision test bench of Harbin Electric Machinery Company Limited, which provides accurate verification for the numerical simulation results of this study. The head measurement position is the inlet of the inlet pipe and the experimental head is 70 m. The number of nozzles of the Pelton turbine in the experiment is 6. Due to the corresponding simplification of numerical simulation, the total output power is 12 times the simulation result. Also, the pitch diameter of the bucket is 400 mm, and the width of the bucket is 108.04 mm, as shown in Figure (b). The layout diagram of nozzle and the detailed drawings of the nozzle and needle in the experiment are shown in Figures (c)(d), respectively. The layout position of experimental pressure sensors in the experiment is shown in Figure (e). In addition, the total uncertainty of the experimental device is ±0.263%. The static pressure value is measured by a pressure transmitter with an uncertainty of ±0.10%. The uncertainty of using an electromagnetic force flowmeter to measure the flow value is within ±0.2%. Using a torque meter to measure the torque value and obtain the power, the uncertainty is within ±0.10%.
Figure 6. Pelton turbine test bench: (a) overall diagram of the experimental setup (b) Pelton turbine bucket (c) layout diagram of nozzle (d) nozzle and needle (e) layout position of experimental pressure sensors.

The comparisons between numerical simulation and experimental results under different nozzle openings are shown in Figure . The schematic diagram of the nozzle opening is shown in Figure . The formula for nozzle opening Sn is shown in Equation 5. As shown in Figure , the unit flow rate Q11 (Equation 6) and efficiency coefficient ηc (Equation 7) calculated by numerical simulation under different nozzle openings are compared with the experimental results. The analysis results indicate that the relative errors between the unit flow rate Q11 obtained from numerical simulation under different nozzle openings and the experimental results are within ±7% and ±8%, respectively. Therefore, the results obtained by the numerical simulation scheme reflect the actual situation relatively accurately, laying a foundation for the accuracy of analysis of the subsequent results.
(5)
(5)
(6)
(6) Where Smax is the maximum position that the needle tip can reach, and ΔS is the distance from the position of the needle tip to the maximum position that it can reach. Q is the flow rate, Wd is the bucket width, and H is the experimental head.
4. Evolution process of bucket energy conversion
Power is an important variable reflecting the bucket energy, representing the change in the bucket's working capacity. Figure (a) presents the power curves of different buckets over time. With the evolution of time, the power of different buckets shows a periodic change trend. That is, the power first increases to the peak value and then continues to decrease to 0. The distance between the peak points of different bucket power curves is approximately 3.34 × 10−3s, which is about 1/21 of the time when the runner rotates for one cycle (21 is the number of buckets). Analysis of the power change characteristics of one bucket helps to understand its energy conversion characteristics because the power of the bucket changes periodically. Nine moments of a bucket cycle are selected for further analysis (T0-T8), as shown in Figure (b). The T4 moment is the peak point of the power curve.
Figure 9. Power characteristics of the bucket: (a) bucket power variation curve (b) delection of typical moments.

Here, we provide additional explanations on the magnitude of the power value. In fact, the Pelton turbine unit studied in this manuscript is a model unit, not a real unit. The pitch diameter of the runner of the Pelton turbine studied in this manuscript is only 400 mm, as shown in Figure (b). That is to say, the size of the Pelton turbine we studied is very small, which is the first reason for the low power value. Secondly, the initial design requirement for the Pelton turbine was that the specific speed ns (Equation 7) of a single nozzle should be around 16.8. In equation 8, n is the rotational speed of 847.2r/min, Pr is the power, and H is the head. The head of the experiment is 70 m. The power of a single bucket can be obtained to be approximately 16000w, which is consistent with the calculated value [Figure (a)] in the manuscript.
(7)
(7) Figure demonstrates the relative position of the jet and the target bucket in a working periodic. Figure presents the result of the angle between the nozzle jet centerline and the bucket splitter (we define this angle as the incidence angle) within a working period of the bucket. The jet just enters the bucket at the T0 moment when the incident angle is small and the power of the bucket is 0. With the rotation of the runner, the incident angle gradually increases and the power of the bucket rapidly increases. When the incident angle is 90 degrees (T4), the bucket's power is maximum. As the incident angle increases, the bucket's power begins to show a downward trend. At the T8 moment, the power of the bucket is 0, the bucket splitter tip almost reaches the center line of the nozzle jet, and the target bucket no longer gets filled with water. It is necessary to recognize its basic flow pattern to understand the reason for this trend in bucket power with a change in the incident angle.
Figure 10. Schematic diagram of the relative position of the jet and the bucket: (a) T0 (b) T2 (c) T4 (d) T6 (e) T8.

The power of the target bucket at T0 and T8 is 0, and hence, the streamline distribution is insignificant. Therefore, only Figures (a)(c)(e)(g)(i)(k)(m) demonstrate the streamline of the target bucket near the extreme position of the wall and the contour map of the water phase volume fraction at T1∼T7 moments. When the jet first enters the bucket (T1), it is accompanied by a higher flow velocity, and as time passes (T2∼T3), the range of the high-velocity area gradually increases. When the jet flow is perpendicular to the bucket splitter (T4), the high-velocity area is mainly concentrated near the bucket outlet edge, and with further increase of the incident angle (T5∼T7), the range of the high-velocity area gradually begins to decrease. From T1 to T3 moments, the main flow pattern is from the tip of the bucket splitter, whereas from T5 to T7 moments, it is from the root of the bucket splitter that obliquely flows out in both cases. The above two flow patterns make the flowing stroke of water in the bucket too long. The flow pattern at the T4 moment is the transition state between T1∼T3 and T5∼T7. At this time, the main pattern presents a vertical inflow from the bucket splitter and flows out along the tangential direction of the outlet edge of the bucket. This is the ideal flow pattern that almost follows the geometric contour of the bucket. The main flow patterns are similar, and hence, at T1∼T3, it is defined as flow pattern one, T4 as flow pattern two (transition), and T5∼T7 as flow pattern three. Figures (b)(d)(f)(h)(j)(l)(n) illustrate the cloud chart of the change of bucket power with time, which can effectively reflect the change in the bucket's working capacity. The power calculation formula is given in equation (8), where A is the bucket area, r is the radial vector, τ is the wall stress (including normal stress and shear stress), N is the wall-normal phase vector direction, k is the rotation axis direction, and ω is the rotational angular speed of the runner. The final obtained power value is the integral result of Equation (8).
(8)
(8) Figures (b)(d)(f)(h)(j)(l)(n) demonstrate that when the jet just enters the bucket (T1), its main working position is concentrated near the tip of the bucket splitter which gradually increases with the continuous rotation of the runner. From T1 to T3, the main working position of the bucket moves from the tip of the bucket splitter to the root of the bucket. As the runner continues to rotate from T5 to T7, the range of the working position gradually decreases, and the main position moves from the root to the tip of the bucket.
Figure 12. Flow characteristics of the bucket and bucket power at different moments: (a) Water velocity at T1 (b) Bucket power at T1 (c) Water velocity at T2 (d) Bucket power at T2 (e) Water velocity at T3 (f) Bucket power at T3 (g) Water velocity at T4 (h) Bucket power at T4 (i) Water velocity at T5 (j) Bucket power at T5 (k) Water velocity at T6 (l) Bucket power at T6 (m) Water velocity at T7 (n) Bucket power at T7.
Figure 13. Schematic diagram of the spanwise and radial direction of the bucket:(a) spanwise and radial direction (b) location of quantitative analysis.

Figure 14. Density of power distribution at different moments: (a) 0.2 times radial position (b) 0.5 times radial position (c) 0.8 times radial position.

To further analyze the variation characteristics of bucket power, the quantitative results of power variation at different radial and span positions at different times are given.
The schematic diagram of the bucket's spanwise and radial positions is shown in Figure . Among them, when the relative radial position is 0, it is the root of the bucket, and when the relative radial position is 1, it is the tip position of the bucket. When the relative spanwise position is 0, it is the bucket splitter, and when the relative spanwise position is 1, it is the outlet edge of the bucket.
Figure illustrates the quantitative results of bucket power at different radial positions at different moments. Abscissa 0 is located at the bucket splitter, and abscissa 1, at the outlet edge. From a radial perspective, the power of the bucket at different moments at 0.5 radial positions is greater than that at other positions, and the power at different moments at 0.8 radial positions is the smallest. Viewed from the spanwise direction, the maximum power at 0.2 and 0.5 times radial positions both appear at 0.35 times spanwise position between the bucket outlet edge and the bucket splitter, to which they are closer. The maximum power at 0.8 times radial position appears at 0.75 times spanwise position between the bucket splitter edge and the bucket outlet edge, to which it is closer. The change of the bucket power over time corresponds to the distribution of bucket streamlines (Figure ), which also indicates the flow pattern change as the main reason for the change of the bucket power. Flow pattern one increases bucket power, whereas flow pattern three reduces it. The specific reason for such changes in the power of the bucket at different flow patterns needs further analysis.
5. Mechanism of bucket energy conversion
Here, the influence of different flow patterns on the bucket's working capacity from the perspective of energy conversion is analyzed. As shown in Figure , assume that the total energy entering the bucket is Ein, the flow loss in the bucket is hf, and the total energy at the outlet of the bucket is Eout. The effective energy Ep converted by the jet impact on the bucket is expressed as the total energy of the bucket inlet Ein minus the bucket outlet energy Eout, and then, minus the flow loss hf. This means that the energy entering the bucket loses a certain amount to obtain the bucket outlet energy. The Euler equation derived based on Newton's second law can get the total energy at the inlet and outlet of the bucket, as shown in Equation (9), where ω is the rotational angular velocity of the runner, which is a constant when the rotational speed is constant. is the mass flow rate, and Cu1r1 and Cu2r2 are the velocity moments at the inlet and outlet of the bucket, respectively. From the perspective of energy conversion, the greater the energy Ein entering the bucket, and the smaller the flow loss hf in the bucket and the energy at the outlet of the bucket Eout, the better. At this time, the effective energy Ep is larger, and the bucket's working capacity is stronger. The main objective of this article is to reveal the reasons for the influence of different flow patterns on the bucket's working capacity. The relationship between them can be established by analyzing the changing laws of the four variables (
, Cu1r1, Cu2r2, hf) that affect the effective energy Ep in the bucket. This is analyzed separately in subsequent sections.
(9)
(9)
Figure 15. Energy conversion process in the bucket: (a) explanation of bucket position (b) energy conversion characteristics.

5.1. Evolution process of the mass flow rate
The change of the mass flow rate in the bucket with time is analyzed. This article uses CFX software to simulate the internal flow of the Pelton turbine. The calculation of mass flow is carried out according to the following formula: Mass flow = areaInt_x (Water. Velocity u * Density)@ Locaion + areaInt_y (Water. Velocity v * Density)@ Locaion + areaInt_z (Water. Velocity w* Density)@ Locaion. Among them, areaInt_ x, areaInt_ y. areaInt_ z refers to the area integral of the bucket in the x, y, and z directions, Water Velocity u, Water Velocity v, Water Velocity w refers to the velocity of water in the x, y, and z directions, while Density refers to the density of water. Figure presents the mass flow rate distribution cloud diagram in the bucket at different moments which has almost the same distribution trend as the power cloud diagram in Figure . When the jet just enters the bucket (T1), its mass flow rate distribution area is mainly concentrated near the tip of the bucket splitter. As time passes, from T1 to T3, the mass flow rate distribution area in the bucket gradually moves from the tip of the bucket splitter to the root of the bucket, expanding the distribution area. As the runner continues to rotate from T5 to T7, the mass flow rate distribution area in the bucket gradually decreases, and it moves from the root to the tip of the bucket. Generally speaking, the closer the incident angle is to 90 degrees, the wider the mass flow rate distribution range in the bucket.
Figure shows the quantitative results of the total mass flow rate in the bucket at different moments. The total mass flow rate in the bucket at different moments showed a trend of first increasing and then decreasing, and the closer the incident angle is to 90 degrees, the greater the mass flow rate in the bucket. The changing trend of the total mass flow rate with the power results at different moments in the bucket [Figure (b)] is similar when compared. The Pearson correlation coefficient between the mass flow rate and the power in the bucket is approximately 0.95, which means that the changing trend between them is strongly correlated.
5.2. Evolution process of velocity moment
Cur is known as the velocity moment in rotating machinery, which reflects the head concept. As aforementioned, the total energy Ein at the inlet of the bucket should be as large as possible, and the smaller the Eout, the better. When comparing the velocity moment, the total energy at the inlet and outlet of the bucket at the same radius is generally compared, so r1 = r2. At this time, the comparison of the velocity moment at the inlet and outlet of the bucket is essentially the comparison of the circumferential component Cu of absolute velocity at the inlet and outlet of the bucket. Therefore, if the total energy Ein at the inlet of the bucket is larger, the angle between absolute and circumferential velocities should be as small as possible. The absolute velocity has a larger component in the direction of the circumferential velocity, and this results in a smaller energy loss on the circumference. The angle between absolute and circumferential velocities at the inlet of the bucket is 0. At this time, the energy conversion efficiency Cu1 of the jet entering the bucket is the highest. When the total energy Eout at the outlet of the bucket is smaller, the angle between absolute and circumferential velocities is larger. Then, the absolute velocity component in the direction of circumferential velocity is smaller and the total energy Eout at the outlet of the bucket is expected to be 0. That is, the absolute velocity is perpendicular to the direction of the circumferential velocity, Cu2 at the outlet of the bucket is 0, and there is no energy loss on the circumference. The above analysis gives a qualitative understanding of the energy conversion characteristics of the bucket, and a more specific comparison of these results is denoted below.
Figure presents the absolute and circumferential velocity vector distributions in the bucket at different times. Figure demonstrates the distribution of quantitative results of the angle between absolute and circumferential velocities (absolute flow angle θ) at different radial positions. The absolute flow angle is solved according to Equation (10), where V and U are the absolute and circumferential velocity vectors, respectively.
(10)
(10) Further analysis in conjunction with Figures and is also presented below. The absolute flow angle at the inlet of the bucket at different radial positions presents a decreasing trend first that increases over time. When the incident angle is 90° (T4), the absolute flow angle at the inlet of the bucket is the smallest, and at the outlet at different radial positions, it shows an increasing trend first that decreases over time, which is the opposite. The closer the incident angle is to 90 degrees, the larger the absolute flow angle at the outlet of the bucket. A comparison of the absolute flow angles at different radial positions reveals that the angle at the inlet of the bucket at 0.5 times the radial position is the smallest (close to 0 degrees) and that at the outlet is the largest (close to 90 degrees) at the T4 moment. This partially explains why the power at 0.5 times radial position in Figure (b) is the largest.
Figure 18. Absolute velocity and circumferential velocity vector distribution: (a) T1 (b) T2 (c) T3 (d) T4 (e) T5 (f) T6 (g) T7.

Figure 19. Absolute flow angle and velocity moment distribution at different radial positions: (a) Absolute flow angle at 0.2 times radial position (b) Velocity moment at 0.2 times radial position (c) Absolute flow angle at 0.5 times radial position (d) Velocity moment at 0.5 times radial position (e) Absolute flow angle at 0.8 times radial position (f) Velocity moment at 0.8 times radial position.

Figure 20. Difference between inlet and outlet velocity moments of buckets at different radial positions.

Figure 21. Average entropy production at different moments: (a) T1; (b) T2; (c) T3; (d) T4; (e) T5; (f) T6; (g) T7.

Figure illustrates the distribution of the difference between the velocity moments at the inlet and outlet of the bucket at different radial positions that can be used to characterize the magnitude of energy conversion in these positions. Figure summarizes two results. First, the main energy conversion position in the bucket is located in the middle and root of the bucket. Second, the range of energy conversion in the bucket increases first and then decreases with time, and the closer the incident angle is to 90 degrees, the more sufficient the energy conversion is.
5.3. Evolution process of hydraulic loss
Entropy production rate is a commonly used variable to measure the loss in hydraulic machinery (Hahn et al., Citation2022; Kan et al., Citation2022). The entropy production rate is composed of two parts: one (average entropy production rate Sa) is the flow loss caused by average velocity, and the other (local entropy production rate Sf) by fluctuating velocity. The expression of the entropy production rate is shown in Equation (11).
(11)
(11) This variable is selected to analyze the flow loss in the bucket at different moments. Under the same scale, the cloud charts of the average and fluctuating entropy production rates at different times are given in Figures and . The average entropy production rate is smaller than the local entropy production rate, which indicates that fluctuating velocity causes flow loss in the bucket. The local entropy production rate can be further understood as the flow loss caused by the unsteady flow in the bucket.
Figure 22. Local entropy production and turbulence kinetic energy at different moments: (a) Local entropy production at T1 (b) Turbulence kinetic energy at T1 (c) Local entropy production at T2 (d) Turbulence kinetic energy at T2 (e) Local entropy production at T3 (f) Turbulence kinetic energy at T3 (g) Local entropy production at T4 (h) Turbulence kinetic energy at T4 (i) Local entropy production at T5 (j) Turbulence kinetic energy at T5 (k) Local entropy production at T6 (l) Turbulence kinetic energy at T6 (m) Local entropy production at T7; (n) Turbulence kinetic energy at T7.
Figures (a)(c)(e)(g)(i)(k)(m) classify the streamline distribution in the bucket at different times, which is dyed by the local entropy production rate. When the jet just enters the bucket, the local entropy production rate (T1) is large, and the flow loss near the bucket splitter and the outlet edge is high. As time passes, the high flow loss area gradually decreases. When the incidence angle is 90 degrees (T4), the local entropy production rate is the minimum. As the runner continues to rotate (T5∼T7), the high flow loss range starts to increase. Except near the outlet edge of the bucket, the high flow loss range at the bucket splitter starts to increase gradually. From another perspective, Figure (b)(d)(f)(h)(j)(l)(n) classify the streamline distribution in the bucket at different times (turbulent kinetic energy dyeing). When the jet just enters the bucket (T1), the flow around the bucket has a longer stroke, and the instability of the flow in the bucket is increased, accompanied by a higher turbulence effect, which leads to a higher flow loss. When the incidence angle is close to 90 degrees (T4), after the jet enters the bucket from the splitter, the flow in the bucket almost follows the bucket profile. At this time, the flow around the bucket is shorter, tends to be more stable, and the flow loss is smaller. From T5∼T7, the jet generally flows from the root to the tip of the bucket. As the outflow stroke of the fluid increases, the turbulent effect of the fluid near the bucket splitter and the flow loss increases accordingly. In general, the distribution of flow loss and turbulent kinetic energy is consistent.
5.4. Topological structure of energy conversion process
This section summarizes the basic topology of flow patterns and energy conversion characteristics. Figure provides a schematic diagram of the relative positions of the jet and the bucket. The flow patterns and energy conversion characteristics are the same from the moment when the jet just enters the bucket (Ts) to the moment when it is perpendicular to the bucket diverter (Tm), and hence, the period from Ts to Tm is defined as stage I. Similarly, the period from the moment Tm to the moment when the jet completely leaves the bucket (Tf) is defined as stage II. The above analysis reveals three typical flow patterns in these two stages: Ts∼Tm (excluding the Tm moment) is flow pattern one, the Tm moment is flow pattern two, and Tm∼Tf (excluding the Tm moment) is flow pattern three.
Figure illustrates the flow patterns and triangular velocity distribution at different stages. From the perspective of the flow pattern, for flow pattern one [Figure (a)], the jet has just entered the bucket, the incident angle is relatively small, and the incident point is close to the tip of the bucket splitter. The pattern now is that the flow enters from the tip of the bucket splitter and flows out obliquely. From the perspective of energy conversion, in stage I, the absolute flow angle at the inlet of the bucket is relatively large. This results in energy loss in the direction of the circumferential velocity of the absolute velocity, and the energy is not effectively utilized. The absolute velocity at the outlet of the bucket still has a certain component in the direction of the circumferential velocity. This results in energy conversion but the remaining energy at the outlet of the bucket is not effectively utilized. In addition, the water flow in stage I has a longer flow path in the bucket, and the turbulent effect is more obvious, resulting in a greater flow loss. Therefore, the energy conversion efficiency is not high in stage I.
Figure 24. Energy conversion law of bucket: (a) flow pattern one and energy conversion characteristics (b) flow pattern two and energy conversion characteristics (c) flow pattern three and energy conversion characteristics.

From the perspective of the flow pattern, for flow pattern two [Figure (b)], the incident angle is close to 90 degrees at this time, and the incident point is close to the middle of the bucket splitter. Hence, the main flow pattern presents a vertical inflow from the bucket splitter and flows out along the tangential direction of the outlet edge of the bucket, which is ideal. From the perspective of energy conversion, for flow pattern two, the absolute flow angle at the inlet of the bucket is close to 0 degrees, and the energy is effectively converted. Whereas at the outlet of the bucket, it is close to 90 degrees, there is almost no residual energy, and the energy utilization is relatively sufficient. In addition, the flow around the bucket is the shortest, the turbulence effect is small, and the flow loss caused is also the smallest. Therefore, the energy conversion efficiency is highest.
As the runner continues to rotate, for flow pattern three [Figure (c)], the incident angle continues to increase, and the incident point moves to the root of the bucket splitter. At this time, the flow pattern presents an inflow from the root of the bucket splitter that obliquely flows out. From the perspective of energy conversion, compared with flow pattern two, the absolute flow angle at the inlet of the bucket increases, resulting in energy loss in the direction of the absolute velocity of the circumferential velocity, and the energy is not effectively utilized. The absolute flow angle at the outlet of the bucket decreases, resulting in energy conversion where the remaining energy is not effectively utilized. In addition, the water flow in flow pattern three has a longer flow path in the bucket, and the turbulent effect is more obvious, resulting in a greater flow loss. Therefore, the energy conversion in this pattern is reduced compared to flow pattern two. This also explains the reason for the power drop in stage II.
6. Conclusions and future work
In this study, numerical simulation studies have been carried out on a Pelton turbine to clarify the flow evolution process in the bucket and its relationship with the energy conversion characteristics. The following conclusions have been drawn:
When the relative position of the jet and the bucket changes, the bucket power exhibits periodic changes. In this evolution process, three main flow patterns in the bucket are summarized and provide general topological structures for different flow patterns.
The characteristics of the three flow patterns are: firstly, the main flow pattern tends to flow in from the bucket splitter tip and then obliquely flows out. Secondly, the main flow pattern is a vertical inflow from the bucket splitter and then flows out along the tangent direction of the outlet edge. Finally, the main flow pattern tends to flow in from the root of the bucket splitter and then obliquely flows out.
From the perspective of energy conversion, the influence laws of different flow patterns on flow rate, velocity moment, and flow loss are systematically constructed. During the power change process of the bucket, the flow rate entering the bucket and the velocity moment at the inlet of the bucket shows a trend of first increasing and then decreasing, while the velocity moment at the outlet of the bucket and flow loss show an opposite trend, resulting in the most sufficient energy conversion when the jet is perpendicular to the bucket.
Disclosure statement
No potential conflict of interest was reported by the author(s).
Additional information
Funding
References
- ANSYS Inc. (2021b). ANSYS CFX-solver theory guide. ANSYS Inc.
- Avellan, F., Dupont, P., Kvicinisky, S., Chapuis, L., Parkinson, E., & Vullioud, G. (1998). Flow calculations in Pelton turbines - part 2: Free surface flows. 19th IAHR symposium on hydraulic machinery and cavitation, Singapore.
- Benzon, D., Židonis, A., Panagiotopoulos, A., Aggidis, G. A., Anagnostopoulos, J. S., & Papantonis, D. E. (2015). Impulse turbine injector design improvement using computational fluid dynamics. Journal of Fluids Engineering, 137(4), 041106. https://doi.org/10.1115/1.4029310
- Bhattarai, S., Vichare, P., Dahal, K., Makky, A. A., & Olabi, A. G. (2019). Novel trends in modelling techniques of Pelton turbine bucket for increased renewable energy production. Renewable and Sustainable Energy Reviews, 112, 87–101. https://doi.org/10.1016/j.rser.2019.05.045
- Celik, I. B., Ghia, U., Roache, P. J., Freitas, C. J., Coleman, H., & Raad, P. E. (2008). Procedure for estimation and reporting of uncertainty due to discretization in CFD applications. Journal of Fluids Engineering, 130, 078001–7. https://doi.org/10.1115/1.2960953
- Chen, S. T., Zhao, W. W., & Wan, D. C. (2022). Turbulent structures and characteristics of flows past a vertical surface-piercing finite circular cylinder. Physics of Fluids, 34(1), 015115. https://doi.org/10.1063/5.0078526
- Chitrakar, S., Solemslie, B. W., Neopane, H. P., & Dahlhaug, O. G. (2020). Review on numerical techniques applied in impulse hydro turbines. Renewable Energy, 159, 843–859. https://doi.org/10.1016/j.renene.2020.06.058
- Ge, X. F., Sun, J., Zhou, Y., Cai, J. G., Zhang, H., Zhang, L., Ding, M. Q., Deng, C. Z., Binama, M., & Zheng, Y. (2021). Experimental and numerical studies on opening and velocity influence on sediment erosion of Pelton turbine buckets. Renewable Energy, 173, 1040–1056. https://doi.org/10.1016/j.renene.2021.04.072
- Guo, B., Xiao, Y. X., Rai, A. K., Zhang, J., & Liang, Q. W. (2020). Sediment-laden flow and erosion modeling in a Pelton turbine injector. Renewable Energy, 162, 30–42. https://doi.org/10.1016/j.renene.2020.08.032
- Hahn, F. J. J., Maly, A., Semlitsch, B., & Bauer, C. (2023). Numerical investigation of Pelton turbine distributor systems with axial inflow. Energies, 16(6), 2737. https://doi.org/10.3390/en16062737
- Hahn, F. J. J., Semlitsch, B., & Bauer, C. (2022). On the numerical assessment of flow losses and secondary flows in Pelton turbine manifolds. IOP conference series: Earth and environmental science, IOP publishing, 1079(1): 012082.
- Janßen, C., & Krafczyk, M. (2011). Free surface flow simulations on GPGPUs using the LBM. Computers & Mathematics with Applications, 61(12), 3549–3356. https://doi.org/10.1016/j.camwa.2011.03.016
- Kan, K., Zhang, Q. Y., Xu, Z., Zheng, Y., Gao, Q., & Shen, L. (2022). Energy loss mechanism due to tip leakage flow of axial flow pump as turbine under various operating conditions. Energy, 255, 124532. https://doi.org/10.1016/j.energy.2022.124532
- Kubota, T. (1989). Observation of jet interference in 6-nozzle Pelton turbine. Journal of Hydraulic Research, 27(6), 753–767. https://doi.org/10.1080/00221688909499107
- Kumar, P., & Saini, R. P. (2010). Study of cavitation in hydro turbines—a review. Renewable and Sustainable Energy Reviews, 14(1), 374–383. https://doi.org/10.1016/j.rser.2009.07.024
- Liu, C. Q., Gao, Y. S., Tian, S. L., & Dong, X. R. (2018). Effects of planar shear on the three-dimensional instability in flow past a circular cylinder. Physics of Fluids, 30(3), 034103. https://doi.org/10.1063/1.5018844
- Menter, F. R. (1994). Two-equation eddy-viscosity turbulence models for engineering applications. AIAA Journal, 32(8), 1598–1605. https://doi.org/10.2514/3.12149
- Messa, G. V., Mandelli, S., & Malavasi, S. (2019). Hydro-abrasive erosion in Pelton turbine injectors: A numerical study. Renewable Energy, 130, 474–488. https://doi.org/10.1016/j.renene.2018.06.064
- Mitali, J., Dhinakaran, S., & Mohamad, A. A. (2022). Energy storage systems: A review. Energy Storage and Saving, 1(3), 166–216. https://doi.org/10.1016/j.enss.2022.07.002
- Nigussie, T., Engeda, A., & Dribssa, E. (2017). Design, modeling, and CFD analysis of a micro hydro Pelton turbine runner: For the case of selected site in Ethiopia. International Journal of Rotating Machinery, 2017, 1. https://doi.org/10.1155/2017/3030217
- Panagiotopoulos, A., Židonis, A., Aggidis, G. A., Anagnostopoulos, J. S., & Papantonis, D. E. (2015). Flow modeling in Pelton turbines by an accurate Eulerian and a fast lagrangian evaluation method. International Journal of Rotating Machinery, 2015, 1. https://doi.org/10.1155/2015/679576
- Perrig, A., Avellan, F., Kueny, J., Farhat, M., & Parkinson, E. (2006). Flow in a Pelton turbine bucket: Numerical and experimental investigations. Journal of Fluids Engineering, 128(2), 350–358. https://doi.org/10.1115/1.2170120
- Petley, S. M. (2018). Numerical and experimental investigation of flow in horizontal axis Pelton turbine [Doctoral dissertation]. Lancaster University.
- Qu, W. J., Gao, Y., He, S., Zhang, J., Peng, K. W., Wu, H. F., Wang, R. L., & Hong, H. (2023). Further study on carbon fixation using green power for a solar-assisted multi-generation system with carbon capture. Energy Conversion and Management, 276, 116574. https://doi.org/10.1016/j.enconman.2022.116574
- Quaranta, E., Bonjean, M., Cuvato, D., Nicolet, C., Dreyer, M., Gaspoz, A., & Tomaselli, G. (2020). Hydropower case study collection: Innovative low head and ecologically improved turbines, hydropower in existing infrastructures, hydropeaking reduction, digitalization and governing systems. Sustainability, 12(21), 8873–8888. https://doi.org/10.3390/su12218873
- Quaranta, E., & Trivedi, C. (2021). The state-of-art of design and research for pelton turbine casing, weight estimation, counterpressure operation and scientific challenges. Heliyon, 7(12), e08527. https://doi.org/10.1016/j.heliyon.2021.e08527
- Rejeb, O., Alirahmi, S. M., Assareh, E., Assad, M., Jemni, A., Bettayeb, M., & Ghenai, C. (2022). Innovative integrated solar powered polygeneration system for green hydrogen, oxygen, electricity, and heat production. Energy Conversion and Management, 269, 116073. https://doi.org/10.1016/j.enconman.2022.116073
- Sengpanich, K., Bohez, E. L. J., Thongkruer, P., & Sakulphan, K. (2019). New mode to operate centrifugal pump as impulse turbine. Renewable Energy, 140, 983–993. https://doi.org/10.1016/j.renene.2019.03.116
- Shadab, M., Karimipour, M., Najafi, A. F., Paydar, R., & Nourbakhsh, S. A. (2022). Effect of impeller shroud trimming on the hydraulic performance of centrifugal pumps with low and medium specific speeds. Engineering Applications of Computational Fluid Mechanics, 16(1), 514–535. https://doi.org/10.1080/19942060.2021.2016492
- Stamatelos, F. G., Anagnostopoulos, J. S., & Papantonis, D. E. (2011). Performance measurements on a Pelton turbine model. Proceedings of the Institution of Mechanical Engineers, Part A: Journal of Power and Energy, 225(3), 351–362. https://doi.org/10.1177/2041296710394260
- Sun, L. G., Li, Y. Y., Guo, P. C., & Xu, Z. F. (2023). Numerical investigation of air admission influence on the precessing vortex rope in a Francis turbine. Engineering Applications of Computational Fluid Mechanics, 17, 1. https://doi.org/10.1080/19942060.2022.2164619
- Vessaz, C., Andolfatto, L., Avellan, F., & Tournier, C. (2017). Toward design optimization of a pelton turbine runner. Structural and Multidisciplinary Optimization, 55(1), 37–51. https://doi.org/10.1007/s00158-016-1465-7
- Wang, C. Y., Wang, F. J., Chen, W. H., He, Q. R., Chen, X., & Zhang, Z. C. (2022). A dynamic particle scale-driven interphase force model for water-sand two-phase flow in hydraulic machinery and systems. International Journal of Heat and Fluid Flow, 95, 108974. https://doi.org/10.1016/j.ijheatfluidflow.2022.108974
- Xiao, Y. X. (2006). Numerical analysis method of unsteady interference for jet and for water sheet [Doctoral dissertation]. South China University of Technology.
- Xiao, Y. X., Wang, Z. W., Zhang, J., Zeng, C. J., & Yan, Z. G. (2014). Numerical and experimental analysis of the hydraulic performance of a prototype pelton turbine. Proceedings of the Institution of Mechanical Engineers, Part A: Journal of Power and Energy, 228(1), 46–55. https://doi.org/10.1177/0957650913506711
- Zeng, C. J. (2018). Research on the Internal flow characteristic and flow interference in the Pelton turbine [Doctoral dissertation]. Tsinghua University.
- Zeng, C. J., Xiao, Y. X., Wang, Z. W., Zhang, J., & Luo, Y. Y. (2017). Numerical analysis of a Pelton bucket free surface sheet flow and dynamic performance affected by operating head. Proceedings of the Institution of Mechanical Engineers, Part A: Journal of Power and Energy, 231(3), 182–196. https://doi.org/10.1177/0957650916689507
- Zhang, Z. H. (2007). Flow interactions in Pelton turbines and the hydraulic efficiency of the turbine system. Proceedings of the Institution of Mechanical Engineers, Part A: Journal of Power and Energy, 221(3), 343–355. https://doi.org/10.1243/09576509JPE294
- Zhao, H. R., Wang, F. J., Wang, C. Y., Chen, W. H., Yao, Z. F., Shi, X. Y., Li, X. Q., & Zhong, Q. (2021). Study on the characteristics of horn-like vortices in an axial flow pump impeller under off-design conditions. Engineering Applications of Computational Fluid Mechanics, 15(1), 1613–1628. https://doi.org/10.1080/19942060.2021.1985615
- Zhao, H. R., Wang, F. J., Wang, C. Y., & Wang, B. H. (2022). Investigation on the hump region generation mechanism of pump mode in low-head pumped hydro-storage unit. Physics of Fluids, 34(11), 115148. https://doi.org/10.1063/5.0130836
- Židonis, A. (2015). Optimisation and efficiency improvement of Pelton hydro turbine using computational fluid dynamics and experimental testing [Doctoral dissertation]. Lancaster University.
- Židonis, A., & Aggidis, G. A. (2016). Pelton turbine: Identifying the optimum number of buckets using CFD. Journal of Hydrodynamics, 28(1), 75–83. https://doi.org/10.1016/S1001-6058(16)60609-1