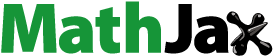
Abstract
Mini solar flat plate collectors have gained traction due to their cost-effectiveness, high efficiency in converting solar radiation to heat energy, and versatility in residential and commercial applications, offering long lifespans with minimal maintenance. Optimizing the thermal performance of solar flat plate collectors using numerical simulations helps enhance their efficiency, making them even more appealing for small-scale heating and hot water applications. This study investigates the flow behavior and temperature distribution of air within a small-sized solar collector (286 × 800 × 70 mm) and optimizes its design for achieving the highest outlet air temperature. The optimization process included varying geometric parameters, such as the wavy structure hole diameter, and considering a range of operating conditions, including ambient conditions and solar intensities. This analysis aimed to understand the flow behavior and temperature distributions within the solar collector. The numerical studies provided valuable insights into the thermal and flow characteristics of the collector. The velocity distribution of the fluid within the collector aided in understanding the flow behavior, optimizing the flow path to minimize pressure drop, and maximizing heat transfer. Additionally, calculations were performed to determine total useful heat, outlet temperatures, and efficiencies. The study also discusses the optimal design and operating conditions for the collector.
Nomenclature
W | = | total heat useful |
m | = | mass flow rate |
Cp | = | specific heat |
dT | = | difference in temperature (To − Ti) |
w | = | total heat |
Isolar | = | solar intensity |
A | = | solar flat plate collector area |
= | efficiency | |
To | = | outlet temperature |
Ti | = | inlet temperature |
1. Introduction
Solar flat plate collectors offer numerous advantages due to their relatively low-cost and simple design. They exhibit high efficiency in converting solar radiation into heat energy, making them an excellent choice for harnessing solar power. Moreover, the versatility of solar flat plate collectors enables effortless integration into a wide range of settings, including both residential and commercial buildings. This inherent adaptability not only amplifies their appeal but also expands their potential applications in various contexts. A mini solar flat plate collector is a compact solar energy device specifically designed for small-scale applications. This versatile equipment harnesses the power of the sun to generate heat, making it ideal for heating water, air, or even confined spaces. In addition to its compact size and efficient design, the mini solar flat plate collector excels in optimizing the utilization of solar radiation, making it a highly effective and sustainable solution for diverse heating needs. By harnessing the power of sunlight, this innovative device offers significant energy savings while minimizing environmental impact. Its versatility allows it to cater to various applications, including heating water, air, or small spaces, providing a reliable and eco-friendly alternative to conventional heating systems. The solar flat plate collector comprises a flat plate constructed from a material with excellent heat absorption properties, such as copper or aluminium. To optimize solar absorption, the plate is coated with a selective coating that enhances its ability to capture solar heat effectively. This coating is specifically designed to maximize the absorption of solar radiation, ensuring efficient heat transfer and conversion within the collector system (Lizama-Tzec et al., Citation2019). The mini solar flat plate collector's design closely resembles that of larger flat plate collectors, featuring a heat-absorbing plate covered with a transparent material like glass or plastic. This transparent cover serves the dual purpose of trapping the captured solar heat within the collector and preventing any undesired heat loss. However, mini solar flat plate collectors are significantly smaller in size, and capacity compared to their larger counterparts, making them ideal for applications with limited space or lower heating demands. Mini solar flat plate collectors find common utilization in small-scale applications, including camping, off-grid cabins, and emergency preparedness. These compact collectors serve the purpose of providing hot water for washing or showering, as well as heating up confined spaces such as tents or small rooms. Their versatility and efficiency make them well-suited for meeting the specific heating needs of these scenarios, where portability and self-sufficiency are paramount. With their ability to harness solar energy, mini solar flat plate collectors offer a sustainable and practical solution for generating heat in diverse small-scale environments.
One of the primary benefits of mini solar flat plate collectors is their portability and user-friendly design. These collectors are lightweight and compact, facilitating effortless transportation and installation (Karki et al., Citation2019). In addition, mini solar flat plate collectors boast a remarkable durability, allowing them to function for numerous years with minimal or virtually no maintenance requirements. Their compact size and lightweight design enable effortless portability, making them highly suitable for deployment in remote areas, including campsites and off-grid locations. Mini solar flat plate collectors offer another notable advantage in terms of their environmental benefits. These devices harness solar energy, diminishing the reliance on fossil fuels and conventional electricity sources. As a result, they contribute to the reduction of greenhouse gas emissions and mitigate air pollution. By utilizing clean and renewable solar power, these collectors actively promote a more sustainable and environmentally friendly energy ecosystem. Moreover, mini solar flat plate collectors find valuable application in remote areas lacking access to conventional energy sources. By being able to function in such environments, they effectively diminish reliance on non-renewable energy sources. This capability further enhances their utility and underscores their role in promoting energy sustainability in areas where traditional power infrastructure is absent or limited. Mini solar flat plate collectors offer an efficient solution for maintaining warmth in cold weather conditions, eliminating the dependence on electricity or fuel. Nevertheless, there are inherent challenges associated with these collectors. A primary concern is their limited capacity due to their compact size. Consequently, they can only generate a restricted amount of hot water or heat, which may prove inadequate for larger households or buildings. It is important to consider the sizing and capacity requirements of the collectors to ensure their suitability for the intended application, particularly in scenarios where greater heating demands are present. Mini solar flat plate collectors are reliant on weather conditions and may not function optimally during cloudy or rainy days. Consequently, their effectiveness is weather-dependent. Despite this limitation, these collectors present a compact and portable solution for small-scale heating and hot water needs. They are user-friendly, eco-friendly, and demand minimal maintenance. Though they possess certain limitations, they hold promise as an attractive option for off-grid living, camping, and emergency preparedness situations.
A wide range of experimental and numerical studies have been conducted to investigate the performance of flat plate solar thermal collectors. These studies have explored various design modifications, such as the utilization of triangle shapes, mini-channel structures, and inner grooved copper tubes. The objective of these investigations has been to optimize the collector's shape and materials to enhance its efficiency. As a result, significant advancements have been made in the development of efficient flat plate collectors. Yasin Varol and Hakan F. Oztop conducted a numerical analysis of natural convection heat transfer within an inclined solar collector. The focus of the study was to compare two different collector designs: a wavy collector and a flat plate collector. The findings of the research demonstrated that both the flow and thermal fields were significantly influenced by the shape of the enclosure (Varol & Oztop, Citation2008). Mansour conducted a thermal analysis using both experimental and numerical methods to investigate the performance enhancement achieved through the incorporation of a mini-channel array in a solar collector. The results revealed that the novel collector exhibited a 16.1% higher heat removal factor compared to the conventional collector (Mansour, Citation2013). Nan Wang et al. investigated to examine the impact of a corrugated upward surface and a flat downward surface, as well as the influence of tilt, in a solar collector. The findings revealed that, with a 30° tilt, an inlet water mass flow rate of 0.15 kg/s, and a 20 mm air gap distance, the collector exhibited the highest instantaneous efficiency (Wang et al., Citation2015). Abhishek et al. conducted theoretical studies to examine the impact of the amplitude and wavelength of the wavy fin on the thermal performance of a solar air heater. Their findings indicate that, across the entire range of mass flow rates and for a constant wavelength of 7 cm, the thermal efficiency increases as the amplitude of the wavy fin increases (Priyam & Chand, Citation2018).
Oussama Ibrahim et al. conducted a comprehensive study on an array of macro flat plate solar thermal collectors featuring rectangular channels. In their research, they proposed an optimization model to obtain an optimal design for the proposed collector. By solving this model, they successfully identified the most efficient configuration for the collector, resulting in significant advancements in its design and performance (Ibrahim et al., Citation2018).
2. Numerical studies on solar flat plate collectors
Numerical studies are widely conducted to analyse and evaluate the performance of mini solar flat plate collectors (Akbar et al., Citation2022). Mini solar flat plate collectors are designed to efficiently convert solar energy into thermal energy. This thermal energy can be utilized for various applications such as heating water or providing warmth to indoor spaces.
Numerical studies play a vital role in optimizing the design of mini solar flat plate collectors by accurately predicting and analysing their thermal performance under diverse operating conditions. These studies enable engineers and researchers to fine-tune the collector's design parameters to maximize its efficiency and effectiveness in harnessing solar energy. By simulating various scenarios and evaluating the thermal behaviour through numerical models, the optimal design configurations can be identified, leading to enhanced performance and improved utilization of solar energy. Previous numerical studies have extensively focused on utilizing data from simulations to investigate several crucial aspects related to mini solar flat plate collectors. These studies delve into areas such as analysing the flow pattern within the collector, studying heat transfer mechanisms, and assessing pressure drop characteristics. By employing advanced techniques, these studies can gain valuable insights into the complex fluid dynamics, heat transfer phenomena, and pressure distribution occurring within the collector. This comprehensive analysis supports the optimization of the design and performance of mini solar flat plate collectors, facilitating their efficient utilization in converting solar energy into thermal energy (Amara et al., Citation2023; Kansara et al., Citation2021; Mukanema & Simate, Citation2023; Unar et al., Citation2020). The flow pattern inside the collector can be analysed to identify any areas of stagnant flow or recirculation that may reduce its efficiency. Additionally, studying the heat transfer between the absorber plate and the fluid flowing through the collector can help optimize the design and maximize the heat transfer coefficient. Furthermore, numerical studies can help predict the pressure drop across the collector, which is an important parameter for ensuring the proper flow rate and avoiding excessive pumping power consumption. Moreover, these studies can investigate the effects of different materials and coatings on the collector's performance, as well as analyse the impact of environmental factors such as wind and solar irradiance.
Nanofluids were used as the fluid medium in a flat plate solar collector to examine the thermal performance of the collector (Said et al., Citation2022). The study was conducted under various nanofluid concentrations and flow rates. It has been found that a significant increase in the heat transfer coefficients is observed with the use of nanofluids in the flat plate collectors. Various studies have investigated the transient flow behaviour in a solar dryer using heat transfer models (Sileshi et al., Citation2022). The results of these studies have demonstrated that the drying process can be improved by incorporating vertical air distribution channels to enhance the homogeneity of the drying air distribution. Furthermore, combining numerical studies with experimental research offers the potential to enhance the performance of natural convection mixed type dryers. To enhance the efficiency of solar flat plate collectors, the utilization of phase change materials (PCMs) for storing latent thermal energy can be employed. A three-dimensional transient model was used to investigate a solar flat plate collector that integrates a PCM layer into its design (Badiei et al., Citation2020).
Each module employs a numerical solution to model heat transfer and fluid dynamics by solving the equations of energy and momentum. The results indicate that, despite providing hot water for a longer duration in the evening during discharge, the system equipped with phase change material (PCM) exhibits lower output temperatures in the morning. Additionally, the research findings reveal a notable enhancement in average collector efficiency during the summer months when utilizing phase change materials (PCMs) with lower minimum melting temperatures. Specifically, the average collector efficiency exhibits a substantial increase, rising from 33% to 46%. This observation contributes valuable insights to the field of heat transfer and fluid dynamics modelling, highlighting the potential benefits of employing PCMs in thermal energy systems. The recent study conducted by Nsofor EC et al. in 2021 presents insights related to the improvement of heat collectors in Phase Change Materials (PCMs) through the utilization of multiple PCMs in the passive thermal management of photovoltaic modules. The numerical simulations demonstrate that employing multiple PCMs based on the proposed arrangement can effectively increase the PCM melting time and extend the duration of photovoltaic (PV) thermal management by up to 18% and 33%, respectively. The extent of improvement depends on the PV inclination and the number of multiple PCMs in use (Nsofor et al., Citation2021).
Solar flat plate collectors are widely employed in low and medium-scale solar heating systems. These collectors play a crucial role in absorbing solar thermal energy, converting it into heat, and facilitating heat transfer to a fluid (typically water or air) that circulates through the collector. The effectiveness of a solar flat plate collector, as well as the fluid behaviour within a pipeline featuring three different cross sections, has been thoroughly investigated using CFD commercial code (Toapanta et al., Citation2020). The analysis of Computational Fluid Dynamics (CFD) results revealed that the solar flat plate collector featuring the Type I cross section achieved impressive temperatures of up to 330 K at the pipe outlet. This collector exhibited a remarkable efficiency of 68%, surpassing the efficiencies attained by the collectors with Type II and Type III cross sections, which achieved efficiencies of 51% and 60% respectively. The potential use of helical coiled inserts fixed inside a modified evacuated tube collector has been extensively studied by Singh and Vardhan (Citation2023) for air heating applications (Singh & Vardhan, Citation2023). The research involved experimental and Numerical investigations of the Evacuated Tube Solar Collector with Helical Coiled Inserts (ETSC-HI). A comparative analysis was conducted between the enhanced collector and an unmodified Evacuated Tube Collector (ETC) solar air heater, operating under similar conditions. The numerical investigations incorporated the fastening of the coiled wire to the vacuum pipe, enabling the determination of the optimal geometrical parameters that yield the highest heat transfer coefficient. These identified parameters have been carefully considered for future experimental studies, providing valuable insights for further research and design optimization. The comparative analysis revealed that the Evacuated Tube Solar Collector with Helical Coiled Inserts (ETSC-HI) outperformed the unaltered Evacuated Tube Collector (ETC) by a factor of 3.23, as indicated by the Nusselt number. Moreover, the maximum thermal efficiencies achieved were 70.99% for the ETSC-HI and 64.86% for the unmodified collector, highlighting a significant gain in efficiency with the implementation of the ETSC-HI design. Limited research has been conducted on the heat transmission characteristics of a solar water pump utilizing mono/hybrid nanofluids, specifically Cu/EO and Au – Cu/EO, in conjunction with a parabolic trough surface collector (PTSC) integrated into the solar water pump system (Ouni et al., Citation2022; Shahzad et al., Citation2022).
The review of the existing literature shows that numerical studies conducted on solar flat plate collectors can provide valuable insights into their thermal performance. These insights can be utilized to optimize their design and enhance their overall efficiency. Furthermore, ongoing research and development in this field are expected to drive further advancements in the design and performance of mini solar flat plate collectors. These developments will likely enhance their suitability for small-scale heating and hot water applications, making them an increasingly appealing choice. Therefore, the present study focuses on comprehensively investigating the flow behaviour and temperature distribution of air within a small-sized solar collector measuring 286 × 800 × 70 mm. Additionally, the design of the solar collector has been optimized to attain the highest possible outlet air temperature. Additionally, the solar collector's design has undergone meticulous optimization to achieve the utmost outlet air temperature. The comprehensive analysis involves the systematic variation and analysis of various parameters, including the diameter of wavy structure holes, ambient conditions, and solar intensities. This approach enables a thorough understanding of flow behaviour and temperature distributions within the system.
3. Numerical methodology
The numerical studies are conducted using a commercial Computational Fluid Dynamics (CFD) solver. To ensure the accuracy and reliability of the simulations, established practices from the literature are adhered to for mesh generation and refinement. These practices are implemented to create an appropriate mesh and subsequently refine it. The numerical models and physical models employed in this study are carefully selected from the existing literature to effectively address and minimize numerical errors. By incorporating well-established models, the study aims to enhance the accuracy and reliability of the numerical simulations, thereby ensuring the robustness of the findings and minimizing potential uncertainties (Jha et al., Citation2022; Kabir, Citation2022; Vandrangi, Citation2022; Velidi, Citation2022). The basic methodology employed for the CFD simulation in this study is illustrated in Figure , outlining the systematic approach adopted to ensure accurate and reliable results.
3.1. Model description
The model of a wavy structure solar flat plate collector is created using Computer-Aided Design (CAD) tools, taking into account a thorough understanding of the product's intended purpose and the desired design objectives. These objectives may encompass various aspects such as specific functionality, aesthetic considerations, desired size, and other relevant criteria. The CAD tools enable the precise development and visualization of the collector design, ensuring that the final product meets the specified requirements. A two-dimensional model of the wavy structure is designed using CAD drawing tools. This approach facilitates the visualization of the design and allows for refinement before proceeding to create a three-dimensional (3D) model. By utilizing CAD drawing tools, the intricacies of the wavy structure can be accurately represented, enabling a comprehensive assessment and optimization of the design. The two-dimensional model serves as an essential step in the design process, ensuring that the final 3D model is refined and meets the desired specifications. To generate a 3D representation of the wavy structure solar flat plate collector, a series of steps are followed. Firstly, a flat plate collector surface is created as the base. Then, the wavy structure is added to the surface, accurately capturing its intricate design details. To ensure accuracy and precision, constraints are implemented to align and constrain the components of the model. This meticulous process results in a comprehensive and realistic 3D representation of the wavy structure solar flat plate collector, facilitating further analysis, optimization, and visualization of the design. To create a complete and realistic model, additional features are incorporated, including inlet and outlet pipes, connections, and other essential design elements. These additions ensure that the model accurately represents the actual structure and functionality of the wavy structure solar flat plate collector. Furthermore, materials and textures are applied to the model to enhance its visual appearance and realism. This step involves assigning appropriate properties to different components of the model, such as the collector surface, pipes, and connectors. By applying materials and textures, the model gains a lifelike representation, allowing for a more immersive and realistic visualization of the wavy structure solar flat plate collector. Designing a wavy structure solar flat plate collector involves adding a wavy design to the surface of a flat plate collector, which creates turbulence in the fluid flow and enhances the heat transfer coefficient. The wavy design can be created using various methods, including parametric modelling, spline modelling, or using pre-made geometry. Once the wavy structure is created, it can be integrated into the flat plate collector surface to create the final model. Figure shows the CAD model developed for this study and Figure (a–e) are the 2D sketches for a better understanding of the flow pattern of the CAD model.
3.2. Definition of schematic configuration
The study focuses on investigating the flow and temperature distributions by optimizing the design in three distinct variations, as illustrated in Table and Figure .
Table 1. Design variants for solar flat plate collector.
3.3. Mesh generation and refinement
The utilization of precise and efficient discrete local approximations (mesh) of the solar flat plate collector CAD model enables the capture of flow and temperature distributions accurately. Meshing is a critical step in the design process of a solar flat plate collector investigations, as it determines the accuracy of the results obtained through simulations. The geometry, size, and material properties of the collector are into consideration to create a mesh that accurately represents the physics of heat transfer and fluid flow within the collector. The meticulous approach to meshing ensures that simulations provide reliable and accurate results, allowing to make informed decisions about the design and performance of their solar flat plate collector. Figure shows the mesh generated for the CAD model. Coarse mesh considered over the wavy structure surface, to avoid creation of high cell count and to reduce the run-time.
Figure 5. CAD Model Mesh, (a) Inlet-side (Maximum Aspect Ratio = 35.82, Skewness: 0.9); (b) outlet-side (maximum aspect ratio = 35.82, Skewness: 0.9).

Figure shows the grid dependence study performed with various mesh sizes. A grid dependence study is a critical analysis conducted to assess the influence of computational grid resolution on the accuracy and reliability of numerical simulations, particularly in computational fluid dynamics (CFD) or other numerical modelling techniques. The computational domain is divided into a grid or mesh, consisting of discrete cells or elements. The grid can be structured (regular) or unstructured (irregular), depending on the nature of the problem and the numerical method employed. Grid dependence studies help in understanding the sensitivity of the numerical model to grid resolution in choosing an appropriate grid for the simulations. With various grid sizes, the simulations are performed till convergence criteria is reached and found that the accuracy and consistency of the simulation has been achieved with 888234 grid elements.
3.4. Boundary conditions
To enhance computational efficiency, the solution adopted the application of uniform mass flow conditions at the inlet and a uniform heat transfer coefficient on the absorber plate's wall. The analysis assumed smooth geometry surfaces, allowing for smooth airflow over them. The analysis assumes smooth surfaces in the geometry, allowing for frictionless airflow over them. Additionally, the study utilizes the standard k-epsilon turbulence model for the computational fluid dynamics (CFD) simulation. The simulation is conducted assuming 3D steady-state conditions, where the fluid is considered to be air under ideal conditions. However, the analysis does not incorporate the radiation model, transmitting coefficient, and emissivity coefficient. Furthermore, it only considers the surface area of the pebble block and does not include its solid domain. Additionally, the analysis does not account for the thickness of the glass. Moreover, the effect of pressure inside the solar flat plate collector is not taken into consideration. The boundary conditions are determined based on the available data obtained from the literature (Ingle et al., Citation2013).
The main operating conditions commonly employed in CFD simulations of solar flat plate collectors encompass the inlet and outlet velocities, as well as the temperature and heat flux boundary conditions. These parameters are fundamental for accurately modelling and analysing the performance of the collectors. Operating conditions play a crucial role in ensuring the accuracy and reliability of CFD simulations for solar flat plate collectors. To accurately model the intricate fluid flow and heat transfer phenomena within the collector, it is imperative to define appropriate operating conditions for each component of the model. The inlet velocity boundary condition is utilized to simulate the incoming airflow into the collector, while the outlet velocity operating condition represents the airflow exiting the collector.
The temperature and heat flux operating conditions are crucial in accurately simulating the heat transfer process within the collector. These conditions are used to define the temperature and heat flux at the surfaces of the collector, ensuring accurate modelling of the heat transfer phenomena. It is important to note that the selection of appropriate operating conditions for a particular simulation will depend on the specific geometry, materials, and operating conditions of the collector being analysed. Therefore, careful consideration must be given to selecting the appropriate operating conditions for each simulation. This is essential to obtain accurate results that can inform the design and optimization of solar flat plate collectors. Table details the material properties considered for different materials in this study. A total of twenty-seven (27) cases has been simulated to understand the flow and temperature distributions. As detailed earlier in this manuscript, three geometric designs are considered (G1, G2 and G3). Each geometric design is investigated with a constant mass flow rate and with varied solar intensity and ambient temperatures. Table details the operating conditions considered for geometric designs G, G2 and G3.
Table 2. Material properties.
Table 3. Operating conditions for G1, G2 and G3.
3.5. Parametric study and governing equations
Numerical studies were conducted to examine the influence of the wavy structure, ambient conditions, and solar intensities on the flow and temperature distributions within a mini solar flat plate collector. The solver conditions are defined by the numerical methods, algorithms, and parameters used to solve the governing equations that describe the fluid flow and heat transfer phenomena within the collector. There are several factors to consider when selecting the appropriate solver conditions for CFD simulations of solar flat plate collectors includes the discretization schemes used for space and time, the turbulence models used to simulate the turbulent flow, and the convergence criteria used to terminate the simulations. In addition, the choice of solver conditions may be influenced by the specific geometry, materials, and operating conditions of the collector being simulated. The procedure adopted to simulate the solar air collector is as follows The 3D model has been modelled by using ANSYS WORKBENCH software After creation of 3D model, the unstructured grid has been created by using ANSYS Meshing software The unstructured grid created consist around one million mesh elements, The unstructured grid created, imported in ANSYS FLUENT The model was defined by using 3D segregated solver with steady condition, energy equation, and K-epsilon of viscous model. Air at ideal condition is used as working fluid.
CFD aids in understanding patterns of flow that are otherwise impossible, expensive, or difficult to investigate via the use of experimental methods (Anderson & Wendt, Citation1995). In process industries, the CFD concept applies to different disciplines and its growing use has reduced the demand for physical experiments. In laminar flow regimes, differential equations for mass, momentum, and energy govern the heat transfer and fluid flow processes. The governing equations are as follows:
Continuity equation:
(1)
(1) Momentum equation:
(2)
(2)
Energy equation:
(3)
(3) Notably, the differential equations concerning the parameters of mass, momentum, and energy are applicable to laminar flows. Based on the target flow regime, these differential equations can be modified. In heat exchangers, crude oil fouling occurs under turbulent conditions. This turbulent nature is experienced because the heat exchangers have complex geometries and also experience higher velocities, examples being shell and tube heat exchangers. Also, almost all the studies focusing on crude oil fouling involve multi-phases such as fluids and solids. The presence of particles and the influencing forces will affect the turbulence in the bulk fluid. A high turbulence is said to be generated at the interfaces between the phases (Knotek et al., Citation2017). Therefore, a detailed understanding of the fluid flow modelling involving turbulence, multiphase flow and heat transfer modelling is necessary to predict the crude oil fluid flow behaviour in the heat exchangers. The differential equation of energy is responsible for the heat transfer modelling of the fluid flow. The turbulence and multiphase modelling of the fluid flow are described below.
3.6. Turbulence modelling for the fluid flow
Intensive research has been extended to turbulent flows, especially due to their modelling and application in various contexts of fluid dynamics. The turbulent flow is simulated through CFD with one-equation, two-equation, and seven-equation models. In one-equation turbulence model such as Spalart-Allmaras, the eddy viscosities are dependent on the turbulent kinetic energies. However, the modelling of turbulent length scales and capturing the turbulence with flow fluctuations and associated eddies remained as a limitation for the one-equation turbulence models (Celik, Citation1999). In order to model the turbulent length scales and to capture the eddies in the fluid flow, two-equation modes such as k-ε and k-ω turbulence models were developed. In situations, where the k-ε turbulence model is employed, the turbulent dissipation (ε) is used to compute the turbulent kinetic energy’s rate of dissipation while the turbulent kinetic energy (k) is used to compute the energy in the turbulence. In crude oil fouling, the turbulence model that has been employed in most cases involves the k-ε model (Emani et al., Citation2016; Fontoura et al., Citation2013; Fouzia & Park, Citation2017; Li et al., Citation2015; Nazar et al., Citation2016; Souza et al., Citation2006). Indeed, the assumption of this model is that the simulation demands less computational time because the turbulence is isotropic.
Turbulent kinetic energy (k) is computed by:
(4)
(4) On the other hand, ε (epsilon), which represents the rate of dissipation, is established as:
(5)
(5)
Given a specific dissipation rate (ω) for turbulence kinetic energy, the turbulence is predicted through the use of the k-ω turbulence model. Indeed, the latter model poses merits in such a way that it is capable of simulating flows exhibiting adverse pressure gradients. Also, this model is advantageous whereby it has been found to exhibit numerical stability, besides providing precise simulation outcomes (when compared to situations where k–ε turbulence models are employed) (Haghshenasfard & Hooman, Citation2015; Wilcox, Citation1998) and it is noticed that few studies focusing on crude oil fouling have employed the k–ε turbulence model (Bayat et al., Citation2012; Haghshenasfard & Hooman, Citation2015; Seyyedbagheri & Mirzayi, Citation2017). In the k–ε turbulence model, the determination of the specific dissipation rate, ω (omega) is achieved by:
(6)
(6) A general limitation for the two-equation turbulence models is that they are developed to describe only isotropic turbulence. Seven-equation turbulence model such as Reynolds Stress Model (RSM) is known to solve the anisotropic turbulence in the flow field. In RSM, Reynolds transport equations are employed to compute the net transfer rate of momentum, with particular focus on the fluid produced by the turbulence. However, it is notable that the RSM differential transport equations are complex and reduce numerical robustness. This reduction accounts for their decreasing use in complex flows, especially because they also demand an increase in the computational effort.
To establish a Reynolds transport equation, the computation is presented as:
(7)
(7) Indeed, a turbulent flow regime is a fluid motion state that is not only random but also chaotic. This regime experiences laminar flow fluctuations, especially in terms of fluid streamlines. As such, there is a need to model the fluid flow’s fluctuating parameters within the turbulent flow conditions, besides adjusting the differential equations for energy, momentum, and mass. For velocity and other flow parameters, decomposition yields a fluctuating component (
), as well as a steady mean value (
). Relative to the differential equations for energy, momentum, and mass, the fluid velocity becomes:
(8)
(8) For the fluctuating parameters linked to the differential equations for mass, momentum, and energy to be applied in the new equation sets, the Reynolds Averaged Navier Stokes (RANS) equations are employed. Particularly, the Reynolds Averaged Navier Stokes (RANS) equations represent time-averaging techniques and the Reynolds stress turbulence models, two-equation, and one-equation models belong to the RANS models. In CFD, Direct Numerical Simulation (DNS) and Large Eddy Simulations (LES) are also employed to model the turbulent flow. Imperative to indicate is that when LES is used in CFD simulation, the smallest length scales are ignored. This decision is informed by the affirmation that resolving these smallest length scales is expensive. Additionally, the use of LES in CFD simulation does not demand a turbulence model to unearth the impact of turbulence. As such, LES captures only larger length scales. In situations, where the DNS model is employed, turbulence flows are simulated without using a turbulence model (Orszag, Citation1970). Imperative to highlight is that DNS demands a lot of memory and computational time. Given the limitations of LES and DNS, basic conservation equations concerning the parameters of mass, momentum, and energy fail to resolve turbulent flow regimes’ fluid motions (Sun, Citation2007). Hence, most of the cases where turbulent flows are present have witnessed increasing application of RANS equations, which aid in approximating the flow domain’s turbulent fluctuation impact (Kohnke, Citation2001).
4. Results and discussions
The simulation results of a solar flat plate collector using CFD provided valuable information about the thermal and flow characteristics of the collector. One of the key outputs of a CFD simulation is the temperature distribution on the surface of the collector. This information can help in assessing the effectiveness of the collector in capturing and transferring heat from the sun to the fluid passing through it. The temperature distribution can also provide insights into the design of the collector, such as the location of the inlet and outlet ports, and the placement and orientation of the absorber plate. Another important output of a CFD simulation is the velocity and pressure distribution of the fluid inside the collector. This information can help in understanding the flow behaviour of the fluid, and in optimizing the flow path to minimize pressure drop and maximize heat transfer.
The simulation results can also be used to calculate the total heat useful for the collector. Total heat useful value is an important parameter that represents the rate of heat transfer from the absorber plate to the fluid. A higher total heat useful value indicates a more efficient collector and can help in optimizing the design of the collector to achieve higher thermal efficiency. The simulation results can be compared with experimental data to validate the accuracy of the simulation model. Any discrepancies between the simulation and experimental data can help in identifying areas for improvement in the simulation model, and in refining the design of the collector. The flow behaviour and temperature distribution contour plots from the CFD simulations are provided in the Appendix (Figures A1–A12). Tables detail the outlet temperatures, total heat, and efficiencies for geometric designs G1, G2 and G3, respectively. Also, change in inlet design achieved uniform flow distribution from inlet to outlet, compared to research carried in ‘CFD Analysis of Solar Flat Plate Collector (Ingle et al., Citation2013)’. In comparison with the CFD simulation investigations of solar flat plate collector provided in (Ingle et al., Citation2013), the efficiencies and maximum outlet temperatures are higher in the present design of mini solar flat plate collector, might be due to more heat absorption in mini solar flat plate collector. The equations required for total heat useful, total heat, efficiency and outlet temperature are provided below,
(9)
(9)
(10)
(10)
(11)
(11)
(12)
(12)
Table 4. Temperature distribution for geometric design 1 (G1).
Table 5. Temperature distribution for geometric design 2 (G2).
Table 6. Temperature distribution for geometric design 3 (G3).
5. Conclusions
The study focuses on examining the effect of hole diameter in the wavy structure on the overall performance of the solar flat plate collector. Through numerical simulations, it is observed that even a slight variation in the hole diameter of the wavy structure results in a significant impact on the maximum outlet temperature of the mini solar flat plate collector. This finding highlights the importance of optimizing the hole diameter for achieving the highest possible outlet temperature and enhancing the performance of the solar flat plate collector.
The study reveals that there is no reduction in the outlet temperatures as the number of outlets for the collector increases. Furthermore, a consistent average temperature is observed across all the outlets. However, it is worth noting that with higher ambient temperatures and solar intensities, a significant increase in the outlet temperature is observed. Particularly, in the case of G1, the highest efficiency is achieved, reaching a remarkable value of 78.50%. This observation highlights the strong influence of ambient temperature and solar intensity on the performance of the system. Specifically, the outlet temperatures reach their maximum values under higher solar intensities. These findings indicate the influence of ambient temperature and solar intensity on the outlet temperature, underscoring the importance of considering these factors when assessing the performance of the collector.
A reduction in collector efficiency is evident as solar intensities increase. This can be attributed to the higher total heat loss occurring within the collector. It is possible that additional heat is required to enhance the absorptivity of the material utilized in the simulation, resulting in decreased efficiency.
The modified inlet design successfully achieved a uniform flow distribution from the inlet to the outlet, surpassing the flow distribution observed in existing literature. This improvement may be attributed to enhanced heat absorption capabilities in the mini solar flat plate collector.
To further enhance the study, additional simulations can be performed to integrate a radiation model and accurately model the pebble block in the mini solar flat plate collector. Furthermore, investigating the pressure drop in the collector can provide valuable insights. An alternative sub-domain model can be developed to explore the perforation effects, replacing the existing wavy structure. These advancements will contribute to a more comprehensive understanding of the system's performance.
Disclosure statement
No potential conflict of interest was reported by the author(s).
References
- Akbar, M., Manzoor, T., & Awais, H. (2022). CFD-thermal analysis of flat plate solar collector for different temperature variations. International Journal of Energy and Water Resources, 6(3), 315–321. https://doi.org/10.1007/s42108-021-00177-7
- Amara, W. B., Aryanfar, Y., Koten, H., Bouabidi, A., Chrigui, M., & Alcaraz, J. L. G. (2023). CFD analysis of the effect of internal peak angle and mass flow rates on the thermal performance of solar air heater with triangle cross-section. Thermal Science, 46–46. https://doi.org/10.2298/TSCI220918046A
- Anderson, J. D., & Wendt, J. (1995). Computational fluid dynamics (Vol. 206). Springer.
- Badiei, Z., Eslami, M., & Jafarpur, K. (2020). Performance improvements in solar flat plate collectors by integrating with phase change materials and fins: A CFD modeling. Energy, 192, 116719. https://doi.org/10.1016/j.energy.2019.116719
- Bayat, M., Aminian, J., Bazmi, M., Shahhosseini, S., & Sharifi, K. (2012). CFD modeling of fouling in crude oil pre-heaters. Energy Conversion and Management, 64, 344–350. https://doi.org/10.1016/j.enconman.2012.05.003
- Celik, I. B. (1999). Introductory turbulence modeling. Western Virginia University.
- Emani, S., Ramasamy, M., & Shaari, K. Z. B. K. (2016). Effect of shear stress on crude Oil fouling in a heat exchanger tube through CFD simulations. Procedia Engineering, 148, 1058–1065. https://doi.org/10.1016/j.proeng.2016.06.592
- Fontoura, D., Matos, E., & Nunhez, J. (2013). A three-dimensional two-phase flow model with phase change inside a tube of petrochemical pre-heaters. Fuel, 110, 196–203. https://doi.org/10.1016/j.fuel.2012.09.065
- Fouzia, D., & Park, T. S. (2017). Effect of inlet velocity on the crude Oil coking and Gas phase formation in a straight pipe. Journal of Applied Mathematics and Physics, 05(01), 17. https://doi.org/10.4236/jamp.2017.51003
- Haghshenasfard, M., & Hooman, K. (2015). CFD modeling of asphaltene deposition rate from crude oil. Journal of Petroleum Science and Engineering, 128, 24–32. https://doi.org/10.1016/j.petrol.2015.01.037
- Ibrahim, O., Younes, R., & Ibrahim, M. (2018). Macro flat-plate solar thermal collector with rectangular channels. Journal of Solar Energy Engineering, 140(6). http://doi.org/10.1115/1.4040535
- Ingle, P., Pawar, A., Deshmukh, B., & Bhosale, K. (2013). CFD analysis of solar flat plate collector. International Journal of Emerging Technology and Advanced Engineering, 3(4), 337–342.
- Jha, V., Velidi, G., & Emani, S. (2022). Optimization of flame stabilization methods in the premixed microcombustion of hydrogen–air mixture. Heat Transfer, 51(6), 5896–5918. https://doi.org/10.1002/htj.22574
- Kabir, M. A. (2022). Design and steady state thermal analysis of aircraft fuselage. Journal of Airline Operations and Aviation Management, 1(1), 71–79. https://doi.org/10.56801/jaoam.v1i1.9
- Kansara, R., Pathak, M., & Patel, V. K. (2021). Performance assessment of flat-plate solar collector with internal fins and porous media through an integrated approach of CFD and experimentation. International Journal of Thermal Sciences, 165, 106932. https://doi.org/10.1016/j.ijthermalsci.2021.106932
- Karki, S., Haapala, K. R., & Fronk, B. M. (2019). Technical and economic feasibility of solar flat-plate collector thermal energy systems for small and medium manufacturers. Applied Energy, 254, 113649. https://doi.org/10.1016/j.apenergy.2019.113649
- Knotek, S., Fiebach, A., Schmelter, S., & Schmeyer, E. (2017). Multiphase flow metrology in oil and gas production. EURAMET Project ENG58. Final publishable JPR report.
- Kohnke, P. (2001). ANSYS theory manual. ANSYS Inc, 40.
- Li, X.-G., Zhang, L.-H., Zhang, R.-Y., Sun, Y.-L., Jiang, B., Luo, M.-F., & Li, X.-G. (2015). CFD modeling of phase change and coke formation in petroleum refining heaters. Fuel Processing Technology, 134, 18–25. https://doi.org/10.1016/j.fuproc.2015.03.005
- Lizama-Tzec, F. I., Herrera-Zamora, D. M., Arés-Muzio, O., Gómez-Espinoza, V. H., Santos-González, I., Cetina-Dorantes, M., Vega-Poot, A. G., García-Valladares, O., & Oskam, G. (2019). Electrodeposition of selective coatings based on black nickel for flat-plate solar water heaters. Solar Energy, 194, 302–310. https://doi.org/10.1016/j.solener.2019.10.066
- Mahdi, J. M., Mohammed, H. I., & Talebizadehsardari, P. (2021). A new approach for employing multiple PCMs in the passive thermal management of photovoltaic modules. Solar Energy, 222, 160–174. http://doi.org/10.1016/j.solener.2021.04.044
- Mansour, M. K. (2013). Thermal analysis of novel minichannel-based solar flat-plate collector. Energy, 60, 333–343. http://doi.org/10.1016/j.energy.2013.08.013
- Mukanema, M., & Simate, I. N. (2023). CFD simulation of temperature and Air flow in a natural convection solar tunnel dryer with a bare flat-plate chimney. Energy and Environment Research, 13(1), 1. https://doi.org/10.5539/eer.v13n1p1
- Nazar, S., Ali, R., Banisharifdehkordi, F., & Ahmadzadeh, S. (2016). Mathematical modeling of coke formation and deposition due to thermal cracking of petroleum fluids. Chemical Engineering & Technology, 39(2), 311–321. https://doi.org/10.1002/ceat.201400528
- Orszag, S. A. (1970). Analytical theories of turbulence. Journal of Fluid Mechanics, 41(2), 363–386. https://doi.org/10.1017/S0022112070000642
- Ouni, M., Ladhar, L. M., Omri, M., Jamshed, W., & Eid, M. R. (2022). Solar water-pump thermal analysis utilizing copper–gold/engine oil hybrid nanofluid flowing in parabolic trough solar collector: Thermal case study. Case Studies in Thermal Engineering, 30, 101756. https://doi.org/10.1016/j.csite.2022.101756
- Priyam, A., & Chand, P. (2018). Effect of wavelength and amplitude on the performance of wavy finned absorber solar air heater. Renewable Energy, 119, 690–702. http://doi.org/10.1016/j.renene.2017.12.010
- Said, Z., Sharma, P., Sundar, L. S., Li, C., Tran, D. C., Pham, N. D. K., & Nguyen, X. P. (2022). Improving the thermal efficiency of a solar flat plate collector using MWCNT-Fe3O4/water hybrid nanofluids and ensemble machine learning. Case Studies in Thermal Engineering, 40, 102448. https://doi.org/10.1016/j.csite.2022.102448
- Seyyedbagheri, H., & Mirzayi, B. (2017). CFD modeling of high inertia asphaltene aggregates deposition in 3D turbulent oil production wells. Journal of Petroleum Science and Engineering, 150, 257–264. https://doi.org/10.1016/j.petrol.2016.12.017
- Shahzad, F., Jamshed, W., Safdar, R., Hussain, S. M., Nasir, N. A. A. M., Dhange, M., Nisar, K. S., Eid, M. R., Sohail, M., Alsehli, M., & Elfasakhany, A. (2022). Thermal analysis characterisation of solar-powered ship using oldroyd hybrid nanofluids in parabolic trough solar collector: An optimal thermal application. Nanotechnology Reviews, 11(1), 2015–2037. https://doi.org/10.1515/ntrev-2022-0108
- Sileshi, S. T., Hassen, A. A., & Adem, K. D. (2022). Simulation of mixed-mode solar dryer with vertical air distribution channel. Heliyon, 8(11), e11898. https://doi.org/10.1016/j.heliyon.2022.e11898
- Singh, I., & Vardhan, S. (2023). Experimental and CFD investigation on heat transfer enhancement in evacuated tube solar collector with coiled wire inserts. Energy Sources, Part A: Recovery, Utilization, and Environmental Effects, 45(2), 3742–3759. https://doi.org/10.1080/15567036.2023.2201187
- Souza, B., Matos, E., Guirardello, R., & Nunhez, J. (2006). Predicting coke formation due to thermal cracking inside tubes of petrochemical fired heaters using a fast CFD formulation. Journal of Petroleum Science and Engineering, 51(1), 138–148. https://doi.org/10.1016/j.petrol.2005.11.013
- Sun, D.-W. (2007). Computational fluid dynamics in food processing. CRC Press.
- Toapanta, L. F., Anthony Xavier, A., & Quitiaquez Sarzosa, W. (2020). CFD analysis of a solar flat plate collector with different cross sections. Enfoque Ute, 11(2), 95–108. https://doi.org/10.29019/enfoque.v11n2.601
- Unar, I. N., Maitlo, G., Ahmed, S., Ali, S. S., Memon, A. Q., Kandhro, G. A., & Jatoi, A. S. (2020). Performance evaluation of solar flat plate collector using different working fluids through computational fluid dynamics. SN Applied Sciences, 2(1), 1–10. https://doi.org/10.1007/s42452-019-1685-8
- Vandrangi, S. K. (2022). Aerodynamic characteristics of Naca 0012 airfoil by Cfd analysis. Journal of Airline Operations and Aviation Management, 1(1), 1–8. https://doi.org/10.56801/jaoam.v1i1.1
- Varol, Y., & Oztop, H. F. (2008). A comparative numerical study on natural convection in inclined wavy and flat-plate solar collectors. Building and Environment, 43(9), 1535–1544. http://doi.org/10.1016/j.buildenv.2007.09.002
- Velidi, G. (2022). Pressure and velocity variation In remote-controlled plane using Cfd analysis. Journal of Airline Operations and Aviation Management, 1(1), 9–18. https://doi.org/10.56801/jaoam.v1i1.2
- Wang, N., Zeng, S., Zhou, M., & Wang, S. (2015). Numerical study of flat plate solar collector with novel heat collecting components. International Communications in Heat and Mass Transfer, 69, 18–22. http://doi.org/10.1016/j.icheatmasstransfer.2015.10.012
- Wilcox, D. C. (1998). Turbulence modeling for CFD (Vol. 2). DCW Industries.