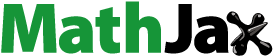
Abstract
A pump−pipeline experiment platform is designed and built for exploring the dynamic flows in a pipe-conveying fluid with entrapped air, and a corresponding CFD simulation is executed based on the volume-of-fluid (VOF) model. The transient flow characteristics of the entrapped air in pipelines under different pump and valve working conditions are studied by experimental and numerical approaches. The experimental data and simulation results are in good agreement. Both of them show that the pressure impact can be mitigated by increasing the air volume ratio (air volume/pipe volume) or the valve opening time. Two types of the air–water interfaces, a clear interface and a broken interface, appear in the process of air pressurization to the peak pressure as the flow rate rises and the valve opening time decreases. The influence of the flow rate, air volume and valve opening time on the peak pressure and arrival time are investigated, and a corresponding equation with respect to the dimensionless parameters is proposed for the pressure prediction of a system with entrapped air during start-up.
1. Introduction
The pipeline system is an important part of fluid transportation and energy transmission in the energy, petrochemical and other industries. A common pipeline system composed of pipes, flow control valves and pumps for conveying fluids can be specified as a pump−pipeline system. When entrapped air exists in a pump−pipeline system, the entrapped air could be impacted when the pump and valve are started, resulting in transient pressure impact and pressure fluctuation. The flow characteristics caused by entrapped air in pipes may pose a threat to the normal operation and safety of a pipeline system. Accurately predicting the dynamic flow characteristics of pump−pipeline systems with entrapped air in the start-up condition can ensure its operation stability and safety.
Pump−pipeline systems are widely employed in engineering applications. Since the fluid flow is directly related to operational performance and system safety, many scholars have paid attention to research on the fluid flow characteristics, especially the flow interaction effects between pumps, valves, pipes, etc. Nikpour et al. (Citation2014) conducted a two-dimensional (2D) computational fluid dynamics (CFD) simulation of the water hammer in a pump−pipeline system, and compared different turbulence models according to the experiment data. Gu et al. (Citation2021, Citation2022) analysed and discussed the influence of the interaction between pump and pipeline on the transient flows by experimental, theoretical and numerical approaches. Wan et al. (Citation2018) established a numerical model based on the method of characteristics (MOC) and found that increasing the pump’s moment of inertia had positive effects on water hammer control. The above studies focus on single-phase flow, while two-phase flow is not considered.
In the pump−pipeline system, air may be trapped as air pockets in the pipe owing to the release of bubbles dissolved in the liquid, rapid injection after interruption, and other reasons. A significant pressure impact of entrapped air caused by changes of operating conditions has been found in practical pump−pipeline systems. The corresponding problem has attracted the attention of some scholars. Izquierdo et al. (Citation1999) used a one-dimensional (1D) model to study rapid pressurization in a pump−pipeline containing multiple entrapped air pockets, and concluded that smaller air pockets would induce higher peak pressure. Fuertes et al. (Citation1999) extended the work of Izquierdo et al. (Citation1999) and investigated the effects of several relevant parameters on air peak pressure, including the characteristic curve shape of the pump, the slope of the pipe and friction. However, these studies only carried out numerical analysis, and research on the effects of some of the key factors, such as valve opening time, flow rate, etc., on pressure impact was relatively lacking. The existing research results are insufficient to solve the pressure impact problem caused by entrapped air in pump−pipeline systems. It is necessary to conduct systematic and in-depth research on the dynamic flow characteristics of pump−pipeline systems with entrapped air, especially during start-up.
Conversely, research on simple pipeline with entrapped air, especially reservoir pipelines, has been carried out earlier. Sudden compression of the entrapped air in reservoir pipelines has been identified as the reason for transient pressure impact (Martin, Citation1976; Wylie et al., Citation1993). It has also been confirmed by many scholars through experiments and engineering cases (Pozos-Estrada et al., Citation2012; Wright et al., Citation2012; Zhou, Liu, Karney, et al., Citation2011). Early numerical models for transient flows with entrapped air were usually 1D and could be divided into three categories: the rigid water column model (RWCM) (Izquierdo et al., Citation1999; Martin, Citation1976; Zhou et al., Citation2002), the elastic water model (EWM) (Chaudhry, Citation1979; Wylie et al., Citation1993) and the Preissmann slot method (PSM). RWCM neglects the compressibility of the fluid and the elasticity of the pipe walls, while EWM considers both. RWCM and EWM assume that the air–water interface is perpendicular to the pipeline. However, Zhang et al. (Citation2020) provided a way to avoid this assumption by using MOC to solve the pressure flow and free surface flow. Based on PSM, Vasconcelos and Wright (Citation2004) incorporated air phase pressure into the momentum equation to solve the pressurized flow under limited ventilation. One-dimensional numerical models are idealized and simplified, and provide limited information. Many studies have reported that 1D models may overestimate the peak pressure and misjudge the variation rule of peak pressure (Huang & Zhu, Citation2021; Zhou, Liu, Karney, et al., Citation2011). Therefore, 1D numerical models are limited as regards studying transient flows with entrapped air. The development of CFD and the volume-of-fluid (VOF) model has provided an attractive approach for further understanding the dynamic behaviour of two or more fluids. He et al. (Citation2022) described the dynamic behaviour of an isolated slug driven by pressurized air in a voided line with an end orifice by a three-dimensional (3D) CFD model. Zhou, Liu, and Ou (Citation2011) were the first to apply the VOF model to the simulation of transient flow in a water filled pipe containing entrapped air. Many studies have confirmed that CFD and the VOF model are more effective than 1D models in flow simulation with entrapped air in terms of physical correlation and numerical accuracy (Hargreaves et al., Citation2007; Martins, Citation2012).
The transient flows are different in reservoir−pipeline and pump−pipeline system (Malekpour, Citation2014). Advanced methods and rich contents are emerging in the study of reservoir−pipeline systems with constant pressure upstream, while the research about pump−pipeline systems are still relatively simple. Vasconcelos and Wright (Citation2009) believe that the use of multi-dimensional models to simulate the formation and movement of air pockets is an important improvement for dynamic flows in pump−pipelines with air. Pozos-Estrada et al. (Citation2016) proved that it is desirable to analyse the potential destructive effects of air pockets on hydraulic transients for various conditions of pump operation. Therefore, it is necessary to study the dynamic flows of pump−pipeline systems by CFD simulation. Unlike a constant pressure in the reservoir, the output pressure of the pump is difficult to control accurately in an experiment, and the function of the pump cannot be expressed easily in CFD. This is a major obstacle to the study of transient flows in pump−pipeline systems with entrapped air.
In this article, experiments and CFD numerical simulations are carried out to study systematically the dynamic flow of pump−pipeline systems with entrapped air during start-up. The output pressure of the pump is controlled in the experiments and CFD by using the characteristic curve of the pump. The influence is analysed of three important parameters, namely the flow rate, the entrapped air volume and the valve opening time, on the peak air pressure and the arrival time of peak pressure. A calculation equation for peak air pressure considering the above three parameters is proposed with respect to the dimensionless parameters. The movement of entrapped air is preliminarily understood through experiments and CFD, and the flow information of CFD provides a basis for further research and interpretation of air dynamics.
2. Experimentation and CFD simulation of pump−pipelines with entrapped air
An experimental system of dynamic flow with entrapped air is designed and built in order to study the transient pressure impact on the pump−pipeline system with entrapped air during start-up. Based on the experimental system and working conditions, a 2D-VOF CFD model of a pump−pipeline system with entrapped air is established so as to conduct numerical simulation analyses and combine with the experimental results to explore the action mechanism and influence of flow rate, air volume and valve opening time on the dynamic flow characteristics.
2.1. Experimental system
As shown in Figure , an experimental system consisting of a pump−pipeline with entrapped air is designed and built to study the effects of flow rate, air volume and valve opening time on the dynamic flow characteristics, and relevant experimental tests and data analyses are carried out.
Figure 1. Experimental system for the dynamic flow characteristics of a pump−pipeline system with entrapped air: (a) general view of the experimental system (1 − horizontal pipe with entrapped air, 2 − dead-end valves, 3 − pump and control valve, 4 − water tank, 5 − air injection system); (b) pressure sensor and dead-end valve; (c) air injection system; (d) pump and control valve; (e) control and power system.

The system is composed of a polymethyl methacrylate (PMMA) pipe with entrapped air, a dead-end valve, a pump and control valve, a water tank, an air injection system, a control and power system, a main pipe, a return pipe, and elbows, tees, etc. The main pipe, the size of which is DN100, is made of stainless steel. At the back of the pipeline, a horizontal pipe of length 2 m and a vertical pipe of length 0.4 m are the PMMA pipes with entrapped air, which can facilitate the observation of liquid level height and fluctuation behaviour. The end of the pipeline forms a dead end by closing the valve, as shown in Figures (a) and (b). The entrapped air is formed and controlled by an air compressor, an air transmission pipe, a control valve, etc. (Figures (a) and (c)). The water comes from the water tank, the maximum volume of which is 8 m3. As shown in Figures (a) and (d), the pressure source is a horizontal centrifugal pump with a frequency conversion function, which could be used to achieve different flow rates. The control valve, located behind the centrifugal pump, is a quarter-ring butterfly valve that opens at a constant speed, and the opening time is adjustable. The whole device is controlled through the control and power system shown in Figure (e).
The specific composition and operation process of the experimental system are shown in Figure . Before the experiment, the pipe is filled with water and air is injected into the pipe by the air compressor to form air entrapped in the PMMA pipe. The volume of air can be obtained by measuring the height of the air pocket, and adjusted through the dead-end valves and pores. When the air volume reaches the set requirements, the valve on the air transmission pipe is closed to block the fluid channel between the air injection system and the main pipe.
During the experiment, the flow rate of the centrifugal pump and the opening time of the control valve are set according to the working conditions. The centrifugal pump starts when the control valve is closed and runs at the set rotation speed. With the opening of the valve, the water in the tank is injected into the main pipe through the centrifugal pump to form the fluid impact pressure for the entrapped air, and then flows back to the tank through the return pipe. Measuring instruments include flowmeter, dynamic pressure sensor, dynamic signal analyser and camera. The flow rate is measured at a frequency of 50 Hz by an electromagnetic flowmeter with an accuracy of 0.2%. As shown in Figure (b), the dynamic pressure sensor (PCB 112A22, with a linearity of 0.08% FS) is located at the end of PMMA pipe, 0.1 m away from the dead end. The dynamic pressure is collected at a sampling frequency of 40 kHz. Pressure signals are recorded and processed using a dynamic signal analyser (CRYSTAL CoCo-80X). As shown in Figure , a camera is used to record the change of flow state in the whole horizontal PMMA pipe during the experiment. The camera allows a frame rate of 30 fps for a 3840 × 2160 pixels resolution.
In the experiment, the dynamic flow characteristics are studied under 24 working conditions, composed of two kinds of flow rate (3 m3·h−1 and 6 m3·h−1), four kinds of air volume (0.66 × 10−3, 3.6 × 10−3, 6.7 × 10−3 and 11 × 10−3 m3), and three kinds of control valve opening time (1, 2.5 and 5 s).
2.2. CFD model and numerical simulation
According to the designed experimental system and experiment conditions, a CFD model of the pump−pipeline with entrapped air can be established using Fluent® software and the 2D-VOF model so as to carry out a numerical simulation analysis of the transient pressure impact.
2.2.1. Flow path of pump−pipeline system
In the pump−pipeline system, the water in the tank is injected into the main pipe through the centrifugal pump and control valve, forming a fluid impact pressure for the entrapped air, and then flows back to the tank through the return pipe. According to the flow process of the system, the water and entrapped air in the pipe downstream of the control valve can be selected as a fluid domain for numerical simulation, while the water tank, centrifugal pump and control valve constitute the upstream boundary, as shown in Figure , where the relevant physical parameters include the potential head of water level in the tank, HR, the centrifugal pump head, HP, the loss coefficient of the control valve, k, the water velocity at the inlet of the fluid domain, v, the water pressure at the inlet of the fluid domain, Pw, the potential head at the location of entrapped air, HT, and the pressure of entrapped air, P. Thus, the 2D geometric model corresponding to the fluid region in Figure can be established for meshing. It consists of an 8.6 m long horizontal pipe, a 2 m long horizontal PMMA pipe and a vertical connection pipe, as well as an outlet pipe. Considering the flow area consistency of the 2D model and an actual 3D pipe, the diameter of the main pipe in the 2D model is equivalent to 95 mm and that of the outlet pipe is 10.78 mm.
2.2.2. 2D CFD model
Two mesh parameters, namely the global mesh parameter X and the horizontal PMMA pipe mesh parameter Y, are set with three different sizes, respectively, to ensure the accuracy and efficiency of the numerical simulation. The numerical simulation is carried out under the same conditions (flow rate 3 m3·h−1, air volume 6.7 × 10−3 m3, valve opening time 1 s) after corresponding mesh generation. The number of mesh nodes and the maximum peak pressure, Pmax, obtained by numerical simulation are listed in Table in ascending order according to the number of nodes. In Table , i is the number of the mesh parameter combination; is the peak pressure under each i in the simulation;
is the peak pressure in the experiment;
;
. When X = 5 mm and Y = 2 mm, E1 and E2 are small enough to prove the accuracy of the simulation, and there is no significant change in E1 and E2 as the node number increases. This indicates that the simulation data are in good agreement with the experimental results, and that further refinement of the mesh size causes little improvement in the result, but it increases the calculation time significantly. Therefore, a mesh size of X = 5 mm and Y = 2 mm is selected to generate the mesh and form the 2D CFD model as shown in Figure .
Table 1. Mesh independence analysis.
As shown in Figure , the entrapped air is located in the horizontal PMMA pipe and the pressure monitoring point is set at its end. The VOF model provided in Fluent software can simulate two or more immiscible fluids with recognisable interfaces by defining the percentage of each phase in each element. Thus, The VOF model is used to analyse the interaction between air and water in the PMMA pipe.
2.2.3. Boundary conditions and settings of CFD
The inlet boundary of the numerical fluid domain can be defined as the pressure inlet, as shown in Figure , which is related to the water tank, centrifugal pump and control valve, then
(1)
(1) where ρ is the density of fluid and g is the acceleration due to gravity. The Suter curve of the centrifugal pump is applied to describe the general pump behaviour. According to the Suter curve,
(2)
(2) where AP is the closing head of the centrifugal pump, CP is a constant defining the characteristic curve of the centrifugal pump, Q is the flow rate of the centrifugal pump, and S is the cross-sectional area of the pipe. By substituting Equation (2) into Equation (1),
(3)
(3)
According to the study of Izquierdo et al. (Citation1999), the value of k is
(4)
(4) where k0 is the loss coefficient when the valve is fully opened, t is the time, and T is the opening time of the control valve. Equations (3) and (4) describe the relationship between Pw and v when the centrifugal pump operates and the control valve is started. Based on Equations (3) and (4), the inlet boundary of the CFD model is written by the user defined function (UDF) of Fluent and the C language. The main process of UDF writing isas follows.
At the end of each time step of numerical simulation, perform the following steps: (a) obtain the total area of the inlet elements and the sum of the velocities of each element; (b) obtain the average velocity weighted by the area of the inlet elements; (c) assign the average velocity to v in Equation (3) and find Pw.
At the beginning of the next time step of the numerical simulation, assign Pw to the pressure inlet boundary.
The outlet boundary of the 2D CFD model is at constant pressure. A realizable model is used for turbulence. Air is regarded as an ideal gas and water is regarded as a compressible fluid. The surface tension coefficient is set to 0.072 N·m−1. Considering the roughness of the pipe wall, the wall roughness height of the stainless steel pipe is set to 0.046 mm, and that of the PMMA pipe is set to 0.0015 mm. The boundary conditions at the pipe wall are described by a ‘no slip condition’. The flow solver uses the semi-implicit method for pressure-linked equations. Convergence can be evaluated by progressively tracking the residuals at each iteration step. When the residual is less than 10−3, i.e. the convergence standard preset by the iterative solver, the convergence solution is realized. The time step is set according to the Courant number, which is defined as
(5)
(5) where CN is the Courant number, u is the fluid velocity, Δt is the time step and Δx is the mesh element size. To ensure convergence of numerical simulation, CN is set to one, then the time step is Δt = Δx/u. The time step ranges from 10−5∼5 × 10−4 s depending on the different pressure inlets.
According to the principle of equivalent air volume ratio (air volume/pipe volume) with the experiment pipeline, CFD simulations are carried out for four air volume ratios, 1.12%, 4.93%, 10.00% and 16.72%, as shown in Table . In addition to the four air volume ratios, the simulations also consider three steady flow rates (3, 6 and 8.5 m3·h−1) and six valve opening times (0.1, 0.2, 0.5, 1, 2.5 and 5 s) in corresponding combinations, to form a total of 72 kinds of simulation conditions.
Table 2. Initial air volume ratios of the CFD model and experiment.
3. Analysis and discussion of the dynamic flow characteristics of the pump−pipeline system with entrapped air
Based on the above experiments and numerical simulations, the experiment data and CFD simulation results of the transient pressure and flow state under different working conditions are obtained and analysed so as to discuss the dynamic flow characteristics and flow state of the pump−pipeline system with entrapped air.
3.1. Dimensionless parameters
In order to extrapolate the analysis results to general pump−pipeline systems with different hydraulic conditions, the following four dimensionless parameters are defined.
The first dimensionless parameter, λ, was proposed by Martins et al. (Citation2017). It is described by the ratio of wave velocity to the theoretical Torricelli velocity caused by the initial pressure difference. In this research, it is used to characterise the steady flow rate of the system, which can be achieved by adjusting the rotatation speed of the centrifugal pump under a constant pipeline system configuration and parameters.
(6)
(6) where c is the wave velocity, Ps is the steady pressure of the entrapped air, Qs is the steady flow rate of the system, P0 is the initial pressure of the entrapped air, and V = [2(Ps+ρgCpQs2-P0)/ρ]0.5 is obtained by Torricelli's law. When the pipeline system is running stably, the entrapped air is stationary, as shown in Figure :
(7)
(7) where (HP)s is the steady head of the centrifugal pump. Since AP and Qs could vary with the rotation speed of the centrifugal pump, λ can be used to characterise the steady flow rate of the system.
The second dimensionless parameter, α, is used to characterise the entrapped air volume, which is described by the ratio of the initial air volume to the total volume:
(8)
(8) where Vair 0 is the initial volume of entrapped air and Vwater 0 is the initial volume of water in the closed pipe section.
The third dimensionless parameter, τ, represents the opening time of the control valve. It is described by the ratio of the opening time to the time required for the pressure wave to travel from the control valve to the pressure monitoring point:
(9)
(9) where l is the axial distance of the pipeline from the control valve to the pressure monitoring point.
The fourth dimensionless parameter, hm, is the maximum transient overpressure, which is used to describe the extent to which the maximum peak pressure exceeds the steady pressure:
(10)
(10)
Dimensionless parameters corresponding to parameters in different working conditions are shown in Table .
Table 3. Working condition parameters and corresponding dimensionless parameters for the numerical simulation.
3.2. Variable inlet pressure
Based on the CFD model, numerical simulations are carried out in various working conditions. The inlet pressure of different working conditions is given by Equations (3) and (4). The inlet pressure curves of some working conditions are shown in Figure . By comparing the four curves in Figure , it can be seen that λ, α and τ all have an influence on the inlet pressure curves. Different λ could lead to different peak and steady values of the inlet pressure curves, while different τ and α could result in different peak pressures and arrival times to peak pressure, and basically the same steady value of the inlet pressure curves. It can be seen that λ directly affects the inlet pressure through AP from Equations (3) and (6). According to Equations (4) and (9), the inlet pressure curves in the opening process of the control valve could change if τ changes, thus affecting the peak pressure and arrival time. However, k0 is a constant, so the steady values of the inlet pressure curve under different τ are basically the same. The influence of α on the inlet pressure is not directly set by the equations like λ and τ, but α indirectly affects the inlet pressure mainly by influencing the fluctuation period of the entrapped air. As can be seen from Equation (3), the inlet pressure is affected by the fluid velocity, v. Different α could lead to different fluctuation periods of entrapped air, and different fluid velocity v at the same t, thus affecting the inlet pressure. In summary, the transient pressure impact and pressure fluctuation of the pump−pipeline system with entrapped air during start-up is related to the interaction between the variable pressure associated with the pump-valve control and the dynamic characteristics of the entrapped air. The dynamic flow characteristics are affected by the pump flow rate, the opening time of the control valve and the air volume ratio.
3.3. Analysis of the dynamic flow characteristics
Based on the CFD model, the dynamic flow characteristics of the pipeline with entrapped air are numerically calculated under multiple working conditions, and pressure curves at the monitoring point under different dimensionless parameters λ, α and τ are obtained, as shown in Figures , and , in which the pressure curves obtained from RWCM and the fluctuating pressure obtained by experiment are also given. Note that the fluctuating pressure refers to the fluctuating component of the pressure, and the direct current component is excluded.
It is worth noting that pressure fluctuation exists throughout the pipeline during transient events. We set several monitoring points in the experiment and simulation, but it is found that the pressure fluctuation at the monitoring point in Figures , and is the largest and most threatening to operational safety. Therefore, only the pressure fluctuation at this monitoring point is described and discussed for the sake of concise description.
As shown in Figures , and , the pressure curves of RWCM are obviously inconsistent with those of experiment and the CFD model, and the average value of the peak error is 12.25%. Therefore, as described in the introduction, the RWCM has limitations for solving transient flows with entrapped air in horizontal pipes. The pressure curves obtained by numerical simulation are basically consistent with those obtained by experiment, and the main features of the pressure curves, such as the peak pressure and the arrival time to the peak pressure (Tp), are largely consistent. Tables and show hm and Tp obtained from CFD simulation and experiment under all experimental conditions, as well as the errors between simulation and experiment, respectively. It can be seen from Tables and that the errors in hm and Tp are below 10% in most working conditions. Therefore, the CFD model established in this research can simulate effectively the dynamic flow characteristics of pump−pipeline systems with entrapped air during start-up.
Table 4. CFD simulation hm, experimental results and error.
Table 5. Tp of the CFD simulation, experimental results and the error.
According to Figure and Table , the peak pressure increases while hm decreases when λ decreases. That is, larger steady flow rates lead to higher peak pressures, but lower maximum overpressure. A larger steady flow rate requires a larger pump speed and head, leading to a higher inlet pressure of the fluid domain, thus increasing the peak pressure and steady pressure. However, the increase of the steady pressure is larger than that of the peak pressure, which is manifested as a decrease of the maximum overpressure under the working conditions studied in this research.
Figure and Table show that the peak pressure and hm decrease when α increases. This implies that a larger air volume ratio leads to a lower peak pressure and a maximum overpressure, which is consistent with the studies of Izquierdo et al. (Citation1999) and Malekpour (Citation2014). When the initial pressure and steady pressure of air remain constant, a larger air volume could result in a larger variation of air volume and a longer Tp. As the air pressure reaches its peak, water entering the system all flows to the outlet pipe, where the fluid velocity increases with t. Thus, a larger Tp could result in a larger v at Tp and a lower inlet pressure, leading to a lower peak pressure and a maximum overpressure.
Figure 7. Pressure curves under different α (λ = 19.07; τ = 50): (a) α = 1.12%; (b) α = 4.92%; (c) α = 10%; (d) α = 16.72%.

According to Figure and Table , the peak pressure and hm decrease when τ increases. This means that a longer valve opening time could lead to a lower peak pressure and maximum overpressure. According to Equations (3) and (4), when the valve is opened for a longer time, k at the same t in the opening process of the control valve is larger. As a result, the inlet pressure of the fluid domain is lower, leading to a lower peak pressure and a maximum overpressure.
Figure 8. Pressure curves under different τ (λ = 19.07; α = 16.72%): (a) τ = 20; (b) τ = 50; (c) τ = 100.

The influence of λ on Tp is not significant, as can be seen from Figure . As the steady flow rate increases, the inlet pressure increases, resulting in a larger fluid velocity and a larger air volume change. A larger fluid velocity reduces Tp, while a larger air volume change increases Tp. Thus, the effect of λ on Tp is not significant under the working conditions studied in this research. Tp tends to increase with increasing α, i.e. a larger air volume ratio could result in a longer arrival time to peak pressure. This trend can be attributed to Boyle's law, which states that, when the initial pressure and steady pressure remain unchanged, a larger air volume could result in a larger variation of air volume and a longer Tp. Tp increases with increasing τ; namely, a longer valve opening time could result in a longer arrival time. When the valve is opened for a longer time, the k at the same t in the opening process of the control valve is larger, leading to a smaller v. As a result, Tp becomes larger with the variation of air volume unchanged.
The simulation capability of the 2D CFD approach is verified based on the above discussion. The characteristic analysis of the pressure impact can provide support for fault analysis and risk assessment of pump−pipeline systems.
3.4. Analysis of the two-phase flow state in the pipe with entrapped air
In order to understand the transient flow state of the two-phase fluid when the pump−pipeline is started, changes of the air–water flow state in the horizontal PMMA pipe are photographed by camera during the experiment, and the corresponding transient flow state is calculated by the CFD model. By analysing the changes of flow state shown in photos under various working conditions, the transient flow changes can be divided into two types. Type I is shown in Figure , and its typical working condition parameters are: λ = 19.07, α = 10%, τ = 20; Type II is shown in Figure , and its typical working condition parameters are: λ = 9.53; α = 10%; τ = 20. Both types of transient flow processes are described by photos and simulation results. In Figures and , the region is the green area recorded by the camera in Figure . The left-hand side is connected to the upstream pipe, and the right-hand side is the dead end.
Figure 9. The transient flow state changes of Type I in the experiment and the CFD simulation (λ = 19.07; α = 10%; τ = 20). (air


Figure 10. The transient flow state changes of Type II in the experiment and the CFD simulation (λ = 9.53; α = 10%; τ = 20). (air


According to Figures and , the overall change process of the two types can be described as follows.
t = t1 (t1′). After the control valve is opened, the water in the upstream pipe is impacted and enters the horizontal PMMA pipe along the bend, causing the water surface on the left-hand side to rise. The air–water interface of t1 is clear, while the left air–water interface in the experimental photo of t1′ is broken, and that of the simulation results is clear. The air is compressed and the air pressure begins to increase.
t = t2∼t4 (t2′∼t4′). The water in the upstream pipe continues to enter the horizontal PMMA pipe through the bend, pushing the surging water to occupy the entire section of the left-hand side, and forming the first surge, which is constantly moving forward. The air–water interface of t2∼t4 is clear, but the air–water interface on the left-hand side of t2′∼t4′ is broken. The air–water mixing phenomenon appears in the experimental photos and simulation results of t2′∼t4′. The air is continuously compressed, and the air pressure gradually increases, reaching its peak when t = t4 (t4′).
t = t5∼t6 (t5′∼t6′). The first surge moves forward, and its height is reduced by gravity and air pressure, and the water continues to form subsequent surges. The air–water interface tends to be completely clear. The water experiences an air intrusion at the pipe crown, and the air pressure decreases.
t = t7 (t7′). The first surge hits the dead end, and the water is kicked up by inertia, hitting the pipe crown. The air pressure is basically steady. The water in the upstream pipe flows to the outlet pipe and no longer enters the PMMA pipe.
t = t8 (t8′). After the first surge hits the dead end, it reverses direction and moves in the opposite direction as the subsequent surge.
t = t9 (t9′). When the two surges collide, the water arches and impacts the pipe crown. The air–water interface is partially broken and droplets splash.
t = t10 (t10′). The two surges are separated and run away from each other. The left-hand surge hits the subsequent surge, and the right-hand surge hits the dead end. The above impact process is repeated until the air–water interface is stationary.
By comparing the experimental photos and the CFD model results at different time points, the CFD model results are basically consistent with the experimental photos when the air–water interface is clear. When the air–water interface is broken (t1′∼t4′) in the experiment photos, the CFD simulation results can show part of the air–water mixing phenomenon, but it still tends to be clear air–water interface. It is not completely consistent with the experimental photos, which is caused by the limitations of the 2D VOF model and simulation conditions, but the CFD model can still in general display the subsequent changes of the air–water interface (t5′∼t10′). Hence the 2D-VOF CFD model can generally simulate the transient flow changes of air and water when the pump−pipeline system with entrapped air is started.
The time points of the experimental photos and simulation results in Figures and are marked on the air pressure curves under the corresponding working conditions, as shown in Figure . It is found that t1∼t4 (t1′∼t4′) is the process in which water continuously compresses air, making the air pressure gradually rises to its peak. t5∼t10 (t5′∼t10′) is the process in which water no longer enters the horizontal PMMA pipe, and the air pressure gradually becomes steady. t1∼t4 (t1′∼t4′) is the key process for studying the peak pressure and peak time of entrapped air. The main difference between the two types is in this process.
By comparing the experimental photos for t1∼t4 and t1′∼t4′, the air–water interface on the left-hand side remains clear and steady in the process of t1∼t4, and there is no obvious air–water mixing phenomenon; while in the process of t1′∼t4′, the air–water interface on the left-hand side is partly broken, with droplets splashing and bubbles entering the water, and there is an obvious air–water mixing phenomenon. By comparing the working condition parameters of the two types, it can be seen that the λ of Type II is smaller. The flow rate and the upstream pressure are larger when the valve is opened, and the water flowing into the horizontal PMMA pipe is more likely to break through the air–water interface. By comparing the experimental photos under other working conditions, it is found that τ also affects the stability of the air–water interface. A smaller τ makes the water flow into the horizontal PMMA pipe and break the air–water interface more easily. α has no significant effect on the stability of the air–water interface.
The movement of entrapped air is basically understood through experiments and CFD based on the above analysis. A more intuitive interpretation of the transient pressure impact with entrapped air is understood by connecting the movement of the air–water interface with pressure information. The flow field information of CFD provides the basis for further study and interpretation of the air motion behaviour.
4. Calculation equation of the peak pressure in the pump−pipeline system with entrapped air
On the basis of the above study of the influence of flow rate, air volume and valve opening time on peak pressure, through the statistical analysis of a large number of simulation results, a calculation equation for the maximum overpressure hm in terms of λ, α and τ can be obtained:
(11)
(11) where A and B are functions of α and τ. A = 5ατ+385α+5τ−187, and B = 2ατ+11,224α+176τ−15,329.
Equation (11) is the equation of peak pressure based on the simulation results of the CFD model, and describes the quantitative relationship between the dimensionless parameters λ, α, τ and hm. The quantitative effects of steady flow rate, air volume and valve opening time on peak pressure can be analysed by this equation. Equation (11) can be used to calculate the peak pressure of a pump−pipeline system with entrapped air during start-up. Figure shows the curve of hm with the parameters λ, α, and τ according to Equation (11), as well as the CFD simulation results.
Figure 12. Comparison of the CFD model and the calculation equation presented in this research: (a) λ = 19.07; (b) λ = 9.53; (c) λ = 7.01.

Table shows the errors between Equation (11) and the experimental data. It can be seen that the errors are mostly less than 10%, which reflects the consistency of the overall data. It can also be seen that the average error is the largest when α = 1.12%, which we believe is caused by limitations of the 2D model. This formula is more suitable for the working condition where α is greater than 1.12%. When α is less than 1.12%, which is closer to the water hammer, a conservative conclusion can be obtained by using the corresponding single-phase water hammer calculation formula.
Table 6. Error between the experiment data and the calculation equation presented in this research (%).
5. Conclusions and improvements
When the pump−pipeline system with entrapped air is started, the entrapped air could induce pressure impact and fluctuation, which may pose a threat to pipeline safety. In this research, an experimental platform and a 2D-VOF CFD model are established, and the transient characteristics of a pump−pipeline with entrapped air during start-up are studied by experimental and numerical approaches. The main conclusions are as follows:
The CFD simulation and experimental results have a good consistency in both the pressure curves and the flow state, which verifies the rationality of the 2D-VOF CFD model used to simulate the dynamic flow characteristics of a pump−pipeline system with entrapped air during start-up.
The transient pressure impact and pressure fluctuation during pump−pipeline start-up are related to the interaction between the variable pressure conditions associated with the pump-valve control and the dynamic characteristics of the entrapped air. Transient pressure characteristics are affected by flow rate, control valve opening time and air volume ratio.
Lower peak pressure can be achieved at a smaller flow rate, larger air volume ratio and longer valve opening time; longer arrival time can be achieved at larger air volume ratio and longer valve opening time.
Two types of air–water interface, a clear interface and a broken interface, exist in the process of air pressurization to the peak pressure. As the flow rate rises and the valve opening time decreases, the air–water interface becomes more fragile.
Based on the results of the 2D-VOF CFD model, a calculation equation with respect to the dimensionless parameters is proposed for prediction of the dynamic characteristics of the pump−pipeline system with entrapped air during start-up. The formula is more suitable for working conditions where the air volume ratio is greater than 1.12%.
Accurate pressure results and abundant flow information are obtained through experiments and simulations in this article, which promotes the study of transient flow in a pump−pipeline system with entrapped air. Some work can be done to advance the research. Based on the motion phenomena of the air–water interface obtained in experiments and simulations, the dynamic of the air–water interface can be studied further and explained. The transient flows under variable pressure of more complex and longer pipeline systems with entrapped air could be studied, and a more accurate and efficient calculation method could be explored by comparing and combining the VOF model with 1D models.
Notation
A | = | function (−) |
Ap | = | closing head of centrifugal pump (m) |
B | = | function (−) |
c | = | wave velocity (m·s−1) |
CN | = | Courant number (−) |
CP | = | characteristic curve constant of the centrifugal pump (−) |
g | = | acceleration due to gravity (m·s-²) |
hm | = | dimensionless maximum transient overpressure (−) |
Hp | = | head of centrifugal pump (m) |
(Hp)s | = | steady head of centrifugal pump (m) |
HR | = | potential head of water level in the tank (m) |
HT | = | potential head of water level at the location of entrapped air (m) |
i | = | the number of the mesh parameter combination |
k | = | loss coefficient of the control valve (−) |
k0 | = | loss coefficient of the fully opened control valve (−) |
l | = | axial distance of the pipeline from the control valve to the pressure monitoring point (m) |
P | = | pressure of entrapped air (kPa) |
P0 | = | initial pressure of entrapped air (kPa) |
= | peak pressure in the experiment (kPa) | |
Pmax | = | maximum peak pressure of entrapped air (kPa) |
Ps | = | steady pressure of entrapped air (kPa) |
= | the peak pressure under each i in the simulation (kPa) | |
E1 | = | the error of |
E2 | = | the error of |
Pw | = | pressure at the inlet of the fluid domain (kPa) |
Q | = | flow rate (m3·s−1) |
Qs | = | steady flow rate (m3·s−1) |
S | = | cross-sectional area of the pipe (m2) |
t | = | time (s) |
T | = | opening time of the control valve (s) |
Tp | = | arrival time to the peak pressure (s) |
u | = | fluid velocity (m·s−1) |
v | = | fluid velocity at the inlet of the fluid domain (m·s−1) |
V | = | theoretical Torricelli velocity (m·s−1) |
Vair0 | = | initial volume of entrapped air (m3) |
Vwater0 | = | initial volume of water in the closed pipe section (m3) |
X | = | global mesh parameter (mm) |
Y | = | horizontal PMMA pipe mesh parameter (mm) |
Δt | = | time step (s) |
Δx | = | mesh element size (mm) |
α | = | dimensionless entrapped air volume (−) |
ρ | = | density of water (kg·m−3) |
λ | = | dimensionless steady flow rate (−) |
τ | = | dimensionless opening time of the control valve (−) |
Acknowledgements
The authors thank China Nuclear Power Engineering Co. Ltd for their help with the experiment.
Disclosure statement
No potential conflict of interest was reported by the authors.
References
- Chaudhry, M. H. (1979). Applied hydraulic transients (2nd ed.). Van Nostrand Reinhold.
- Fuertes, V., Cabrera, E., Izquierdo, J., & Iglesias, P. (1999). Peak pressure evaluation in pipelines with entrapped air pockets. Proc., 3rdASME/JSME Joint Fluids Engineering Conf., ASCE, New York.
- Gu, Z., Bai, C., & Zhang, H. (2021). Nonlinear dynamic modeling and fluid–mechanism interaction analysis of reciprocating pump–pipeline system. Proceedings of the Institution of Mechanical Engineers, Part I: Journal of Systems and Control Engineering, 235(6), 869–880. https://doi.org/10.1177/0959651820965108
- Gu, Z., Bai, C., & Zhang, H. (2022). Dynamic modeling of reciprocating pump valves with considering the interaction effects between pump and pipeline. Proceedings of the Institution of Mechanical Engineers, Part I: Journal of Systems and Control Engineering, 236(8), 1491–1502. https://doi.org/10.1177/09596518221098294
- Hargreaves, D. M., Morvan, H. P., & Wright, N. G. (2007). Validation of the volume of fluid method for free surface calculation: The broad-crested weir. Engineering Applications of Computational Fluid Mechanics, 1(2), 136–146. https://doi.org/10.1080/19942060.2007.11015188
- He, J., Hou, Q., Lian, J., Tijsseling, A. S., Bozkus, Z., Laanearu, J., & Lin, L. (2022). Three-dimensional CFD analysis of liquid slug acceleration and impact in a voided pipeline with end orifice. Engineering Applications of Computational Fluid Mechanics, 16(1), 1444–1463. https://doi.org/10.1080/19942060.2022.2095440
- Huang, B., & Zhu, D. Z. (2021). Rigid-column model for rapid filling in a partially filled horizontal pipe. Journal of Hydraulic Engineering, 147(2), 06020018-1–06020018-9. https://doi.org/10.1061/(ASCE)HY.1943-7900.0001849
- Izquierdo, J., Fuertes, V. S., Cabrera, E., Iglesias, P. L., & Garcia-Serra, J. (1999). Pipeline start-up with entrapped air. Journal of Hydraulic Research, 37(5), 579–590. https://doi.org/10.1080/00221689909498518
- Malekpour, A. (2014). Analysis of rapid pipeline filling including column separation & entrapped Air effects [PhD thesis]. University of Toronto. https://www.researchgate.net.
- Martin, C. S. (1976). Entrapped air in pipelines. Proc. Of the second international conference on pressure surges. London, England.
- Martins, N. M. C., Delgado, J. N., Ramos, H. M., & Covas, D. I. C. (2017). Maximum transient pressures in a rapidly filling pipeline with entrapped air using a CFD model. Journal of Hydraulic Research, 55(4), 506–519. https://doi.org/10.1080/00221686.2016.1275046
- Martins, S. C. (2012). Pressurization dynamics of hydraulic systems with entrapped air [Portuguese] [PhD thesis]. Instituto Superior Técnico, Lisbon, Portugal. https://www.researchgate.net.
- Nikpour, M. R., Nazemi, A. H., Dalir, A. H., Shoja, F., & Varjavand, P. (2014). Experimental and numerical simulation of water hammer. Arabian Journal for Science & Engineering, 39(4), 2669–2675. https://doi.org/10.1007/s13369-013-0942-1
- Pozos-Estrada, O., Fuentes-Mariles, O. A., & Pozos-Estrada, A. (2012). Gas pockets in a wastewater rising main: A case study. Water Science and Technology, 66(10), 2265–2274. https://doi.org/10.2166/wst.2012.462
- Pozos-Estrada, O., Sánchez-Huerta, A., Breña-Naranjo, J. A., & Pedrozo-Acuña, A. (2016). Failure analysis of a water supply pumping pipeline system. Water, 8(9), 395. https://doi.org/10.3390/w8090395
- Vasconcelos, J., & Wright, S. (2009). Investigation of rapid filling of poorly ventilated stormwater storage tunnels. Journal of Hydraulic Research, 47(5), 547–558. https://doi.org/10.3826/jhr.2009.3390
- Vasconcelos, J., & Wright, S. J. (2004). Numerical modeling of the transition between free surface and pressurized flow in storm sewers. Journal of Water Management Modeling, 220(10), 188–214. https://doi.org/10.14796/JWMM.R220-10
- Wan, W., Zhang, B., & Chen, X. (2018). Investigation on water hammer control of centrifugal pumps in water supply pipeline systems. Energies, 12(1), 1–20. https://doi.org/10.3390/en12010108
- Wright, S. J., Determan, K. V., & Vargas, S. M. (2012). Pressure transients due to compression of trapped air in rapidly filling sewer storage tunnel. On Modeling Urban Water Systems—Monograph, 20, CHI Press, Guelph, ON, 2–18. https://doi.org/10.14796/JWMM.R245-01
- Wylie, E. B., Streeter, V. L., & Suo, L. (1993). Fluid transient in systems. Prentice-Hall.
- Zhang, B., Wan, W., & Bi, L. (2020). Improvement on simulation methods of fluid transient processes in turbine tailrace tunnel. Journal of Pressure Vessel Technology, 143(3), 031403-1–031403-8. https://doi.org/10.1115/1.4048325
- Zhou, F., Hicks, F. E., & Steffler, P. M. (2002). Transient flow in a rapidly filling horizontal pipe containing trapped air. Journal of Hydraulic Engineering - ASCE, 128(6), 625–634. https://doi.org/10.1061/(ASCE)0733-9429(2002)128:6(625)
- Zhou, L., Liu, D., Karney, B., & Zhang, Q. (2011). Influence of entrapped air pockets on hydraulic transients in water pipelines. Journal of Hydraulic Engineering, 137(12), 1686–1692. https://doi.org/10.1061/(ASCE)HY.1943-7900.0000460
- Zhou, L., Liu, D. Y., & Ou, C. Q. (2011). Simulation of flow transients in a water filling pipe containing entrapped air pocket with VOF model. Engineering Applications of Computational Fluid Mechanics, 5(1), 127–140. https://doi.org/10.1080/19942060.2011.11015357