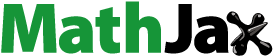
Abstract
Tangential intake structures with vertical dropshafts are commonly employed in stormwater and urban drainage systems. Vortex and plunging flows are the two typical flow patterns observed in dropshafts, with the former being the desired due to its favourable drainage performance and enhanced safety. The transition between these flow patterns is primarily influenced by the structural design and rate of flow discharge. To investigate the interception performance and the transition criteria between vortex and plunging flows, a series of numerical simulations were conducted. The effects of the approach channel slope, contraction ratio, and flow discharge were analyzed by comparing the evolution of the water surface, pressure and velocity distributions, and swirling distance across various scenarios. With a higher flow rate of 20 L/s, obvious water congestion was observed in dropshafts with contraction ratios below 0.4 and horizontal approach channels. However, the inflow channel was not congested in cases with sloping approach channels, indicating higher drainage efficiency of intake structures with slopping channels. Two hydraulic factors, namely, the minimum to maximum wall pressure ratios (Pmin/Pmax) and the minimum Vr/Vz ratios (Vr/Vz)min, were employed to differentiate between vortex flow and nonrotational flow. These two factors consistently led to the same conclusions under both subcritical and supercritical inflow conditions, indicating their validity and their potential application in the design and optimization of intake structures. The criteria of Pmin/Pmax > 0.2 and (Vr/Vz)min > 0.2 can be used as criteria for the formation of stable vortex flow.
1. Introduction
The process of urbanization has resulted in a gradual reduction in permeable areas in cities. Additionally, the effects of global climate change have led to an increased frequency of extreme rainfall events. Consequently, urban flooding and runoff pollution have emerged as significant hazards to public safety and the local environment (Jianyun et al., Citation2016). To address urban flooding issues, the adoption of deep tunnel stormwater systems is increasingly common in larger cities. These systems quickly transport surface runoff into an underground network (Yu & Lee, Citation2009; Chang & Wei, Citation2022a). Nevertheless, the rapid drop in elevation and interception of entrapped air in the tunnel may result in scouring, cavitation erosion, and flow choking. Therefore, the design of intake structures plays a crucial role in the overall performance of the water discharge system (Chan & Qiao, Citation2022; Chang & Wei, Citation2022b).
The intake structure and dropshaft serve as critical elements in deep tunnel systems, as they collect stormwater from the surface and direct it into underground tunnels. Various types of dropshafts are available, including plunging dropshafts, vortex dropshafts, baffle dropshafts, and helicoidal-ramp dropshafts, each with a unique design structure. Plunging and vortex dropshafts are commonly utilized due to their straightforward geometry and high flow discharge capacity (Sun et al., Citation2023). In a plunging dropshaft, the flow collides with the sidewall, resulting in significant air entrainment (Rajaratnam et al., Citation1997; Jianyun et al., Citation2016). The entrapment of air can lead to a reduced flow interception rate and give rise to environmental concerns such as sewer odour and gas geysers (Wang et al., Citation2023; Zhang et al., Citation2022). The energy of the incoming flow is mainly dissipated through impact with the dropshaft wall and intense interaction with the entrapped air. However, this dissipation may not be sufficient and could potentially result in cavitation erosion and scouring.
Vortex dropshafts featuring tangential intake structures are extensively employed in water supply, drainage, and sewerage systems due to their compact design and efficient conveyance of water from higher to lower elevations (Yu & Lee, Citation2009; Chang & Wei, Citation2022b). A vortex dropshaft typically comprises an approach flow channel and a sloped tapering section that connects to a vertical dropshaft. The tangential connection between the approach flow channel and the vertical dropshaft allows the flow to spiral downward along the inner wall of the dropshaft, generating an air core at its centre (Kan et al., Citation2023; Shen et al., Citation2022). Compared to plunging dropshafts, the flow diversion is smoother, and energy is more effectively dissipated due to the wall friction and spiral flow turbulence. In comparison to plunging dropshafts, this spiral flow promotes smoother flow diversion and more effective energy dissipation through wall friction and turbulence. The hydraulic behaviour of a tangential vortex intake structure is heavily influenced by both the structural design and incoming flow conditions. The combined influence of the design structure and inflow conditions can lead to various flow patterns within the dropshaft, including plunging, transitional, or vortex flow patterns.
Extensive research has been conducted utilizing experimental, theoretical, and numerical studies to explore the flow characteristics of tangential vortex dropshafts, including the transition between plunging and vortex flows. Yu and Lee (Citation2009) conducted a series of laboratory experiments using tangential intake structures and identified the width of the tangential intake contraction at the dropshaft junction as a critical factor. Their study provided valuable design guidelines for achieving smooth water flow in tangential vortex intakes. Ma (Citation2016) further investigated the transition between plunging and vortex dropshaft flows and proposed theoretical models based on experimental findings to establish design guidelines for stable vortex flows in dropshaft structures (Wei & Chang, Citation2023; Yu & Lee, Citation2009). Numerical models, particularly three-dimensional (3D) numerical models, have been employed to gain a detailed understanding of flow characteristics within dropshaft structures. Researchers such as Plant and Crawford (Citation2016) and Chang and Wei (Citation2022b) have utilized these models to investigate key factors associated with flow transitions, including the transition between plunging and vortex flows. While subcritical and supercritical inflow conditions have been separately studied (Pfister et al., Citation2018; Zhang et al., Citation2009), further investigation is required to comprehensively understand the distinct performance of subcritical and supercritical inflow conditions.
This study aims to investigate flow transitions in dropshaft structures under both subcritical and supercritical flow conditions. To achieve this, a series of three-dimensional (3D) computational fluid dynamics (CFD) models are utilized, incorporating different contraction ratios to simulate the effect of structure design under subcritical and supercritical inflow conditions. The subcritical inflow condition is achieved by employing horizontal approach channels, while a slope of 1:10 is used for the approach channels to generate the supercritical inflow condition. The study analyzes the wall pressure and velocity distribution to examine the influence of the contraction ratio and inflow condition on flow performance. Furthermore, the study seeks to identify the optimal hydraulic factors that can be employed to determine the suitable performance of tangential intake structures in vortex dropshafts under various flow discharge conditions.
2. Computational Fluid Dynamics model
2.1. Model setup
The numerical models were developed based on a physical prototype with a dropshaft diameter D and an inflow channel width of 0.24 m (Figure ). The flow characteristics within the dropshaft were primarily determined by the tangential intake design parameters, which included the dropshaft diameter D, the contraction width e, and the tapering section slope β. The original design featured a horizontal approach channel and a tapering section with a constant bottom slope (β) of 13° between the chute and the dropshaft. An additional numerical design was developed with a bottom slope of 1:10 (5.71°) in the approach channel, while the tapering section maintained the original slope. Various contraction widths with contraction ratios (e/D) ranging from 0.1 to 0.8 were tested to investigate the impact of the tangential intake design on the flow pattern within the dropshaft. Flow simulations were conducted within the tunnel system with flow discharges (Q) ranging from 5 L/s to 40 L/s. (Table )
Table 1. Nomenclature.
2.2. Governing equations
The current investigation utilized the VOF technique in conjunction with the k-ϵ turbulence model to simulate stratified air–water flow and evaluate the requisite air discharge. The governing equations are presented below:
Continuity equation:
(1)
(1) Momentum equation:
(2)
(2) k equation:
(3)
(3) ϵ equation:
(4)
(4) where ρ and μ are the average density of the volume fraction and the molecular viscosity, respectively; p is the pressure; and μt is the turbulent viscosity, which can be deduced from the turbulence intensity k and energy dissipation rate ϵ as:
(5)
(5) The detailed values are shown in Table . The governing equations for the water fraction αw are:
(6)
(6)
(7)
(7)
(8)
(8) where ui is the velocity component and xi is the coordinate (i = 1, 2, 3). The air–water interface was tracked by solving the continuity equations of multiple species. The sum of the water and air volume fractions was 1 in one computational cell. ρw and ρa are the densities of water and air, respectively, and μw and μa are the viscosities of water and air, respectively. The parameters of the governing equations can be found in Chang and Wei (Citation2022b).
Table 2. Inflow condition parameters of simulated scenarios
2.3. Model grid, boundary conditions and other computational details
The present study employed the commercial software ANSYS Fluent to numerically solve the governing equations of fluid dynamics. The Reynolds-averaged Navier–Stokes (RANS) equations were employed to simulate the turbulent flow, using the renormalization group (RNG) k-ϵ model to describe the turbulence characteristics. To simulate the wall boundary conditions, standard wall functions were implemented. The implicit volume-of-fluid (VOF) method was utilized to track the interface between water and air flow, which was proven to provide highly accurate simulation results in previous studies (Chau & Jiang, Citation2004; Yang et al., Citation2018).
The computational domain was discretized into unstructured meshes consisting of hexahedral cells for the approach channel and dropshaft and triangular-prismatic cells for the tapering section. The cell size varied from 1 mm close to the wall to approximately 50 mm along the approach channel and vertical dropshaft. The computational domain was composed of a total of 106,990 unstructured hexahedral cells (Figure ), with a minimum grid size of 1 mm close to the tapering section and walls. A typical time step of 0.002 s was utilized in all simulations, and a steady state was reached after approximately 10 s. The computations were performed on an Intel i7-4790 3.6 GHz CPU computer with quad-core parallel computation, and the simulation took approximately 20 h to complete. The predicted velocities at the junction point of the dropshaft and tapering section (Point B1 in Figure (a)) were within 1% as the cell number increased from 106,990 to 152,958.
Figure 2. Numerical model of the tangential vortex intake design with (a) horizontal approach channel and (b) slopping approach channel (1:10 slop).

The inflow discharge was introduced at the inlet section of the approach channel. At the top of the approach channel and the top and bottom of the dropshaft were open to the atmosphere, the pressure-outlet boundary condition was used, and one standard atmospheric pressure was introduced. For the solid wall boundary condition, a no slip solid boundary condition was applied, and the velocity on the solid wall was set to zero. The validation of the model is performed in previous studies of this series of numerical simulations (Chang & Wei, Citation2022a, Citation2022b). Detailed documentation of the governing equations and quantitative comparisons are available below.
2.4. Model validation
The validity of the CFD scheme was conducted by comparing the water depth in the intake structure and the wall pressure at the dropshaft wall with experimental data (Figures and ). The experiments were performed in the State Key Laboratory of Hydraulic and Mountain River Engineering, Sichuan University. The wall pressure was measured by a series of piezoresistive pressure sensors (CY201, Test, Inc., China) distributed along the cross-section of the dropshaft and an acquisition device (RS485-20). The pressure sensor had an accuracy of 0.1% relative to the 100 kPa full scale, and the acquisition system enabled multiple sensors to sample concurrently at a maximum frequency of 1,000 Hz. Prior to each test, the pressure measurement system underwent calibration through a hydrostatic operation to ensure the reliability of measurements.
Figure 3. The comparison of predicted and observed water depth in approach channel with inflow rates of 10 and 20L/s.

Figure 4. The comparison of predicted and observed wall pressure distributions along the cross-section 10 cm lower than the junction point (Point B1 in Figure ) of tapering section and dropshaft.

Figures and show good agreements between the measured and predicted water depths and pressure distribution data. The relative root mean square error (RMSE) between all measured and predicted data could then be evaluated as follows:
(9)
(9) where
is the predicted value,
is the observed value, and n is the number of observations. The RMSE values of the predicted and measured water depths under 10 and 20 L/s flow rates were within 0.015 and 0.018 m, respectively. The RMSEs between the predicted and measured wall pressures were 124 and 76 Pa, respectively. Considering the possible systematic error and human error during the measurement, the CFD model had good accuracy and could be used to simulate the flow in both the approach channel and dropshafts.
3. Results
3.1. Water surface elevation
At an inflow rate (Q) of 20 L/s and a water depth (d) of 0.0891 m introduced at the start of the approach channel (x = 2.4 m), the water surfaces exhibited varying changes in both the approach channel and tapering section based on the e/D ratios. In cases with a horizontal approach channel, a contraction ratio of 0.7–0.5 allowed for the maintenance of water depth in the approach channel until the end of the channel (Figure (a)). However, after the contraction, the water depth rapidly dropped due to the slope of the tapering section. For the case with an e/D ratio of 0.5, a slight rise in the water surface occurred near the inlet of the dropshaft. As the e/D ratio decreased to 0.4, the change point of the water surface elevation moved upstream before the junction of the tapering section and approach channel. With a further decrease in the e/D ratio to 0.25, the water depth in the approach channel rose above the initially introduced 0.2 m at the inlet, reaching a level of 0.25 m. Consequently, the water interception rate decreased due to water blockage in the approach channel.
Figure 5. Water surface along the approach channel and tapering section with inflow flow rate Q of 20 L/s. (a) Horizontal approach channel; (b) Sloping approach channel (1:10).

In structures with a sloping approach channel (with a 1:10 slope), no water blockage was observed in the approach channel for all e/D ratios ranging from 0.25 to 0.7 (see Figure (b)). The continuous conversion of potential energy into kinetic energy led to an increase in flow velocity along the approach channel, accompanied by a gradual decrease in the thickness of the water surface from the entrance to the tapering section. For contraction ratios of 0.7 and 0.5, the water levels continued to decrease in the tapering section. However, when the e/D ratio was reduced to 0.4 and 0.25, a significant increase in the water level was observed in both the dropshaft and tapering sections. Moreover, the water jump height surpassed that of structures with a horizontal approach channel. Nevertheless, this hydraulic jump did not cause the water level in the approach channel to rise and therefore did not impact drainage efficiency. However, it could potentially lead to excessive mixing of air in the dropshaft, which may result in explosive eruptions of the air–water mixture.
The influence of the inflow flow rate on the water surface elevation was examined for the structure with an e/D ratio of 0.25, and the findings are depicted in Figure . In the intake structure with a horizontal approach channel (Figure (a)), the water depth in the approach channel rapidly increased from 0.15 m to 0.48 m as the flow rate increased from 5 L/s to 40 L/s. Conversely, in cases with a sloping approach channel (Figure (b)), the hydraulic jump above the tapering section progressively moved upstream with higher flow rates. At a flow rate of 40 L/s, the jump height reached as high as 0.45 m.
3.2. Pressure distribution
To distinguish between rotational and nonrotational operations of the dropshaft, the wall pressure was utilized as a classification factor. We conducted an analysis of the pressure distribution along the dropshaft wall for different contraction ratios (e/D) and inflow rates (Q). Figure presents the circular pressure distribution at a cross-section located 0.1 m below the end of the tapering section (z = −0.1 m) for a contraction ratio of 0.25 and flow rates of 5, 10, 20, and 40 L/s.
Figure 7. Circular pressure distribution at cross-section z = −0.1 m (e/D = 0.25). (a) Horizontal approach channel; (b) Sloping approach channel (1:10).

Under identical inflow conditions, the structure with a horizontal approach channel exhibited higher maximum pressure on the dropshaft wall; however, this pressure quickly declined after reaching its peak (Figure (a)). Conversely, in the dropshaft with a sloping approach channel, the circular pressure difference indicated a stronger rotational flow, and the pressure remained more stable across the cross section (Figure (b)). The pressure distribution at cross-Section z = −0.1 m was analyzed for various contraction ratios, as depicted in Figure , using an inflow rate of 20 L/s. In the structure with a horizontal approach channel, cases with e/D ratios of 0.25 and 0.4 exhibited stronger swirling flow, as evidenced by sustained pressure up to a rotation angle of 120 degrees. Conversely, when the e/D ratio increased to 0.5, the rotation weakened, and the pressure dropped rapidly after a rotation distance of 75 degrees. Furthermore, for an e/D ratio of 0.7, the pressure suddenly increased followed by a drop, indicating the presence of plunging flow and the failure of rotational flow.
Figure 8. Circular pressure distribution variation with e/D ratio at cross-section z = −0.1 m (Q = 20 L/s). (a) Horizontal approach channel; (b) Sloping approach channel (1:10).

Similar trends were observed in structures with sloping approach channels, with clear evidence of plunging flow in cases with an e/D ratio of 0.7. The peak pressure in the dropshaft reached 1400 Pa and 1800Pa for the horizontal and sloping cases, respectively, when the e/D ratio was 0.7. Such high pressures can potentially lead to erosion of the dropshaft.
The vertical pressure distributions along the dropshaft wall at rotation angles of 0, 90, 180, and 270 degrees are presented in Figure for flow rates of 5 and 20 L/s. For cases with a smaller flow rate of 5 L/s, structures with sloping approach channels exhibited higher maximum pressures after all rotation angles. Additionally, the maximum pressure points in sloping cases occurred earlier than those with a horizontal approach channel. However, in cases with a flow rate of 20 L/s, especially in the first 90 degrees of rotation, the pressure differences were less noticeable. Clear differences became apparent after 180 degrees. Furthermore, the sustained pressure distance was longer in cases with higher flow rates. The vertical pressure distribution indicated that rotational flow easily formed and was sustained in sloping cases with higher flow rates.
3.3. Velocity distribution
The velocity ratio distribution (Vr/Vz) is another factor that can be used to analyze the flow rotational performance of the dropshaft. We examined the velocity distribution along the dropshaft wall in structures with various e/D ratios and incoming flow rates. Figure illustrates the circular Vr/Vz distribution at a cross section where z = −0.1 m with an e/D ratio of 0.25.
Figure 10. Circular Vr/Vz distribution at cross-section z = −0.1 m (e/D = 0.25). (a) Horizontal approach channel; (b) Sloping approach channel (1:10).

For the intake structure with a horizontal approach channel, the maximum Vr/Vz ratios in all four cases, with flow rates ranging from 5 to 40 L/s, were nearly equal to 1.0. However, the maximum Vr/Vz ratio occurred later with increasing flow rate. After a 180-degree rotational angle, the Vr/Vz ratio decreased for flow rates of 5 and 10 L/s, while no such decrease was observed in higher flow rate cases, indicating stronger rotation of the flow. In structures with a sloping approach channel, the Vr/Vz ratios were larger than 1.0 for smaller flow rates (5 and 10 L/s), and they exceeded 1.5 for higher flow rates after a 90-degree rotational distance. Similar to the results of the pressure distribution, the rotational effect was stronger in cases with a larger flow rate and sloping approach channel. These findings suggested that the Vr/Vz ratio could be used as an indicator of flow rotation in dropshafts, and it demonstrated the influence of flow rates and e/D ratios on rotational behaviour.
With the same flow rate of 20 L/s and different contraction ratios, the resulting Vr/Vz ratios in dropshaft structures with horizontal and sloping approach channels are presented in Figure . The circular Vr/Vz distribution at the cross-section z = −0.1 mm did not change much with the e/D ratio in the dropshaft with the horizontal approach channel (Figure (a)), whereas significant differences were observed in the structure with the slopping approach channel (Figure (b)). In the sloping case, the Vr/Vz ratio increased from 1.2 to 2.0 when the rotation angle increased from 30 degrees to 180 degrees in the dropshaft with e/D = 0.25. The Vr/Vz values dropped quickly from e/D = 0.25 to 0.4 and 0.5, but the ratio values remained larger than 0.5 across the section. However, when e/D increased to 0.7, the rotational strength was weaker when Vr/Vz was below 0.5 with a rotational angle of 30-115°. The failure of the rotation in cases with a larger e/D ratio in the structure with a sloping channel may have been influenced by the larger vertical velocity that developed along the sloping channel.
Figure 11. Circular Vr/Vz distribution with e/D ratio at cross-section z = −0.1 m (Q = 20L/s). (a) Horizontal approach channel; (b) Sloping approach channel (1:10).

The vertical distributions of Vr/Vz in the dropshaft with an e/D ratio of 0.25 and inflow rates of 5 and 20 L/s are presented in Figure for the two types of intake structures. At a flow rate of 5 L/s, the maximum Vr/Vz values were greater in structures with sloping channels than those with horizontal channels. Specifically, the maximum Vr/Vz ratio could exceed 60 in the sloping case at a rotational angle of 0 degrees, but it decreased to below 5 after a 90-degree rotation. However, with a larger flow rate of 20 L/s, the maximum Vr/Vz ratio was observed at locations with a 90-degree rotation, and the value exceeded 1000, indicating flow stagnation at that point (almost zero vertical velocity). The occurrence of the flow stagnation point was delayed in sloping cases compared to horizontal cases, which was attributed to the development of a larger vertical velocity component in the sloping channel.
3.4. Swirling distance
For the purpose of estimating the swirling distance of flow within the dropshaft, the pressure ratio Pmin/Pmax and velocity ratio (Vr/Vz)min have been utilized (Chang & Wei, Citation2022a, Citation2022b). Figure (a) and (b) present the Pmin/Pmax ratio along the cross section at different drop distances z/D in dropshafts with horizontal and sloping approach channels, respectively. In both cases, the Pmin/Pmax ratio increased with increasing flow rate, with dropshafts featuring a sloping approach channel exhibiting a higher ratio. Using a Pmin/Pmax threshold of 0.2 as a criterion for hydraulic assessment of stable vortex flow (Chang & Wei, Citation2022), vortex flow failed to develop in dropshafts with horizontal approach channels for flow rates of 10 and 20 L/s. However, in both design structures, vortex flow was sustained for a distance of at least z/D = 7 under a flow rate of 40 L/s. In dropshafts with a sloping approach channel, the rotation distance remained within z/D = 2 for a flow rate of 20 L/s.
Figure 13. The Pmin/Pmax ratio in cross-sections with drop distance z/D in dropshaft with horizontal and slopping approach channel (e/D = 0.25). (a) Horizontal approach channel; (b) Sloping approach channel (1:10).

Figure (a) and (b) illustrate the minimum Vr/Vz values ((Vr/Vz)min) across the cross sections of the two intake structures. In both structures, the (Vr/Vz)min values decreased rapidly from the dropshaft inlet. Similar conclusions were drawn when using (Vr/Vz)min > 0.2 as the criterion for stable vortex flow. Under a flow rate of 40 L/s, vortex flow formed and was sustained in dropshafts with horizontal approach channels for z/D > 7, while it failed to develop for smaller flows. Conversely, in dropshafts with sloping approach channels, vortex flow was maintained at flow rates of 20 and 40 L/s.
Figure 14. The (Vr/Vz)min varies with drop distance z/D in dropshaft with (a) horizontal and (b) slopping approach channel (e/D = 0.25). (a) Horizontal approach channel; (b) Sloping approach channel (1:10).

Figure depicts the Pmin/Pmax ratios across the cross section at different drop distances z/D for cases with a flow rate of 20 L/s and varying e/D ratios. In all cases with a horizontal approach channel, the Pmin/Pmax ratios remained below 0.2, indicating the absence of rotational flow. However, in dropshafts with a sloping approach channel and e/D ratios of 0.25 and 0.4, vortex flow was observed within z/D = 3, followed by a decrease in the Pmin/Pmax ratio below 0.1. The Pmin/Pmax ratios for the case with an e/D ratio of 0.5 ranged from 0.1 to 0.2, representing a transition flow. Similarly, when using a (Vr/Vz)min > 0.2 as the criterion for stable vortex flow (Figure ), the same conclusions was drawn. The only discrepancy was that the case with an e/D ratio of 0.5 in the sloping channel dropshaft was classified as vortex flow based on the velocity criteria.
4. Discussions
4.1. Interception performance
The interception performance of intake structures was analyzed by studying the water surface elevation along the approach channel and dropshaft. In horizontal approach channels, the water surface elevation increased as the contraction ratio decreased, particularly when the ratio fell below 0.4. Blockages in the dropshaft and tapering sections caused a rapid rise in the water surface of the approach channel, resulting in a lower interception rate for the intake structure. In dropshafts with a sloping approach channel, no significant increases in water level were observed along the approach channel, although water jumps were clearly visible in dropshafts and tapering sections. The influence of the inflow rate demonstrated varying changes in water depth and hydraulic jump movements at different flow rates. The water depth in the approach channel rapidly increased with increasing flow rate, while the water surface elevation remained relatively stable in cases with sloping channels.
4.2. Rotation criteria
The classification of dropshaft operations into rotational and nonrotational operations was performed based on the criteria of wall pressure and velocity ratio. For the same inflow conditions and contraction ratio, dropshafts with a horizontal approach channel exhibited more pronounced plunging flow characteristics than those with a sloping approach channel. Specifically, in dropshafts with horizontal channels, the pressure peak was larger but not sustained over a long distance (refer to Figures and ). The highest values of Vr/Vz occurred earlier in the horizontal cases, followed by a rapid drop (refer to Figures and ), indicating weaker rotational strength. There are other criteria we can use to evaluate the performance of dropshafts, such as the vorticity distribution and reduction (Norizan et al., Citation2018), which we can discussed further in our future works.
When considering the effect of the contraction ratio, stronger rotation occurred in cases with smaller contraction ratios (e/D) in dropshafts with both horizontal and sloping approach channels. A larger e/D ratio led to a sharp peak in the pressure and velocity ratio followed by a rapid decrease, causing the flow to transition into plunging flow. Hence, selecting an appropriate contraction ratio and a gentle slope can ensure the formation of successful rotational flow. The rotational distance is longer in cases with a sloping channel and smaller e/D ratios. However, caution should be exercised to avoid water surges and explosions in cases with small e/D ratios.
5. Conclusions
For the same flow rate, reducing the e/D ratio led to an increase in water level and a decrease in drainage efficiency under subcritical inflow conditions (with horizontal approach channel cases), whereas this effect was not observed in cases with supercritical inflow conditions (with sloping approach channels). However, in dropshafts with supercritical inflow conditions, the water level rose significantly in the tapering section and dropshaft wall, resulting in excessive mixing of air and water. This phenomenon could potentially lead to explosive eruptions of air–water mixtures, particularly in cases with higher flow rates. While a dropshaft with a horizontal approach channel exhibited a higher maximum wall pressure, this pressure quickly dropped after reaching its peak and could not be sustained over a long distance. The rotational distance decreased as the contraction ratio increased. Plunging flow, characterized by sudden changes in wall pressure that could cause erosion, were observed in cases with larger e/D ratio ( 0.7) and medium flow rate (∼20 L/s). Similar to the pressure distribution results, the rotational effect was more pronounced in cases with higher flow rates and critical inflow conditions. By using criteria such as a pressure ratio Pmin/Pmax > 0.2 and a velocity ratio (Vr/Vz)min > 0.2 across the cross sections of the dropshaft, similar classifications of rotational and nonrotational flow patterns were made.
Disclosure statement
No potential conflict of interest was reported by the author(s).
Data availability statement
All data that support the findings of this study are available from the corresponding author upon reasonable request. All models used during the study appear in the published article.
Additional information
Funding
References
- Chan, S. N., & Qiao, Q. S. (2022). Flow characteristics of a tangential vortex intake with steep-slope tapering section. Water Supply, 22(6), 5818–5832. https://doi.org/10.2166/ws.2022.215
- Chang, L., & Wei, W. (2022a). Numerical investigation of the plunging and vortex-flow regimes occurring in drop shafts with a tangential intake. Journal of Irrigation and Drainage Engineering, 148(8), 04022026. https://doi.org/10.1061/(ASCE)IR.1943-4774.0001692
- Chang, L., & Wei, W. (2022b). Numerical study on the effect of tangential intake on vortex dropshaft assessment using pressure distributions. Engineering Applications of Computational Fluid Mechanics, 16(1), 1100–1110. https://doi.org/10.1080/19942060.2022.2072954
- Chau, K. W., & Jiang, Y. W. (2004). A three-dimensional pollutant transport model in orthogonal curvilinear and sigma coordinate system for Pearl River estuary. International Journal of Environment and Pollution, 21(2), 188–198. https://doi.org/10.1504/IJEP.2004.004185
- Jianyun, Z. H. A. N. G., Yintang, W. A. N. G., Ruimin, H. E., Qingfang, H., & Xiaomeng, S. (2016). Discussion on the urban flood and waterlogging and causes analysis in China. Advances in Water Science, 27(4), 485–491.
- Kan, K., Xu, Y., Li, Z., Xu, H., Chen, H., Zi, D., Gao, Q., & Shen, L. (2023). Numerical study of instability mechanism in the air-core vortex formation process. Engineering Applications of Computational Fluid Mechanics, 17(1), 2156926. https://doi.org/10.1080/19942060.2022.2156926
- Ma, Y. Y. (2016). Air entrainment and energy dissipation in drop structures in drainage system [PhD thesis]. Zhejiang University. (in Chinese).
- Norizan, T. A., Reda, E., & Harun, Z. (2018). Enhancement of vorticity reduction by floor splitter in pump sump to improve pump efficiency. Sustainable Energy Technologies and Assessments, 26, 28–36. https://doi.org/10.1016/j.seta.2017.06.001
- Pfister, M., Crispino, G., Fuchsmann, T., Ribi, J. M., & Gisonni, C. (2018). Multiple inflow branches at supercritical-type vortex drop shaft. Journal of Hydraulic Engineering, 144(11), 05018008. https://doi.org/10.1061/(ASCE)HY.1943-7900.0001530
- Plant, J., & Crawford, D. (2016, September). Pushing the limits of tangential vortex intakes: Is higher capacity and flow measurement possible in a smaller footprint? In WEFTEC 2016. Water Environment Federation.
- Rajaratnam, N., Mainali, A., & Hsung, C. Y. (1997). Observations on flow in vertical dropshafts in urban drainage systems. Journal of Environmental Engineering, 123(5), 486–491. https://doi.org/10.1061/(ASCE)0733-9372(1997)123:5(486)
- Shen, W., Wang, S., Zhang, X., & Liang, X. (2022). Large-eddy simulation and mathematical model of vortex breakdown and pressure drop in a cavity with tubeless vortex reducer. Engineering Applications of Computational Fluid Mechanics, 16(1), 1344–1363. https://doi.org/10.1080/19942060.2022.2091662
- Sun, J., Qian, S., Xu, H., Chen, Y., & Ren, W. (2023). Standing waves of the stepped dropshaft in a deep tunnel stormwater system. Water Science and Technology, 87(2), 407–422.
- Wang, B., Lv, W., Chen, R., Zhang, J., & Li, C. (2023). Research on the mechanism and influencing factors of geysers in deep tunnel drainage systems. Frontiers in Civil and Hydraulic Engineering, 1, 183–195.
- Wei, W., & Chang, L. (2023). Analytical solutions for vortex flow at the tangential inlet of a vertical dropshaft. Physics of Fluids, 35(1), 015160. https://doi.org/10.1063/5.0135575
- Yang, Z., Zhu, C., & Zheng, N. (2018). Droplet impact simulation of hydrophobic patterned surfaces by computed fluid dynamics. Academic Journal of Engineering and Technology Science, 1(1), 49–55.
- Yu, D., & Lee, J. H. (2009). Hydraulics of tangential vortex intake for urban drainage. Journal of Hydraulic Engineering, 135(3), 164–174. https://doi.org/10.1061/(ASCE)0733-9429(2009)135:3(164)
- Zhang, C., Tang, H., Del Giudice, G., Gisonni, C., & Rasulo, G. (2009). Vortex drop shaft for supercritical flow. In Advances in water resources and hydraulic engineering: Proceedings of 16th IAHR-APD Congress and 3rd Symposium of IAHR-ISHS (pp. 1515–1520). Springer.
- Zhang, Y., Chen, Y., Qian, S., Xu, H., Feng, J., & Wang, X. (2022). Experimental study on geysers in covered manholes during release of Air pockets in stormwater systems. Journal of Hydraulic Engineering, 148(5), 06022003. https://doi.org/10.1061/(ASCE)HY.1943-7900.0001978