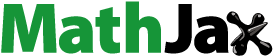
Abstract
Advanced cooling techniques are essential in modern gas turbines to improve the efficiency of the Brayton cycle by raising the turbine inlet temperature. Mist-assisted film cooling offers an innovative solution for cooling high-temperature gas turbine blades. When mist particles with various momenta are injected through the cooling holes, the interplay between mainstream turbulence intensity (Tu) and mist momentum plays a crucial role in mist-assisted film cooling under high Tu conditions. Hence, this numerical study investigates the relationship between mainstream Tu and mist characteristics for the first time, considering the blowing ratio and mist diameter as controlling parameters. Film effectiveness and particle distribution statistics are analysed to understand their correlation. The results demonstrate that mist-assisted film effectiveness follows the trend of air-only film cooling with varying blowing ratios and Tu; however, the magnitude is amplified. The influence of the mist diameter on film effectiveness is determined by the surface-area-to-volume ratio and Stokes number. These factors impact the sensitivity of particles to Tu and subsequently affect film effectiveness. This study provides a novel quantitative analysis of the combined effect of mainstream Tu and mist on film cooling effectiveness, revealing the correlation between mist and mainstream parameters.
Highlights
Combined effect of mainstream turbulence intensity (Tu) and mist characteristics is investigated.
High mainstream Tu significantly increases the cooling enhancement induced by mist.
Mist-assisted film cooling exhibits similar trends to varying mainstream Tu and blowing ratio.
Mist diameter significantly influences the response to Tu, governed by surface-area-to-volume ratio and Stokes number.
Small particles demonstrate the highest cooling effectiveness and variation by Tu.
Nomenclature
D | = | hole diameter (mm) |
dp | = | mist diameter (μm) |
DR | = | density ratio |
F | = | force (N) |
I | = | momentum flux ratio |
L | = | hole length (mm) |
= | mass flow rate (kg/s) | |
M | = | blowing ratio |
Nu | = | Nusselt number |
P | = | pressure (Pa) |
RH | = | relative humidity |
SA:V | = | surface-area-to-volume ratio |
Sh | = | Sherwood number |
Stk | = | Stokes number |
T | = | temperature (K) |
Tu | = | mainstream turbulence intensity |
u, U | = | flow velocity (m/s) |
y+ | = | dimensionless wall distance |
Greek symbols
η | = | film cooling effectiveness |
θ | = | dimensionless temperature |
ρ | = | density (kg/m3) |
Subscripts
aw | = | adiabatic wall |
c | = | coolant |
p | = | particle |
wb | = | wet bulb |
∞ | = | mainstream |
Introduction
The modern gas turbine industry requires an increasing turbine inlet temperature (TIT) to achieve a higher Brayton cycle efficiency. Hence, schemes for vane/rotor cooling have become increasingly important. Film cooling is a cooling method in which a cooling air film is injected on the vane/rotor surface to protect the material from damage by hot gas. In addition, film coverage and length, which are affected by various factors (e.g. hole geometry, mass and momentum fluxes, velocity, and density ratio), are crucial and have been studied by numerous researchers.
Sinha et al. (Citation1991) experimentally investigated the effect of the mainstream-to-coolant density ratio on film effectiveness with a cylindrical hole inclined at 35°. Under fixed blowing, velocity, and momentum ratios, the results indicated that film effectiveness increased with the density ratio. They concluded that a coolant with a higher density ratio had a lower momentum ratio at a constant mass flux, thereby reducing the lift-off reattachment phenomenon and enhancing lateral spreading. Schmidt et al. (Citation1996) investigated the effects of a compound angle in the cooling hole. Using simple cylindrical and forward-expansion holes, the spanwise-averaged effectiveness was increased by the addition of a compound angle. The authors concluded that the compound angle led to higher film effectiveness for a wider range of momentum flux ratios. Liu et al. (Citation2023) proposed a method to distinguish the effects of internal, bore, and film cooling by numerically decoupling the effects. They observed a shift in the dominant cooling factor from film cooling to bore/internal cooling with an increase in the blowing ratio. In a study of supersonic film cooling, Wei et al. (Citation2023) investigated the effects of combustion on near-wall turbulence in supersonic hydrogen film cooling using large eddy simulation (LES). Their findings revealed that the boundary layer combustion suppressed the turbulent heat and mass transfer compared to momentum transfer.
Research associated with actual gas turbine blade cooling has also been conducted. Liu et al. (Citation2021) analysed the flow characteristics of a pre-swirl system in a cooling air delivery channel and discovered a correlation between the mass flow rate and entropy increment. Wang et al. (Citation2022) conducted a comprehensive study on the optimisation of guide vane film cooling with full-coverage analysis. By considering factors such as hole shape and film superposition, they achieved an optimised configuration that led to a shifted coolant mass flow distribution from the leading edge/pressure side to the suction side and an improved discharge coefficient.
In addition to the coolant configuration, mainstream conditions play an important role in film cooling. The effect of mainstream oscillations on film cooling was investigated computationally by Baek and Yavuzkurt (Citation2018). The authors used sinusoidal mainstream velocity in the Reynolds-averaged Navier – Stokes (RANS) simulation and LES to investigate the effects of combustion instability. Their observations revealed that the effects of the oscillation were opposite at low and high blowing ratios. They concluded that this contrasting trend was due to the jet lift-off and coolant flapping. Zhou et al. (Citation2018) experimentally investigated the effect of mainstream oscillations. According to their proper orthogonal decomposition analysis results, when the mainstream oscillation frequency increased, the contribution of the large-scale coherent structure decreased owing to dissipation. Abadi et al. (Citation2020) conducted experimental and numerical investigations on the effect of mainstream pulsation on the film cooling effectiveness of the NASA C3X blade. They found that the lowest pulsating frequency resulted in the lowest averaged film effectiveness and that mainstream pulsation caused variations in the size and strength of the counter-rotating vortex pairs (CRVPs).
In particular, the mainstream turbulence intensity (often referred to as Tu) is an important mainstream condition that affects film cooling effectiveness. The turbulence intensity at the turbine inlet can vary by up to 20%, which is affected by the combustor geometry (Bicen & Jones, Citation1986). Many studies have been conducted to reveal the effect of a high mainstream Tu on film cooling effectiveness. Bons et al. (Citation1996) experimentally analysed the mainstream Tu effect on the film cooling performance at a Tu level of 0.9–17% with cylindrical holes. They concluded that although the mainstream Tu has a significant effect on film effectiveness, this tendency is not consistent throughout the range of blowing ratio (i.e. the trend reverses at some point). In addition, Kohli and Bogard (Citation1998) investigated the jet-mainstream interaction by varying mainstream Tu by up to 20%. Moreover, probability density functions of the dimensionless temperature results revealed the mechanism of mainstream-jet mixing at different Tu levels. Based on their observations, the dominant factor for mixing at a low Tu level was the shear layer between the mainstream and coolant jets, whereas those at high Tu levels were large turbulence structures and mainstream thrust through the core. These results demonstrated the effect of the mainstream Tu on coolant mixing. Two main effects of mainstream Tu were revealed, namely lateral dispersion and dissipation of the coolant (Bons et al., Citation1996; Kohli & Bogard, Citation1998); moreover, Saumweber et al. (Citation2003) reaffirmed these effects by comparing different types of holes. The cylindrical hole results showed that a higher mainstream Tu was beneficial at a higher blowing ratio because the coolant jet was reattached by Tu, although the dissipation was also higher. However, in the case of shaped holes, the jet was not detached and the lateral coverage was already good. Thus, a higher Tu only exhibited a detrimental effect owing to dissipation. The effects of lateral dispersion and dissipation were clearly distinguishable in their study. Schroeder and Thole (Citation2016) investigated the effects of mainstream Tu at different blowing ratios. They reported that the mainstream Tu was not always detrimental to shaped holes when the blowing ratio was sufficiently high (M = 3.0). They also found that the main source of the coolant turbulent intensity changes from the internal disturbance of the hole to the coolant jet wake region when lift-off occurs. Film cooling on a convex 3-D blade geometry showed the same reaction under Tu conditions, as reported in a computational study by Baheri Islami and Jubran (Citation2012). Although the utilised geometry had trenched holes, mainstream Tu was advantageous when jet lift-off occurred. They reported that the sensitivity of effectiveness to the mainstream differed depending on the hole geometry.
As the TIT further increased in recent years, many novel film cooling hole designs were suggested; however, air film cooling slowly reached its potential limit. Subsequently, mist film cooling, a novel cooling scheme to improve cooling performance, was introduced by Li and Wang (Citation2006). By computationally simulating flat-plate film cooling with mist injection, the results indicated that the film effectiveness was enhanced by up to 50% by adding a small fraction of mist (∼ 2% of coolant mass flow rate). Subsequently, the same authors (Li & Wang, Citation2007) investigated the effects of mist particle modelling schemes on simulation results, including sub-models, such as the Saffman lift, as well as thermophoretic and Brownian forces. As the mist evaporation rate changes under elevated conditions, Wang and Li (Citation2008) simulated mist film cooling at much higher temperatures, pressures, and velocities. Rao et al. (Citation2018) performed 2-D numerical simulations to examine the effects of mist diameter, mist mass fraction, and the relative humidity of the coolant. They reported an increase in film effectiveness with a larger mist mass fraction and higher relative humidity, and a decrease with a larger mist diameter. Dwivedi and Sarkar (Citation2023) also conducted numerical simulations of mist-assisted film cooling to examine the effects of mist concentration and diameter. Their study demonstrated a relationship between the mist particle distribution and flow structures, such as the CRVP. Abdelmaksoud and Wang (Citation2020) conducted a conjugate heat transfer simulation of mist film cooling in a 3-D gas turbine blade. They injected mist at the entrance of the internal cooling passage of the blade and compared the cooling performance under different mist/air ratios and mist diameters. Their findings showed that increasing the mist diameter beyond a certain limit resulted in a decrease in cooling effectiveness induced by mist. Zhao and Wang (Citation2014a) conducted experiments on mist-assisted film cooling and compared the film effectiveness of air-only and mist-assisted film cooling. They reported that the overall pattern of film cooling was similar in both cases, indicating that the knowledge gained from air film cooling remains applicable to mist-assisted film cooling. In their subsequent analysis on the particle dynamics of this system (Zhao & Wang, Citation2014b), some intriguing behaviours of droplets with varying sizes were reported; the droplet size population density exhibited a skew in the upward direction. This tendency was attributed to the inertia of mist particles. Pabbisetty and Prasad (Citation2021) conducted similar experiments to investigate the effect of the blowing ratio in mist-assisted film cooling. Consistent with air film cooling, the choice of blowing ratio is essential to maximise the advantages of mist injection.
As mentioned earlier, mist particle motion after injection is in 3-D space and involves both vertical and lateral movements that can affect the coverage and overall efficiency of the film. Moreover, the mainstream Tu reportedly changes the vertical and lateral motions of the injectant. Dwivedi and Sarkar (Citation2022) investigated the effect of mainstream Tu on mist-assisted film cooling using a constant mist diameter of 10 μm. They found that a higher Tu enhances the spanwise distribution of the mist particles, resulting in an improved film effectiveness. However, since realistic mist injection involves a wide range of diameters and velocities (Pabbisetty & Prasad, Citation2021; Zhao & Wang, Citation2014b), particles with significantly different momenta react differently to the mainstream Tu. Therefore, a comprehensive understanding of how various mist particles respond to mainstream Tu is crucial, as it strongly affects the lifespan and coverage of the film layer, as reported in the aforementioned studies. However, to the best of the authors’ knowledge, no studies have addressed the combined effect of the mist and air film cooling parameters.
In this study, numerical simulations are conducted to investigate the interactions between mainstream Tu and parameters influencing mist momentum. The simulations utilise a simple flat-plate film cooling geometry with inclined cylindrical holes. The blowing ratio (M) and mist diameter (dp) are chosen as the mist parameters to vary and explore the characteristics of the mist. Each parameter is tested under low (0.1%) and very high (20%) Tu conditions. To quantitatively assess the response of the particles to Tu, statistical data is provided with its corresponding impact on the film cooling effectiveness. The findings of this study offer valuable insights applicable to the utilisation of mist in diverse turbine operating conditions.
Materials and methods
Geometry and meshing
The simple cylindrical hole and flat plate geometry used in this study were based on the experimental setup from Pietrzyk et al. (Citation1990), as shown in Figure . The hole had a diameter of 12.7 mm with an inclination angle of 35° and a plenum underneath. The length-to-diameter ratio (L/D) of the hole was 3.5. The computational domain for the mainstream had a length, width, and height of 41.7D, 3D, and 6D, respectively.
Figure 1. Computational domain, dimensions, and boundary conditions. The main simulation domain is depicted by the solid black line. The 14D region preceding the inlet was simulated separately and used as the inlet condition for the main simulation. The shaded regions in blue, red, and yellow represent the inlet, outlet, and symmetric boundary conditions, respectively.

In the original experiment rig (Pietrzyk et al., Citation1990), the distance between the mainstream inlet and the leading edge of the hole was 19D. However, because flow simulations coupled with 3-D particle simulations are computationally intensive, the inlet distance was reduced to 5D in this study, and separate simulations were conducted for the inlet boundary layer growth and used as conditions for the profiled inlet in the main simulation cases. This approach for the inlet boundary layer was also used in previous studies (Ahn, Citation2022; Leedom & Acharya, Citation2008). The coordinate origin was set as the centre of the trailing edge of the hole. The geometric details of the computational domain are shown in Figure .
The computational grid presented in Figure was generated using ICEM CFD software (Ansys, Inc.; Canonsburg, PA, USA). A previous study by Yavuzkurt and Habte (Citation2008) highlighted the significance of employing an appropriate mesh type to achieve accurate predictions of film effectiveness. Specifically, it has been established that a single hexahedral mesh aligned with the flow pattern enhances the precision of film effectiveness predictions. Thus, this study employed a structured hexahedral mesh.
Figure 2. Generated hexahedral grid with magnified view: (a) YZ-plane view, (b) XY-plane view, (c) near-hole region, (d) hole cutaway.

To accurately capture the complex flow phenomena of an inclined jet in a crossflow, a high-density mesh was used for the hole and near-hole regions (X/D < 5). The computational domain contained 180, 40, and 60 cells in the X, Y, and Z directions, respectively. The near-wall grid was designed to maintain a dimensionless wall distance y+ between one and two along the downstream region of the coolant hole. As flow becomes less complex downstream and has a reduced impact on film effectiveness, the grid density gradually decreased in the downstream direction.
To select the optimal number of elements while considering grid convergence, the results of centreline film cooling effectiveness were compared for four grid densities. The number of elements used for the grid sensitivity test varied from 0.20–1.37 million. In Figure , the results of the grid sensitivity test are presented based on the centreline film effectiveness. By varying the number of nodes in the X, Y, and Z directions, the centreline film effectiveness results gradually converged as the grid density increased. The maximum percentage gap between the 2nd – 3rd and 3rd – 4th grids was below 0.4%. Therefore, the 3rd grid, which consisted of 0.75 million elements, was selected.
Figure 3. Centreline film effectiveness results calculated from four grid densities with M = 0.78, DR = 1.3, and dry conditions.

Additionally, to ensure the grid independence of evaporating species, the H2O mass fraction data along the streamline at different grid densities are compared in Figure . Similarly to the centreline film effectiveness, the difference in H2O mass fraction values between the 2nd and 3rd grids was insignificant, whereas there were significant differences between the 1st and 2nd grids. This can be attributed to the inadequate prediction of fluid flow and coarser integration of particle trajectories. Therefore, it was concluded that the selected grid in this study demonstrates sufficient grid independence.
The air-only (dry) results obtained from the selected grid were validated against those of previous studies (Harrison & Bogard, Citation2008; Sinha et al., Citation1991) (see Figure (a)). To ensure accurate calculation of the continuous flow field, both the centreline and spanwise-averaged film effectiveness were compared. Under well-matched conditions with density ratio (DR) = 1.2 and M = 0.78, the computational results of this study align closely with the experimental result from Sinha et al. (Citation1991).
Figure 5. (a). Comparison of simulation results with experimental (Exp) and simulation results from previous studies: 1. Exp: Sinha et al. (Citation1991), DR = 1.2, M = 0.78; 2. Computational fluid dynamics (CFD): Harrison and Bogard (Citation2008), DR = 1.5, M = 1.0. (b). Comparison of centreline film effectiveness results with experimental studies (Sinha et al., Citation1991; Zhao & Wang, Citation2014a) for mist-injected cases. The comparisons were conducted under M = 0.6 conditions with coolant-mist mass fractions set to .

The maximum and minimum errors between the experimental and numerical results were 89.3% and 28.6%, respectively. This discrepancy between the experimental and numerical data can be attributed to several factors. First, the experimental rig in the Sinha et al. (Citation1991) study had a shorter hole length (L/D = 1.75) than that used in the current study, which led to stronger lift-off at the near-hole region. Second, RANS simulations have inherent limitations when predicting film cooling effectiveness. Previous studies (Bianchini et al., Citation2013; Baek & Yavuzkurt, Citation2018; Ahn, Citation2022) reported the limitations of RANS when predicting anisotropic turbulence and coolant-mainstream mixing.
However, due to the cost-effectiveness and robustness of RANS methods, they are widely used in film cooling research and provide reliable results. Furthermore, the numerical results of this study demonstrate a closer alignment to experimental data than to the previous numerical results by Harrison and Bogard (Citation2008). Therefore, the numerical method was judged to be valid.
Additionally, the mist-injected results were compared with an experimental study conducted by Zhao and Wang (Citation2014a) to validate the numerical method for mist modelling. In Figure (b), centreline film effectiveness results are compared under M = 0.6 conditions. The dry results exhibited good agreement with experimental data, as did the M = 0.78 cases. Furthermore, the mist-injected results also closely aligned with the experimental data from Zhao and Wang (Citation2014a). Based on the results shown in Figures (a) and (b), we conclude that the numerical method employed in this study is valid.
Boundary conditions
The boundary conditions used in this study are listed in Table . As mentioned in the previous section, the inlet distance was shortened because of the computational intensity of the particle simulation. Hence, the velocity profile was extracted from a separate simulation with the same grid density and wall y+ value, and then assigned as the velocity profile condition at the mainstream inlet. The assigned velocity profiles are presented in Figure . The velocity input for the separate simulation was uniform at a mainstream flow velocity of U∞ = 20 m/s. As the main target of this study is to investigate the effect of mainstream turbulence, turbulence intensities of 0.1% and 20% for low and high conditions were applied to the mainstream inlet, respectively. The plenum inlet was set as the mass flow inlet condition to easily calculate and match the blowing ratio. To include the evaporation and heat absorption effect of the mist, the DR was set as 1.3 (mainstream temperature T∞ = 400 K, coolant temperature Tc = 300 K). The outlet conditions were set as the pressure outlet with an atmospheric pressure of P = 101,325 Pa. Periodic conditions were set on the sides of the domain (the main flow and plenum). The walls (the top of the plenum, hole, and bottom wall) were set as adiabatic no-slip conditions.
Table 1. Boundary conditions used in the numerical simulations.
In addition, the mist injection configuration is discussed. The injection point was located 1D below the centre of the plenum exit (hole). Mist particles were injected into a cone shape with a cone angle of 10° at a velocity of 5 m/s, which is slow compared with that of the airflow toward the plenum exit. The mist temperature was Tp = 300 K, and the mass flow rate was set as 10% of the coolant mass flow rate. While mist injection in actual applications typically exhibits a nonuniform diameter distribution, for the purpose of isolating the effect, the simulated mist particles in this study were injected with a uniform diameter. The corresponding range was 5–20 μm.
Numerical model
Numerical simulations were conducted using the commercial computational fluid dynamics software, FLUENT 2021 (Ansys, Inc.). FLUENT provides multiphase modelling using the Eulerian – Lagrangian point-particle method (also called DPM). In this study, injected mist particles were modelled using the DPM. To ensure sufficiently converged solutions, iterations were performed until the residuals decreased to 10−5 for mass and 10−6 for momentum, energy, k, ϵ, and species. Additionally, scalars such as wall temperature, mist-penetration length, and H2O mass fraction at the outlet were monitored to verify the steady-state condition of the solution. The governing equations for each phase are presented next.
Continuous phase
The continuous phase was solved using RANS equations. As the discrete particle simulation interacts with the continuous phase, source terms are added to the mass, momentum, and energy equations Equations (1–3).
(1)
(1)
(2)
(2)
(3)
(3) Sm, Fj, and Sh are the source terms from the liquid – phase interaction, which represent the mass, momentum (mainly drag), and latent heat source, respectively. In addition, the following species equation was solved to consider the evaporation of water droplets.
(4)
(4) where Cj, Deff.j, and Sj represent the mass fraction, effective diffusion coefficient, and source term, respectively, of species j.
Turbulence was modelled using the k-ϵ model, which has shown good agreement with film cooling experimental data and been widely used in previous studies (Harrison & Bogard, Citation2007; Hassan & Yavuzkurt, Citation2006; Johnson et al., Citation2011). In particular, the realisable k-ϵ model is reportedly better at predicting the dispersion of the coolant jet than other models (Hassan & Yavuzkurt, Citation2006). Therefore, the realisable k-ϵ model with enhanced wall treatment was used in this study.
Discrete phase
In this study, the volume fraction of the liquid phase was negligible (0.01%). Hence, the Eulerian – Lagrangian approach was suitable for multiphase modelling. The Eulerian – Lagrangian method solves the continuous phase using the Eulerian approach and calculates the particle trajectory using the Lagrangian approach. The flow variables stored in each computational cell are used for calculating the particle trajectory (ANSYS, Citation2021). In addition, a two-way coupled calculation allows the continuous and dispersed phases to affect each other (i.e. heat absorption and mass exchange by evaporation). Equations (5) and (6) represent the particle motion balance and particle relaxation time formulations, respectively.
(5)
(5)
(6)
(6) The drag force, which is caused by the difference in velocity between the particle and the surrounding gas, is the primary driving force for the particle. Gravitational and buoyancy forces are also considered. In Equation (5),
represents an additional force term that accounts for forces such as thermophoresis, Brownian motion, Saffman lift, and Magnus lift. In this study, only the thermophoretic force was included, in reference to a previous study (Li & Wang, Citation2007) on the modelling schemes of mist film cooling simulations.
Energy balance was also considered in the heat transfer calculation. Equations (7) and (8) represent the particle energy balance and Nusselt number correlation, respectively.
(7)
(7)
(8)
(8)
(9a)
(9a)
(9b)
(9b)
Equation (7) represents the energy balance of a particle, considering convection and evaporation effects, where cp denotes the specific heat of the droplet and hfg denotes the latent heat of vaporisation. FLUENT calculates the convective heat transfer coefficient h in Equation (7) from the Nusselt number relationship in Equation (8), as reported by Sazhin (Citation2006). In Equation (8), BT is the Spalding heat transfer number, which is calculated using the Spalding mass number (Bm; see Equation (9)). Note that cpv and cpg are the mean specific heats of water vapour and gas, respectively, and Yi is the vapour mass fraction. The radiation of particles was neglected in this study because the temperature was not extremely high.
Equation (10) expresses the mass transfer between the bulk flow and water droplet. Furthermore, kc in Equation (10) is the mass transfer coefficient of the droplet obtained from the Sherwood number correlation in Equation (11) (Ranz, Citation1952).
(10)
(10)
(11)
(11) More detailed information regarding the drop heat and mass transfer laws is available in (ANSYS, Citation2021).
Mist film effectiveness
Due to the evaporation of the injected mist, the temperature drops below the initial coolant temperature (300 K). Therefore, the near-hole region film effectiveness is greater than unity. To correct this, previous studies (Pabbisetty & Prasad, Citation2021; Rao et al., Citation2018) introduced a mist film effectiveness that regards the relative humidity of the coolant. In contrast to the conventional film effectiveness in Equation (12), the mist film effectiveness uses the wet-bulb temperature Twb.
(12)
(12)
(13)
(13) where the subscripts ∞, aw, c, and wb indicate mainstream, adiabatic wall, coolant, and wet bulb, respectively. To include the evaporation effect, the temperature (T) and relative humidity (RH) of the coolant were used to calculate its wet-bulb temperature using the empirical formula in Equation (14), as reported by Stull (Citation2011).
(14)
(14) Equation (14) was derived using linear regression from the psychrometric diagram for standard sea-level pressure; thus, it is only valid for air at approximately 101.325 kPa. The air pressure in this study was close to this value, validating the use of Equation (14). Note that Twb of the dry coolant at 300 K was 282 K. To conclude, the film effectiveness obtained in this study uses the aforementioned definition of mist film effectiveness.
Results and discussion
Table lists the parameter space used in this study. The key parameters are the mist diameter, mainstream Tu, and blowing ratio (M). The blowing ratio can be calculated using the following expression:
(15)
(15)
(16)
(16) The other parameters are identical for all cases (see Boundary Conditions). In the case of cylindrical hole film cooling, the momentum flux ratio (I) effectively scales the film cooling performance under various conditions (Burd & Simon, Citation1997). The momentum flux ratio is defined by Equation (16), and its values for the M = 0.25 and 0.78 cases are 0.048 and 0.468, respectively. This value can be used to relate and match film cooling conditions to any scenario.
Table 2. Parameter space of this study. Note that the case ID includes the important parameters: diameter, mainstream Tu, and blowing ratio.
Overall, mist-assisted film cooling results in significantly higher film effectiveness than dry film cooling. This improvement can be attributed to three mechanisms: (1) latent heat of vaporisation acting as a ‘heat sink;’ (2) direct contact cooling at the wall; and (3) water vapour forming a high specific heat (cp) layer (Li & Wang, Citation2006; Wang & Li, Citation2008; Zhao & Wang, Citation2014a). Furthermore, these effects are influenced by mainstream turbulence and are described later in this section.
Comparison of 2-D and 3-D mist film cooling
Prior to the main analysis, the difference between 2-D and 3-D film cooling was investigated. The 2-D computational domain was identical to the projection of the 3-D domain. The dimensionless temperature distributions for the 2-D and 3-D cases are shown in Figure . As previously mentioned, 2-D slot film cooling results in a higher film effectiveness than 3-D discrete film holes. This is due to the absence of a 3-D secondary flow, which results in mixing between the coolant and mainstream (Wang & Li, Citation2008). Comparing Figures (a) and (c), the 2-D film cooling shows neither jet lift-off nor entrainment of the mainstream. Because the dimensionless temperature (θ) is already sufficiently high in the 2-D dry case, the mist injection in the 2-D case does not have a significant difference. Furthermore, the mist particle distributions are different between the 2-D and 3-D cases. As shown in Figure , the particles in the 2-D case are lifted after the injection and then remain floating on the surface. However, the particles in the 3-D case quickly return to the surface after injection, which was described as ‘bending back’ by Zhao and Wang (Citation2014b). This is because the CRVP of the coolant drags the mist down to the wall (Dwivedi & Sarkar, Citation2023), which is not present in the 2-D cases. Consequently, the test plate (bottom wall) is not sufficiently cooled by the mist in the 2-D cases. These results are also reflected in the centreline film effectiveness plots shown in Figure . The 2-D cases (dashed lines) show no difference, whereas the 3-D cases show a significant difference owing to mist injection. Therefore, the 3-D analysis of mist film cooling is more appropriate for analysing the effects of 3-D flow characteristics that might affect the mist distribution and its cooling enhancement. Furthermore, the mainstream Tu greatly influences the lateral dispersion of the injectant. Therefore, a 3-D analysis of mist-assisted film cooling may lead to a more realistic understanding of mist film cooling with a high mainstream Tu.
Figure 7. Comparison of mid-section (Z/D = 0) dimensionless temperature (θ): (a) 2-D, air only; (b) 2-D, dp = 10 μm; (c) 3-D, air only; (d) 3-D, dp = 10 μm.

Effect of blowing ratio (M)
In this section, the combined effect of the mainstream turbulence intensity and blowing ratio on mist-assisted film cooling is discussed, focusing on the coolant and mist particle behaviour. To isolate this effect, the other parameters, such as mist diameter and mist concentration, were fixed. Specifically, the mist concentration was fixed at 10% of the coolant mass flow rate and the mist diameter was fixed at 5 μm. Table provides an overview of the parameter space and case IDs used to investigate the turbulence effect.
Table 3. Parameter space and case IDs used to investigate the combined effect of mainstream turbulence intensity (Tu) and blowing ratio (M).
Figure provides a comprehensive outlook of mist-assisted film cooling at various blowing ratios, depicted through particle distribution and dimensionless temperature (θ) contours at X/D cut planes. The blowing ratio variation results in significant changes in coolant injection momentum. Moreover, the momentum of mist particles is strongly affected by the coolant flow, leading to distinct variation in particle distribution, as shown in Figure . At low blowing ratios (M = 0.25), both the coolant and particles exhibit lower distribution and attached to the wall; conversely, at higher blowing ratios (M = 0.78), they are lifted and show elevated distributions. This change in distribution with respect to the blowing ratio affects the response of film effectiveness to mainstream Tu. Note that the particle distribution corresponds to the high dimensionless temperature region in the contours. Further discussion on the response to mainstream Tu will be presented next.
Figure 10. Comparison of mist particle distributions for various blowing ratios (M) and corresponding dimensionless temperatures () at cut planes for cases D05T00M25 and D05T00M78 (dp = 5 μm, Tu = 0.1%).

In general, the effect of mainstream Tu on air film cooling is dependent on the blowing ratio. Previous studies (Baheri Islami & Jubran, Citation2012; Bons et al., Citation1996; Kohli & Bogard, Citation1998; Saumweber et al., Citation2003) reported that Tu has two primary roles that affect the coolant jet:
Thrusting down and laterally dispersing the coolant jet,
Dissipating and mixing the coolant into the mainstream.
Conversely, when the blowing ratio is high, the lifted coolant benefits from role (1), while the sufficient mass flux makes role (2) relatively insignificant. Consequently, film effectiveness is higher at higher Tu conditions. These two regimes of Tu response in air cooling are also observed in mist-assisted cases.
Figure shows the film effectiveness contours at the bottom wall for the four mist injection cases. The changing pattern of contour bands with mainstream Tu differs noticeably between the two blowing ratios. For low M values (D05T00M25 and D05T20M25 in Figure ), a decrease in the streamwise film length is evident in the contour. However, at high M values (D05T00M78 and D05T20M78 in Figure ), the streamwise film length increases as Tu increases, attributed to the thrust-down effect that enhances the contact between the coolant and the wall. In contrast to the difference in the change of film length, the spanwise width of the coolant jet increases in both the low and high blowing ratio cases. This indicates that the coolants at both low and high blowing ratios are laterally dispersed by Tu. In summary, these observations of film effectiveness regarding the Tu effect align with those of air-only film cooling, suggesting that the film effectiveness of the mist-injected coolant exhibits a similar response to Tu as the dry coolant.
Figure 11. Contours of film effectiveness (η) with two blowing ratios (M = 0.25 and 0.78) and two Tu levels (Tu = 0.1% and 20%).

The spanwise-averaged film effectiveness () is presented in Figures and as a comprehensive indicator of the lateral effect of the mainstream Tu on the coolant distribution. The trend of
is similar for the air-only and mist-assisted cases, but the presence of mist injection amplifies the gap between quiescent (T00) and turbulent (T20) cases, regardless of the blowing ratios.
Figure 12. Spanwise-averaged film effectiveness of M = 0.25 cases (dry and mist, Tu = 0.1% and 20%).

In the low M cases, the lateral dispersion effect of Tu increases of the dry case (DryT20M25) in the immediate downstream region after the injection. However, as mentioned earlier, the coolant jet decays rapidly, resulting in a decrease in
in the downstream direction. This is evident from the intersection of the solid and dashed lines in Figure . A similar pattern is observed in the mist cases, with a more pronounced gap between the quiescent (T00) and turbulent (T20) cases (red lines). Moreover, the intersection points of the T00 and T20 lines extend further downstream in mist-assisted cases than in dry cases, occurring at X/D = 18 and 10, respectively. In other words, the effect of Tu is amplified for mist-injected cases.
In the high M cases, higher values of by Tu are observed due to the thrust-down effect, which is consistent with the trend reported in the air-only cases mentioned earlier (see Figure ). Similarly, the gap caused by Tu is amplified in mist-assisted cases compared to dry cases, as observed in the low M cases. The intersection does not appear in the simulation domain of this study (X/D ≈ 35) because the coolant mass flux is sufficiently high to persist despite dissipation.
The mist dispersion effect of Tu was analysed by comparing the mist particle distributions for each Tu. As an indicator of the distribution, probability mass functions (PMFs) of the particles along the Y and Z directions are illustrated in Figures and . The vertical particle distribution in Figures (a) and (b) clearly demonstrates the thrust-down effect caused by Tu. The mist particles in the low blowing ratio cases show only a slight variation in their PMFs, and the results indicate that at a higher mainstream Tu, a larger concentration of mist particles is found close to the bottom wall. However, as shown in Figure , the lateral distributions of the two blowing ratios are inconsistent. In Figure (a) (M = 0.25 cases), the particle distribution in the DryT20M25 case is wider than that in the DryT00M25 case because of the lateral dispersion effect of Tu. However, In Figure (b) (M = 0.78 cases), the particle distribution in the DryT20M78 case is narrower than that in the DryT00M78 case. The reason for these opposing distribution trends for Tu is the different jet characteristics at different blowing ratios. In Figure , the jet cores of each case are visualised using dimensionless velocity contours in the X/D = 5 plane section. For the M = 0.25 cases (Figure (a)), the elevated Tu condition results in a slightly lower and laterally dispersed jet core. However, for the M = 0.78 cases (Figure (b)), the jet core in the quiescent condition exhibits a wider distribution than that in the elevated condition. The jet core under elevated conditions is thrust and contracted by Tu, exhibiting a narrower distribution. The responses of the jet structure and particle distribution coincide because the main factor driving the particles is their drag, which is directly related to the coolant flow structure. In other words, the distribution of mist particles follows the coolant response to the mainstream Tu when the blowing ratio varies.
Figure 13. Spanwise-averaged film effectiveness of M = 0.78 cases (dry and mist, Tu = 0.1% and 20%).

Figure 14. (a). Vertical particle distribution of M = 0.25 cases represented by probability mass function (PMF). (b). Vertical particle distribution of M = 0.78 cases represented by PMF.

Figure 15. (a). Lateral particle distribution of M = 0.25 cases represented by PMF. (b). Lateral particle distribution of M = 0.78 cases represented by PMF.

Figure 16. Comparison of dimensionless velocity (u/U∞) between Tu = 0.1 and 20% at different blowing ratios. Note that the jet structure is symmetrical; hence, it has been halved and combined for a clearer comparison.

In Figure , dimensionless temperature (θ) distributions at the X/D = 5 cut plane are compared. As the mist particles follow the coolant jet well, the patterns of the θ distribution are roughly similar for the mist and dry cases, although the magnitude is different. Due to the heat absorption of the mist, the core of the mist-injected jet has a higher θ than that in the dry jet case. Hence, when the jet is thrust down and dispersed laterally by Tu, the wall region touched by the jet core show both a wider and greater θ than that in the dry case. This combined effect increases both the spanwise and area-averaged film effectiveness, similarly to the effects of Tu and mist being multiplied. This is easily observed by comparing the dry and mist contours (upper and lower rows, respectively) in Figure . Regardless of the mist injection and blowing ratio, the coolant jets are thrust down and dispersed by the elevated Tu. However, due to the mist effect, the region touching the wall has a much higher θ than that in the dry cases. This effect is clearly visualised by comparing the M = 0.78 cases (DryT00M78, DryT20M78, D05T00M78, and D05T20M78). Due to the consistent occurrence of this effect after injection, the spanwise-averaged film effectiveness curves in Figures and exhibit an amplified trend compared to the air-only (Dry) cases.
Effect of mist diameter (dp)
To investigate the effect of mist diameter, four mist sizes (dp = 5, 10, 15, and 20 μm) were simulated under varying mainstream Tu conditions, while keeping the other parameters fixed (M = 0.78, DR = 1.3, U∞ = 20 m/s, and ). Figure provides a visual representation of the distinctions in particle distribution and the consequent variations in dimensionless temperature distribution based on the mist diameter. Notably, when comparing the smallest and largest mist diameters (5 and 20 μm, respectively), the difference in the uniformity of the particle distribution is evident. Moreover, the dimensionless temperature at each X/D cut plane is significantly higher for smaller mist diameters. These disparities affect the response to mainstream Tu, which is discussed later in this section.
Figure 18. Comparison of mist particle distributions for varying blowing ratios (M) and the corresponding dimensionless temperatures (θ) at cut planes for cases D05T00M25 and D05T00M78 (dp = 5 μm, Tu = 0.1%).

The film effectiveness, both locally and spatially, decreased as the mist diameter increased. Figure presents the contours of film effectiveness at the wall for all diameters and Tu levels. When the mist diameter was increased from 5 to 20 μm, the film effectiveness at the wall surface decreased and became comparable to that of air-only cooling. The results with dp = 20 μm only exhibited differences in the downstream region, where was slightly higher and had a wider distribution. Moreover, as the mist diameter increased, the highest effectiveness region decreased in magnitude, and the position of the peak moved downstream. In the case of 5 μm, the peak region started immediately after the hole, while in the 10 μm case, it began near X/D = 3. In the 15 μm case, the peak region was near X/D = 20, and the peak contour band was not clearly identified due to the limited differences between adjacent regions. No peak was observed in the 20 μm case. These differences in peak positions are attributed to the surface-area-to-volume ratio (SA:V) and mist distribution, which will be discussed later in this section.
Figure 19. Contours of film effectiveness at the wall for different mist diameters and Tu conditions: (a) Tu = 0.1%, (b) Tu = 20% (dp = 5, 10, 15, and 20 μm or dry).

Meanwhile, when the Tu level increased from 0.1% to 20%, all diameter levels, including those in the dry cases, exhibited wider and higher distributions. Comparing Figures (a) and (b), it can be observed that the regions with high
for the Tu = 20% cases are both wider and longer than those for the Tu = 0.1% cases. As discussed in the previous section, a higher mainstream Tu has a favourable effect, particularly when the blowing ratio is high, due to the thrust-down effect. This effect causes the coolant jet to spread laterally and adhere more closely to the wall than that in the Tu = 0.1% cases.
The spanwise-averaged film effectiveness results for each diameter are presented in Figure . As expected from the contours shown in Figure , the spanwise-averaged film effectiveness is the highest for dp = 5 μm and decreases as the diameter increases. Moreover, increases in a specific pattern for varying diameters in the mist-injected cases. This is attributed to the underlying mechanism of coolant temperature reduction by the mist. Without mist injection (the DryT00M78 case),
gradually decreases in the downstream direction due to the coolant mixing with hot gas. However, in the mist-injected cases, the presence of mist particles continually chills the coolant, allowing it to maintain a lower temperature. Consequently, when coolant with a lower temperature reattaches to the wall,
increases.
The second mechanism involves the coupled effect of the mist evaporation rate and distribution. Small particles with a high SA:V exhibit a significantly higher evaporation rate than larger particles. This disparity in SA:V leads to varying rates of evaporation within the coolant jet, as depicted in Figure . Initially, the smaller particles undergo rapid evaporation, the rate of which decreases downstream as the particles progressively evaporate. Conversely, larger particles experience little evaporation in the upstream region, but their evaporation rate gradually increases in the downstream direction. Consequently, the quickly evaporating droplets absorb more heat from the coolant, resulting in a lower coolant temperature (i.e. higher film effectiveness). Cases with larger particle sizes show a slow and late increase in .
Additionally, an increase in the mist diameter increases the inertia of the particles, resulting in different particle responses to the flow. The Stokes number (Stk), a quantitative indicator of this relationship, is used to indicate the particle characteristics (Barker et al., Citation2013; Bonilla et al., Citation2012; Terekhov & Pakhomov, Citation2009). Stk is the ratio of the particle timescale to the fluid timescale, which represent particle drag and inertia, respectively, and is defined as follows:
(15)
(15) where
represents the characteristic length scale of the fluid. As the mist particles are injected through the hole, the length scale is set as the hole diameter (D). The results of the Stokes number calculations are presented in Figure . The smallest mist particles (dp = 5 μm) have Stokes numbers significantly smaller than unity, indicating that they follow the flow well. When the diameter is increased, Stk is approximately unity at dp = 15 μm and increases significantly at 20 μm, indicating that the particles are resistant and do not follow the flow well. Consequently, the particle distribution inside the coolant jet differs with the diameter, as shown in Figure .
The particle distribution difference shown in Figure is primarily attributed to the CRVP originating from the hole. As the coolant is supplied from the plenum and injected through the hole, an in-hole CRVP is generated. Consequently, both the coolant and injected mist particles are subject to rotation by the CRVP along the coolant stream. However, due to variations in the Stk of the particles, their distribution differs. For example, when the diameter is relatively small (dp ≤ 10 μm), the particles are well distributed and follow the coolant jet, covering both the near-wall and top of the jet. In contrast, when the diameter is large (dp ≥ 15 μm), the particle distribution becomes skewed towards the upper side of the jet. Additionally, the positions of the particles being dragged down to the wall by the CRVP align with the trend of the change in Stokes number. Furthermore, this disparity in particle behaviour contributes to the difference in the increasing trend of in , as depicted in Figure . With the mist particles reaching the near-wall region, a rapid increase in
is observed, resulting in a lower temperature near the wall. It is worth noting that the difference in the gradient of
increases corresponding to the SA:V of the mist.
Figure 22. Stokes number (Stk) of mist particles used in this study (calculated from the initial mist diameter).

Figure 23. Comparison of particle distribution with varying diameters (dp = 5, 10, 15, and 20 μm). Note that the dots in the plot have a constant size and are not scaled for a simple comparison of distribution.

Meanwhile, when the mainstream Tu is increased to 20%, the changes in particle distribution vary depending on Tu at each diameter level. To analyse the coupled effect of mist diameter and mainstream Tu, the vertical particle distribution is examined using a PMF, as described in Effect of Blowing Ratio (M). Since the visibility of the trend is limited when only bar plots are used, a line plot following the top of the bar is used for comparison. Figure clearly illustrates the vertical distribution bias of the particles, characterised by the presence of a ‘crest’ in the curves, with larger particles showing a more elevated distribution. This discrepancy in distribution leads to variations in the location of the Tu effect. By comparing the solid and dashed lines in Figure , it can be observed that the disparity in the distribution caused by the Tu effect primarily occurs at the Y/D location at which the particles are predominantly distributed. Consequently, as the mainstream Tu increases, particles with a lower distribution are thrust down towards the near-wall region, while those with an upper distribution remain suspended above the near-wall region. The particles that are thrust downward near the wall contribute to an additional reduction in the coolant temperature in that region, thereby increasing the film effectiveness.
As a result, the combined effect of particle characteristics (evaporation rate and Stk) and the Tu effect leads to variations in the area-averaged film effectiveness, as depicted in Figure . When comparing the solid and dashed lines in Figure , the difference reaches its maximum and minimum at the smallest diameter (dp = 5 μm) and in the absence of mist injection (dry case), respectively. These findings suggest that the impact of mainstream Tu on film effectiveness is more pronounced for smaller particles, exhibiting greater disparities in both evaporation rate and distribution changes.
Conclusion
This study presents a novel investigation into the combined effect of mainstream Tu and mist injection in mist-assisted film cooling, utilising an idealised flat-plate geometry with a cylindrical hole. In a previous study conducted by Dwivedi and Sarkar (Citation2022), the analysis focused on the effect of Tu with identical mist parameters. However, Zhao and Wang (Citation2014b) emphasised that the injected mist particles have varying diameters and momenta, resulting in unique trajectories. Therefore, this study is the first to demonstrate the combined effect of mainstream Tu and mist momentum. The primary influencing variables investigated are the blowing ratio (M), mist diameter (dp), and mainstream Tu. To analyse the effects of Tu, M and dp were varied at fixed Tu levels of 0.1% and 20%. By primarily focusing on fundamental flow physics and the accompanying particle flow, this analysis led to the following conclusions:
In addition to the coolant jet, the injected mist particles exhibit 3-D motion owing to various flow phenomena (Tu, CRVPs, etc.). Hence, a 3-D computational analysis of mist-assisted film cooling includes realistic effects under varying conditions, especially the effect of Tu.
The film effectiveness in the mist-injected cases responds to Tu in a similar manner to that in the dry cases at the same blowing ratio. However, the presence of mist amplifies the magnitude of the Tu-induced gap due to its additional cooling effect. In particular, while the jet structures remain unchanged, the mist injection results in a decrease in coolant temperature. Furthermore, the particle flow is strongly influenced by the jet structure, exhibiting similar changes in their distribution to that of the jet structure induced by Tu. This study reveals the novel finding that, despite the varying effect of mainstream Tu on air-only cooling at low and high blowing ratios, the distribution of mist particles and corresponding film effectiveness follow the same trend as air-only cooling.
A smaller mist diameter leads to a greater enhancement of the film effectiveness by the mist. This is mainly due to differences in the SA:V and Stokes number. Smaller particles evaporate faster and are distributed more uniformly because of the counter-rotating vortex inside the coolant. Moreover, this difference caused by mist diameter also affects the effect of mainstream Tu. At elevated Tu conditions, mainstream Tu pushes the coolant jet to the wall and disperses it laterally in the investigated conditions (M = 0.78). While the increase in film cooling effectiveness is consistent for all diameter levels, the particle dynamics influence the amount changed by the Tu. Particles with a more uniform distribution are more likely to cool the near-wall region, whereas biased particles are only affected in a specific location. This leads to a reduced Tu effect in the cases with high dp.
In this study, the mist particles demonstrate a clear cooling enhancement as Tu increases. Moreover, mist-injected cases exhibit broader regions of Tu advantage compared to dry cases, despite the generally detrimental effect of Tu on film effectiveness. Therefore, mist-assisted film proves beneficial for gas turbines with highly turbulent combustor exits. This study contributes to a better understanding of the realistic applications of mist-assisted film cooling under various turbulent conditions, as the analysis shows their combined effect.
Despite the valuable insights gained from this study, certain limitations need to be addressed in future research. First, it is necessary to consider dp levels below 5 μm, as these were not simulated due to rapidly increasing computational costs. This aspect is particularly crucial since showed an increase with decreasing dp. In addition, this study focused on the combined effect of mist characteristics and mainstream Tu, without considering other parameters such as the L/D ratio, surface curvature, and nonuniform diameter injection. Incorporating these parameters is crucial for a more realistic representation of mist application in actual gas turbines. Addressing these limitations in future investigations will further enhance our understanding and application of mist-assisted film cooling.
Disclosure statement
No potential conflict of interest was reported by the author(s).
Additional information
Funding
References
- Abadi, S. M. H. B., Zirak, S., & Zargarabadi, M. R. (2020). Effect of pulsating injection and mainstream attack angle on film cooling performance of a gas turbine blade. Physics of Fluids, 32(11), Article 117102. http://dx.doi.org/10.1063/5.0029110
- Abdelmaksoud, R., & Wang, T. (2020). Simulation of air/mist cooling in a conjugate, 3-D gas turbine vane with internal passage and external film cooling. International Journal of Heat and Mass Transfer, 160, 120197. https://doi.org/10.1016/j.ijheatmasstransfer.2020.120197
- Ahn, J. (2022). Large eddy simulation of film cooling: A review. Energies, 15(23), 8876. https://doi.org/10.3390/en15238876
- ANSYS. (2021). ANSYS Fluent Theory Guide 21R1.
- Baek, S. I., & Yavuzkurt, S. (2018). Effects of oscillations in the main flow on film cooling at various frequencies at a low blowing ratio. Turbo Expo: Power for Land, Sea, and Air, https://doi.org/10.1115/GT2018-75440
- Baheri Islami, S., & Jubran, B. (2012). The effect of turbulence intensity on film cooling of gas turbine blade from trenched shaped holes. Heat and Mass Transfer, 48(5), 831–840. https://doi.org/10.1007/s00231-011-0938-x
- Barker, B., Casaday, B., Shankara, P., Ameri, A., & Bons, J. (2013). Coal ash deposition on nozzle guide vanes—part ii: Computational modeling. Journal of Turbomachinery, 135(1), 011015. https://doi.org/10.1115/1.4006399
- Bianchini, C., Andrei, L., Andreini, A., & Facchini, B. (2013). Numerical benchmark of nonconventional RANS turbulence models for film and effusion cooling. Journal of Turbomachinery, 135(4), 321. http://dx.doi.org/10.1115/1.4007614
- Bicen, A., & Jones, W. (1986). Velocity characteristics of isothermal and combusting flows in a model combustor. Combustion Science and Technology, 49(1–2), 1–15. https://doi.org/10.1080/00102208608923900
- Bonilla, C., Webb, J., Clum, C., Casaday, B., Brewer, E., & Bons, J. (2012). The effect of particle size and film cooling on nozzle guide vane deposition. Journal of Engineering for Gas Turbines and Power, 134(10), 101901. https://doi.org/10.1115/1.4007057
- Bons, J. P., MacArthur, C., & Rivir, R. (1996). The effect of high free-stream turbulence on film cooling effectiveness. Journal of Turbomachinery, 118(4), 814–825. https://doi.org/10.1115/1.2840939
- Burd, S. W., & Simon, T. W. (1997). The influence of coolant supply geometry on film coolant exit flow and surface adiabatic effectiveness. Proceedings of the ASME 1997 International Gas Turbine and Aeroengine Congress and Exhibition. Vol. 3: Heat Transfer; Electric Power; Industrial and Cogeneration.V003T09A005. https://doi.org/10.1115/97-GT-025.
- Dwivedi, A., & Sarkar, S. (2022). Effects of freestream turbulence on air-mist film cooling: Two-phase flow simulations. Turbo Expo: Power for Land, Sea, and Air, https://doi.org/10.1115/GT2022-81923
- Dwivedi, A., & Sarkar, S. (2023). Numerical simulation of two-phase flow: Air-mist film cooling over a flat plate. International Journal of Thermal Sciences, 184, 107923. https://doi.org/10.1016/j.ijthermalsci.2022.107923
- Harrison, K. L., & Bogard, D. G. (2007). CFD predictions of film cooling adiabatic effectiveness for cylindrical holes embedded in narrow and wide transverse trenches. Turbo Expo: Power for Land, Sea, and Air, https://doi.org/10.1115/GT2007-28005
- Harrison, K. L., & Bogard, D. G. (2008). Comparison of RANS turbulence models for prediction of film cooling performance. Turbo Expo: Power for Land, Sea, and Air, https://doi.org/10.1115/GT2008-51423
- Hassan, J. S., & Yavuzkurt, S. (2006). Comparison of four different two-equation models of turbulence in predicting film cooling performance. Turbo Expo: Power for Land, Sea, and Air, https://doi.org/10.1115/GT2006-90860
- Johnson, P. L., Shyam, V., & Hah, C. (2011). Reynolds-averaged Navier-Stokes solutions to flat plate film cooling scenarios (No. NASA/TM-2011-217025).
- Kohli, A., & Bogard, D. G. (1998). Effects of very high free-stream turbulence on the jet–mainstream interaction in a film cooling flow. Journal of Turbomachinery, 120(4), 785–790. https://doi.org/10.1115/1.2841790
- Leedom, D., & Acharya, S. (2008). Large eddy simulations of film cooling flow fields from cylindrical and shaped holes. Turbo Expo: Power for Land, Sea, and Air, https://doi.org/10.1115/GT2008-51009
- Li, X., & Wang, T. (2006). Simulation of film cooling enhancement with mist injection. ASME Journal of Heat and Mass Transfer, 128(6), 509–519. https://doi.org/10.1115/1.2171695
- Li, X., & Wang, T. (2007). Effects of various modeling schemes on mist film cooling simulation. ASME Journal of Heat and Mass Transfer, 129(4), 472–482. https://doi.org/10.1115/1.2709959
- Liu, G., Gong, W., Wu, H., & Lin, A. (2021). Experimental and CFD analysis on the pressure ratio and entropy increment in a cover-plate pre-swirl system of gas turbine engine. Engineering Applications of Computational Fluid Mechanics, 15(1), 476–489. https://doi.org/10.1080/19942060.2021.1884600
- Liu, R., Li, H., You, R., Tao, Z., & Huang, Y. (2023). Numerical decoupling of the effect of internal cooling and external film cooling on overall cooling effectiveness. Applied Thermal Engineering, 222, 119905. https://doi.org/10.1016/j.applthermaleng.2022.119905
- Pabbisetty, M. R., & Prasad, B. (2021). Effect of blowing ratio on mist-assisted air film cooling of a flat plate: An experimental study. Journal of Thermal Science and Engineering Applications, 13(3), 031016. https://doi.org/10.1115/1.4048209
- Pietrzyk, J., Bogard, D., & Crawford, M. (1990). Effects of density ratio on the hydrodynamics of film cooling. Journal of Turbomachinery, 112(3), 437–443. https://doi.org/10.1115/1.2927678
- Ranz, W. (1952). Evaporation from drops part II. Chemical Engineering Progress, 48(4), 173–180.
- Rao, P. M., Biswal, P., & Prasad, B. (2018). A computational study of mist assisted film cooling. International Communications in Heat and Mass Transfer, 95, 33–41. https://doi.org/10.1016/j.icheatmasstransfer.2018.03.028
- Saumweber, C., Schulz, A., & Wittig, S. (2003). Free-stream turbulence effects on film cooling with shaped holes. Journal of Turbomachinery, 125(1), 65–73. https://doi.org/10.1115/1.1515336
- Sazhin, S. S. (2006). Advanced models of fuel droplet heating and evaporation. Progress in Energy and Combustion Science, 32(2), 162–214. https://doi.org/10.1016/j.pecs.2005.11.001
- Schmidt, D. L., Sen, B., & Bogard, D. G. (1996). Film cooling with compound angle holes: Adiabatic effectiveness. Journal of Turbomachinery, 118(4), 807–813. https://doi.org/10.1115/1.2840938
- Schroeder, R. P., & Thole, K. A. (2016). Effect of high freestream turbulence on flowfields of shaped film cooling holes. Journal of Turbomachinery, 138(9), 091001. https://doi.org/10.1115/1.4032736
- Sinha, A., Bogard, D., & Crawford, M. (1991). Film-cooling effectiveness downstream of a single row of holes With variable density ratio. Journal of Turbomachinery, 113(3), 442–449. https://doi.org/10.1115/1.2927894
- Stull, R. (2011). Wet-Bulb temperature from relative humidity and air temperature. Journal of Applied Meteorology and Climatology, 50(11), 2267–2269. https://doi.org/10.1175/JAMC-D-11-0143.1
- Terekhov, V. I., & Pakhomov, M. A. (2009). Film-cooling enhancement of the mist vertical wall jet on the cylindrical channel surface with heat transfer. ASME Journal of Heat and Mass Transfer, 131(6), 062201. https://doi.org/10.1115/1.3082404
- Wang, M., Zhu, H., Liu, C., Guo, T., Zhang, L., & Li, N. (2022). Numerical analysis and design optimization on full coverage film-cooling for turbine guided vane. Engineering Applications of Computational Fluid Mechanics, 16(1), 904–936. https://doi.org/10.1080/19942060.2021.2019127
- Wang, T., & Li, X. (2008). Mist film cooling simulation at gas turbine operating conditions. International Journal of Heat and Mass Transfer, 51(21–22), 5305–5317. https://doi.org/10.1016/j.ijheatmasstransfer.2008.04.040
- Wei, J., Zhang, S., Zuo, J., Qin, J., Zhang, J., & Bao, W. (2023). Effects of combustion on the near-wall turbulence and performance for supersonic hydrogen film cooling using large eddy simulation. Physics of Fluids, 35(3), https://doi.org/10.1063/5.0139355
- Yavuzkurt, S., & Habte, M. (2008, January). Effect of pulsating injection and mainstream attack angle on film cooling performance of a gas turbine blade. Turbo Expo: Power for Land, Sea, and Air, 43147, 133–143. https://doi.org/10.1115/GT2008-50153
- Zhao, L., & Wang, T. (2014a). An experimental study of mist/air film cooling on a flat plate with application to gas turbine airfoils–part I: Heat transfer. Journal of Turbomachinery, 136(7), 071006. https://doi.org/10.1115/1.4025736
- Zhao, L., & Wang, T. (2014b). An experimental study of mist/air film cooling on a flat plate with application to gas turbine airfoils–part II: Two-phase flow measurements and droplet dynamics. Journal of Turbomachinery, 136(7), 071007. https://doi.org/10.1115/1.4025738
- Zhou, W., Chen, H., Liu, Y., Wen, X., & Peng, D. (2018). Unsteady analysis of adiabatic film cooling effectiveness for discrete hole with oscillating mainstream flow. Physics of Fluids, 30(12), 127103. https://doi.org/10.1063/1.5055028