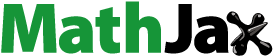
Abstract
It is noteworthy to explore potential measures for further reducing the aerodynamic drag and noise of high-speed pantographs. This paper proposes a method of introducing spanwise waviness into the upper and lower arms. The improved delayed detached eddy simulation (IDDES) method and the Ffowcs Williams and Hawkings (FW-H) equation are used for flow field simulation and sound propagation calculation, respectively. The effects of wavelength, wave amplitude and inclination on the aerodynamic force and radiated noise of the wave cylinder were firstly investigated, and then the aerodynamic characteristics of the pantograph with and without spanwise waviness configurations were compared. Results show that the appropriate spanwise waviness on the cylinder can diffuse the negative pressure core area in the wake, reduce the negative pressure amplitude, suppress the vortex shedding and weaken the fluctuation amplitude of the aerodynamic force, resulting in the reduction of aerodynamic drag and noise. For the high-speed pantograph by introducing spanwise waviness, when the ratio of wavelength and wave amplitude to cylinder diameter is 1.2 and 1/8 respectively, the aerodynamic drag and noise of the pantograph by introducing spanwise waviness are reduced by 1.58% and 1.16 dBA, respectively.
1. Introduction
The aerodynamic performance of the train is one of the key technologies in the design of high-speed trains, including basic aerodynamic characteristics and aerodynamic noise (Sun et al., Citation2013; Thompson et al., Citation2015). The energy consumption caused by aerodynamic drag is linearly related to the square of train speed, while the radiation energy of train noise increases with the sixth to eighth power of train speed. When the train speed exceeds 300km/h, the aerodynamic drag will cause significant energy loss, and the excessive noise of the train will cause noise pollution. The pantograph on the top of the train is one of the main sources of aerodynamic drag, and due to the lack of effective protection and isolation means, the noise generated by it is more significant to the surrounding environment (Chen et al., Citation2022a; Sun et al., Citation2020; Qin et al., Citation2023). It is necessary to explore some potential measures to reduce aerodynamic drag and noise for high-speed pantographs at a speed of 400km/h.
So far, research on pantographs has mainly focused on the sources, generation mechanisms, and control measures of aerodynamic drag and noise. Research efforts aimed at reducing the aerodynamic drag of pantographs have been consistently ongoing. The pantograph is mainly a complex structure composed of rods. After the airflow is disturbed by the components, a highly unstable flow field will be formed. The complex vortex structure in the wake and the high-speed airflow impact on the windward surface are the main sources of aerodynamic drag (Tan et al., Citation2018). In general, drag reduction of the pantograph system is optimized for the pantograph itself or the surrounding area. The pantograph structure is mainly divided into upper panhead area, middle knuckle area and lower bottom area. The panhead area can significantly reduce aerodynamic drag and noise by a streamlined panhead shape and panhead support structure (Ikeda et al., Citation2006). In addition, the aerodynamic drag and noise of the double-strip pantograph can be reduced by reducing the strip spacing (Dai et al., Citation2022; Li et al., Citation2020). In the knuckle area, Lee et al. (Citation2015) compared three types of pantograph structures: single-arm, double-arm, and periscope arm pantograph, and pointed out that the aerodynamic performance of single-arm pantographs is better. For the bottom area of the pantograph, the impact of the airflow on the underframe and insulator can be shielded by adding a fairing, thereby reducing the aerodynamic drag (Niu et al., Citation2017; Wu et al., Citation2020). Different forms of fairings are also installed on high-speed trains in China, such as bathtub and baffle type. Currently, the sinking of the pantograph installation platform has also become one of the main measures to reduce the aerodynamic drag of the pantograph system. This is achieved by reducing the airflow velocity around the pantograph and minimizing the impact on the windward surface of the components (Liu et al., Citation2022; Xiao et al., Citation2020).
With the increase of train speed, pantograph noise has attracted attention. Significant achievements have been made in identifying and reducing the noise sources of the pantograph. Tan et al. (Citation2019) investigated the contribution of each component of the pantograph to the total noise, and pointed out that up to 90% of the radiated noise comes from the base, insulator, balance arm, upper and lower arm. Zhang et al. (Citation2016; Citation2017) studied the formation and propagation mechanism of high-speed pantograph aerodynamic noise. The results show that panhead, base and knuckle are the main noise source components and the propagation of aerodynamic noise is dipole mode. Li et al. (Citation2022a) analyzed the flow field of pantograph. The panhead and the upper arm show tonal noise with peak frequencies of 353 and 252 Hz, respectively, which is related to the vortex shedding structure behind the pantograph. Iglesias et al. (Citation2017) proposed a semi-empirical component-based prediction model to evaluate the aerodynamic noise generated by the airflow over the pantograph by considering the pantograph as a cylinder and bar with different cross sections. Yuan et al. (Citation2021) studied the drag reduction and noise reduction of the pantograph. The results show that the radiation noise of the pantograph mainly comes from the fluctuating pressure on the surface of each component. The tonal noise generated by airflow separation on the surface of the slider can be suppressed by bionic surface microstructure optimization.
In most cases, the aerodynamic drag and noise reduction of the pantograph need to be considered simultaneously. Airflow over the surface of the rod will produce complex flow separation phenomenon, resulting in shedding and breaking of the vortex, which will cause alternating oscillating force on the surface of the rod, and finally form the aerodynamic noise source. The research on the flow control of the rod is of great significance to realize the aerodynamic drag and noise reduction of the pantograph. Choi et al. (Citation2008) studied flow control methods for flow around bluff bodies and pointed out that providing appropriate active or passive control of the separated shear layer of a cylinder can significantly reduce drag and lift fluctuations. King and Pfizenmaier (Citation2009) investigated the aeroacoustic characteristics of rods with different cross-sectional shapes (including circular, square, rectangular and elliptical). The results show that the noise of elliptical section is the smallest. In addition, the addition of lateral ribs and knurled surfaces to the cylinder can significantly reduce noise. Zhang et al. (Citation2020) studied the effect of non-uniform porous coating on the aerodynamic drag and noise of cylindrical rods. The application of porous materials with high porosity in the flow separation zone can increase the basic pressure of the wake and suppress the vortex shedding to achieve drag reduction and noise reduction of the cylindrical rod. In addition, Shi et al. (Citation2012) and Liu et al. (Citation2012) proposed to reduce aerodynamic noise by attaching bionic serrated structures and open-cell metal foam to the surface of a cylinder, respectively. Both measures can change the vortex shedding frequency and control the oscillating force acting on the cylindrical rod, thereby reducing the aerodynamic noise. Liu et al. (Citation2018) studied the aerodynamic noise of a square rod, and proposed that the application of waviness to the surface of the square rod would effectively suppress the shedding of the vortex and greatly reduce the noise level.
For high-speed trains with increasing running speed, pantograph sinking installation, base frame streamlined or additional fairing have been widely used. The effect of the frame system structure will become more and more prominent when the aerodynamic drag and noise of the panhead and the base frame are well controlled (Yao et al., Citation2015). Although there are many technologies that can be used on the upper and lower arm rods to achieve drag reduction and noise reduction, such as porous materials, blowing and suction, bionic structures (Chen et al., Citation2022b; Wu, Citation2017). However, these studies mainly focus on the panhead, and the attention to the aerodynamic drag and noise generated by the frame system is not enough. In addition, there are few studies on whether some drag reduction and noise reduction technologies are applicable to the frame system. Therefore, some potential measures can be explored for the frame system to achieve further drag and noise reduction of the high-speed pantograph. In this paper, the spanwise waviness structure is introduced into the upper and lower arms. Firstly, based on the cylindrical rod model, the influence of waviness parameters on the aerodynamic characteristics of the rod is studied and the waviness parameters are optimized. Then, the effect of inclination is considered. Finally, the spanwise waviness structure is applied to the high-speed pantograph to further explore its aerodynamic drag reduction and noise reduction characteristics. The research achievements can provide reference for further aerodynamic drag and noise reduction design of high-speed pantograph.
2. Numerical method
2.1. IDDES
The appropriate turbulence model should be selected to simulate the turbulent flow field around the pantograph. The details of flow separation and vortex shedding in the flow field need to be captured. Meanwhile, numerical simulation needs to consider the computational efficiency and accuracy. The Reynolds averaged Navier-Stokes (RANS) turbulence model is obviously not enough to obtain a high-precision flow field. Although the large eddy simulation (LES) method can better simulate the pressure pulsation on the surface of the object to obtain ideal results, its computational efficiency has hindered its widespread use. A hybrid RANS/LES method, which can obtain high-precision flow field results and meet the needs of computational efficiency, is called detached eddy simulation (DES) method. Some detailed information can be obtained in the literature (Dong et al., Citation2022; Yao et al., Citation2020).
In practical application, the standard DES method has been found to have problems such as logarithmic layer mismatch and grid-induced separation. Therefore, some improved models have been proposed. Improved delayed detached eddy simulation (IDDES) combined with DDES and wall-modelled LES (WMLES) has successfully solved the appeal problem and is now widely used to simulate unstable and separated turbulent flows at high Reynolds numbers (Shur et al., Citation2008). In this paper, the IDDES method based on SST k-ω model is used to simulate the flow field.
2.2. Noise prediction
The Ffowcs Williams and Hawkings (FW-H) equation can be used to predict far-field noise, and some detailed information can be found in Refs. (Li et al., Citation2022b). In the flow with small Mach number (Ma < 0.3), the quadrupole noise generated by the object is usually much smaller than the dipole noise. The fluctuating pressure on the surface of the object is the main sound source. However, when high-speed trains run at speeds of 400 km/h and above, quadrupole noise should be considered to obtain more accurate noise prediction results. The dipole and quadrupole noises caused by the motion of an object can be obtained by integral solution of FW-H equation based on solid surface or permeable surface. Spalart et al. (Citation2019) compared and analysed the differences between the two methods in the calculation results. It is pointed out that the integral method using permeable surface is more accurate in predicting far-field noise. However, there is no unified conclusion on the selection of the position and size of the permeable surface. In many cases, it is determined by the calculation case. In this paper, two integral methods that use solid surface and permeable surface to predict far-field noise, the size and location of the permeable surface can be seen in Section 4.4.
The step-by-step method is used in the prediction of aerodynamic noise. The fully developed steady flow field is obtained by steady calculation, which provides the initial field data for the subsequent unsteady flow field calculation. The IDDES model was selected as the turbulence model to calculate the unsteady flow field. When the calculation results of the unsteady flow field tend to be stable, the sound source is extracted, and finally the K-FWH equation is used to solve the sound propagation. The convection term and momentum term of the governing equation are solved by second order discrete scheme. The time discretization uses an implicit second-order scheme with a time step of 1 × 10−4 s. Generally, the turbulence effect of the incoming flow can be described by defining the turbulence intensity and characteristic scale of the inlet boundary. The turbulence intensity can be obtained according to the Reynolds number of the flow field, and the characteristic scale is generally selected as the height of the pantograph. The unsteady flow field calculation was performed 3500 iterations, and the simulation time was 0.35 s. When calculating the sound propagation, the sampling frequency is consistent with the time resolution of the flow simulation. The sound source is extracted for 4000 iterations, and the simulation time is 0.4 s. The total duration of the unsteady flow field simulation is 0.75 s.
3. Effect of waviness on the aerodynamic drag and noise of cylinders
In the following, the influence of wave amplitude and wavelength on the aerodynamic performance of wave cylinder is investigated, and the effect of inclination is discussed.
3.1. Numerical geometry and information of cylinders
In this paper, a cylindrical rod with diameter D = 0.08 m and length l = 16D is considered for simulation, as shown in Figure . The selection of the cylinder is based on the consideration of the actual dimensions of the upper and lower arms of the pantograph. The diameter of the wavy cylinder is.
(1)
(1) Where
is the base cylinder diameter, ω = 2A is wave amplitude, λ is the wavelength. The minimum diameter of the wavy cylinder is D to ensure that the strength is not reduced.
A hexahedral box is selected as the computational domain for numerical simulation. The definition of the coordinate system and the origin position are shown in Figure , and the cylinder is placed inside the calculation domain. The inclination of the cylinder is defined by the angle θ, which represents that the airflow acts on the object with different inflow angles. At the upstream boundary 25D from the cylinder, the far-field boundary condition with a Mach number of 0.326 is applied. The downstream boundary from the cylinder 50D is set as a pressure outlet. Symmetrical and periodic boundary conditions are applied on both sides of the computational domain and the upper and lower surfaces respectively. The use of periodic boundary can simulate a cylinder with infinite span.
To eliminate the influence of the number of grids, different mesh sizes are set to discretize the computational domain around the cylinder. A total of three sets of grids are used for numerical calculation, and the results are shown in Table . Compared with the fine grid, the error of the prediction results of the time-averaged aerodynamic drag coefficient (Cd) of the cylinder on the coarse grid and the medium grid is within 2%. However, when predicting the vortex shedding frequency, the medium grid shows more accurate results. Therefore, the medium grid is selected for subsequent numerical calculations.
Table 1. Grid independence test of cylinder.
The mesh results are shown in Figure . Two refinement boxes are created around the cylinder to ensure the mesh quality, and the boundary layer mesh is generated on the cylinder wall. The height of the first grid layer perpendicular to the wall is set to 0.002 mm to ensure that the dimensionless y + value is less than 1. In addition, the growth rate of the boundary layer grid is set to 1.1 to make the grid change evenly. For the flow simulation of all wavy cylinders, the same meshing strategy is used.
3.2. Numerical validation
Based on open access experimental data (Jacob et al., Citation2005; Yu et al., Citation2013; Zhang et al., Citation2017), a cylinder with a diameter of d = 10mm and an incoming flow velocity of U0 = 72m/s was selected for numerical simulation. The Reynolds number Re based on rod diameter is about 4.8 × 104. Previous studies (Szepessy, Citation1994) have shown that in the flow around a cylinder with Re = 4.8 × 104, the spanwise correlation length of the cylinder is found to be 2.7d. When the span of the simulated cylinder is greater than the spanwise correlation length, the simulation can capture the important characteristics of the vortex structure, and the change of the surface pressure is no longer affected by the simulated length. Therefore, a numerical simulation of flow around a cylinder with a finite span of π d is established, and the flow is assumed to be periodic in the spanwise direction to eliminate end effects. In addition, the cylinder is in the subcritical flow region (103 < Re < 2 × 105), where the vortex shedding periodically, the wake is turbulent, but the boundary layer separation is still laminar. Vortex shedding occurs in the wake at a frequency of approximately f0 = 1.4 kHz, which corresponds to a Strouhal number St = f0d/U0 = 0.20. The geometric model and meshing results are shown in Figure . In addition to considering a three-dimensional circular calculation domain, the boundary conditions of the calculation domain are consistent with Section 3.1. The meshing ensures that the dimensionless quantity y + of the wall is less than 1 to meet the requirements of the turbulence model for mesh quality.
For the predicted results of surface pressure coefficient (Cp), the comparison between numerical simulation and experiment is shown in Figure . It can be seen that for a benchmark problem, we and Yu et al. (Citation2013) obtained similar results for the same numerical simulation condition, and basically coincided with the experiment.
In acoustic prediction, the time step for both unsteady flow field and aeroacoustic noise calculation is set to 1 × 10−4 s, simulating 0.25 s respectively. The total physical time simulated is 0.5 s, ensuring a maximum frequency of 5 kHz and a frequency resolution of 4 Hz for dynamic behaviour characterization. Based on the principle of sound source energy superposition, the noise of a cylinder with a finite span of π d is analogized and extrapolated to obtain the noise of a real span of 30d in the experiment. The acoustic results of the far-field sound pressure level spectrum varying with Strouhal number at a distance of 185d from the cylinder are shown in Figure . Numerical simulations and experiments show that the peak difference of the noise spectrum around St = 0.197 is about 2 dB, and there is a certain frequency shift. This is due to the influence of frequency resolution. Similar to the results of Yu et al. (Citation2013) and Zhang et al. (Citation2017), three main frequency peaks of vortex shedding are obtained, which are in good agreement with the experimental results. This shows that the hybrid calculation method in this paper has high accuracy in aerodynamic drag and noise prediction.
3.3. Effect of wave parameters on the flow around a cylinder
Table shows the aerodynamic drag coefficients and acoustic results of wavy cylinders with different wave amplitude. The coordinates of the noise receiver are (0, 20D, 8D). The simulation of the bare cylinder is used as the base case, and the simulations of the other five wavy cylinders with different wave amplitudes (λ/D = 0.8, ω/D = 1/16, 1/10, 1/8, 1/4 and 1/2) are used as comparison cases. It can be obtained that at the same wavelength, as the increase of wave amplitude, the Cd of the wavy cylinder decreases first and then increases. Compared with the bare cylinder, the aerodynamic drag coefficients of all wavy cylinders increase. For aerodynamic noise, the wavy cylinders are greatly reduced. For all cases, the sound pressure level (SPL) at the acoustic receiving point is reduced by more than 12.8 dB. When λ/D = 0.8 and ω/D = 1/8, the noise reduction is the most, which is 18.3 dB, while the aerodynamic drag increases by only 1.5%.
Table 2. Aerodynamic drag and noise of wavy cylinder with different wave amplitudes.
To investigate the source of the increased aerodynamic drag of the wavy cylinder, the time-averaged pressure coefficient along the z = 8D curve on the cylinder surface is compared, as shown in Figure . The aerodynamic drag of the cylinder mainly comes from the pressure difference between front and rear surfaces. For all cases, the difference in pressure on the leeward side of the cylinder is greater than that on the windward side, and the pressure change trend does not show consistency. With the increase of the wave amplitude, the maximum negative pressure amplitude on the surface of the cylinder decreases first and then increases. Compared with the bare cylinder, the maximum negative pressure amplitude on the wavy cylinder surface decreases, but the overall negative pressure on the leeward side rises slowly, resulting in greater aerodynamic drag.
When ω/D = 1/8, the aerodynamic performance of the cylinder is better. Therefore, four other wavy cylinders with different wavelengths were added for study, and the results are shown in Table . Compared with the bare cylinder, when λ/D is greater than 1.2, the Cd and SPL of the wavy cylinder are reduced. With the increase of λ/D, the Cd of the wavy cylinder changes little, while the aerodynamic noise increases. Overall, when ω/D = 1/8 and λ/D = 1.2, the wavy cylinder achieves a drag reduction of 6.3% and a noise reduction of 19.9 dB, reflecting better aerodynamic performance.
Table 3. Aerodynamic drag and noise of wavy cylinder with different wavelengths.
Figure shows the comparison of the Cp on the cylindrical surface. The change of wavelength leads to the difference of the position of the airflow separation point, resulting in the change of the pressure on the windward and leeward sides of the wavy cylinder. Compared with the bare cylinder, the positive pressure area on the surface of the wavy cylinder increases, but the negative pressure area and amplitude are greatly reduced, resulting in a decrease in the resistance of the wavy cylinder.
The influence mechanism of waviness on flow field can be obtained by further analysis of flow characteristics. Figure shows the flow on the z = 8D plane, coloured by the time-averaged pressure coefficient. Compared with the bare cylinder, the wake of the wavy cylinder has changed significantly. The leeward side of the cylinder is the core area of the strong negative pressure. With the change of ω/D, the spanwise waviness attached to the cylinder increase the negative pressure region in the wake, but the negative pressure core area diffuses and the amplitude decreases. However, the resulting reductive resistance is greater than that of the bare cylinder. With the increase of λ/D, the position of the separation point on the cylinder surface is changed by the waviness, which leads to the difference of vortex pattern in the wake. The interaction between the shedding vortices suppresses the generation of large-scale vortices in the wake, which leads to the decrease of the separation region and finally achieves drag reduction.
Figure shows the time-averaged turbulent kinetic energy (K) distribution in the z = 8D plane. In the wake, the turbulent kinetic energy near the surface of the cylinder is stronger. The spanwise waviness attached to the cylinder will affect the turbulent kinetic energy distribution in the wake, pushing the high turbulent kinetic energy region away from the cylinder surface. It is worth noting that the range of high turbulent kinetic energy in the wake of the wavy cylinder will increase compared with the bare cylinder, but the intensity will decrease. In general, the turbulent fluctuation intensity around the wavy cylinder is weaker, resulting in lower turbulent radiation noise.
The generation of aerodynamic radiation noise can be related to the lateral force oscillation mode caused by the alternating vortex shedding on the cylindrical surface. For the selected cases, the cylinder transient lateral force coefficient (CL) is shown in Figure (a). It can be seen that the lateral force has quasi-periodic fluctuation characteristics with different amplitudes. Compared with the bare cylinder, the fluctuation amplitude of the wavy cylinder is smaller. Comparing all cases, the lateral force coefficient fluctuation of the wavy cylinder is reduced by 36.2%∼57.5%, which indicates that the alternating vortex shedding strength on the surface of the cylinder is reduced. The power spectral density (PSD) corresponding to the lateral force coefficient is shown in Figure (b). The obvious dominant frequency characteristic appears on the bare cylinder, while the dominant frequency peak of the wavy cylinder is greatly reduced. The obvious dominant frequency characteristics appear on the bare cylinder. However, the peak frequency and amplitude of the wavy cylinder are greatly reduced. This indicates that there is a change in the wake and the interaction between vortices may induce a new vortex pattern.
Figure 11. Comparison of aerodynamic lateral force coefficient. (a) Transient lateral force coefficient: (b) Power spectrum density.

To evaluate the effect of waviness on the cylinder, Figure shows a comparison of the overall sound power level (OASPL) around the cylinder. The spanwise waviness attached to the cylinder greatly reduces its noise radiation level. In the vertical direction of the airflow, the OASPL is reduced by 20 dB at most and by 8.67 dB at least in the streamwise direction.
3.4. Effect of inclination on the flow around a wavy cylinder
As the upper and lower arms of the pantograph are inclined to incoming flow, it is necessary to investigate the effect of inclination. At different inclination angles θ, the bare cylinder and the wavy cylinder with waviness parameters ω/D = 1/8 and λ/D = 1.2 are used for simulation. Figure shows the aerodynamic and acoustic results of bare and wavy cylinders at different inclination angles. Compared with the bare cylinder, the aerodynamic drag of the wavy cylinder decreases by 0.3% to 6.4% when the inclination angle is between 45° and 135°. However, as the inclination angle continues to decrease or increase, the wavy cylinder leads to greater aerodynamic drag. For acoustic comparison, wavy cylinders show different degrees of noise reduction. The sound pressure level is reduced by 14.4∼19.9dB. Overall, when the cylinder is perpendicular to the incoming flow (θ = 90°), the wavy cylinder has the best drag and noise reduction effect. A larger or smaller inclination angle will lead to an increase in resistance and attenuate the noise reduction effect.
Figure 13. Effect of inclination on aerodynamic and acoustic results. (a) Aerodynamic drag coefficient: (b) Aerodynamic radiation noise.

Figure shows the flow field around different cylinder models in the inclined state, coloured by pressure. The inclination of the cylinder will cause the airflow to flow along the surface. The wavy cylinder has more vortices near the leeward side surface in the wake, and the interaction between the vortices is more complex. Positive pressure can be observed on the leeward surface of the wave cylinder, which is beneficial to drag reduction. However, the positive pressure area on the windward surface of the inclined wave cylinder is significantly larger than that of the bare cylinder, which has a negative effect on reducing the aerodynamic drag. When the angle θ between the incoming flow and the cylinder is within a certain range, the pressure-induced resistance on the surface of the wavy cylinder is lower. At other inclination angles, local high pressure will be caused, resulting in excessive aerodynamic drag.
Figure 14. Flow field around different cylinder models. (a) Base cylinder, θ = 45°: (b) Wavy cylinder, θ = 45°.

Figure further shows the time-averaged vorticity in the plane z = 8D. It can be seen that there are vortices with opposite rotation directions on both sides of the cylinder periodically shedding from the surface. Compared with the bare cylinder, the waviness reduces the recirculation region in the wake by changing the position of the flow separation point. When the cylinder rod is perpendicular to the incoming flow, the vorticity intensity in the wake of the wave cylinder decreases significantly due to the vortex interaction caused by the waviness. However, as the cylinder tilts, the effect of waviness decreases.
Figure 15. The time-averaged vorticity in the z = 8D plane. (a) Base cylinder, θ = 90°: (b) Wavy cylinder, θ = 90°: (c) Base cylinder, θ = 45°: (d) Wavy cylinder, θ = 45°.

Figure shows the instantaneous vortex structure based on Q criterion. It can be seen that compared with the bare cylinder, the vorticity near the near-wall region of the wavy cylinder is significantly reduced. The waviness changes the diameter of the cylinder in the spanwise direction, and the shedding vortices generated at different airflow separation points interfere with each other. Therefore, small-scale vortices will appear and further consume the energy of mainstream large-scale vortices. According to the theory of vortex sound, weaker vortex will produce lower radiated noise.
4. Results of pantographs with or without spanwise waviness
From the above discussion and analysis, the introduction of waviness into the cylinder can reduce aerodynamic drag and radiation noise. Although the effect of inclination will attenuate the ability of waviness to reduce drag and noise, the flexible and adjustable operating height of the pantograph will lead to different degrees of inclination of the upper and lower arms. By introducing spanwise waviness, the aerodynamic performance of high-speed pantograph can be further improved. Therefore, the aerodynamic drag and noise characteristics of high-speed pantograph by introducing spanwise waviness are compared and discussed.
4.1. Numerical geometry and information of pantograph
The pantograph model used for simulation calculation is shown in Figure . The pantograph is mainly composed of three parts: panhead, frame system (knuckle, upper and lower arms) and base frame (base and insulator). Two frame system configurations are used for simulation. The first structure is characterized by the upper and lower arms composed of bare cylinders, named M1. The second structure is shown in Figure (b), named M2, and the upper arm and lower arm are modified to be wavy cylinders. The average diameter of the upper and lower arms is about 0.08 m. The spanwise waviness parameters ω/D and λ/D attached to the cylinder are 1/8 and 1.2, respectively.
The pantograph is placed on the top of the train. As shown in Figure , the train body is approximated to shorten the calculation time. The three-dimensional size of the pantograph is L = 2.45 m, W = 1.95 m, H = 1.65 m. The size of the computational domain is 40L × 16W × 8H. The slip wall is applied to the bottom of the computational domain. The slip velocity is equal to the train speed, and the direction is opposite to the train running direction. At the top and both sides of the boundary, symmetry boundary are used. Pantograph and train body surface are set as a non-slip wall. The inlet and outlet boundary settings of the computational domain are consistent with Section 3.1.
The grid independence test has been completed in Reference (Qin et al., Citation2020). Two refinement boxes are used around the pantograph to generate a higher resolution mesh. The refinement area is shown in Figure . To capture the flow details on the surface of the pantograph, a total of 18 layers of boundary layer meshes are applied around the pantograph surface. The height of the first layer grid is 0.005 mm to ensure that the dimensionless y + value of the wall is less than 1. In addition, the growth rate of the grid is set to 1.1 to ensure a uniform transition of the grid. The computational grid for simulation is shown in Figure .
4.2. Numerical validation
Wind tunnel experiment can provide experimental basis and data support for the evaluation and improvement design of high-speed pantograph. We have conducted aerodynamic noise test verification for pantographs with speeds below 350km/h, and detailed information can be found in (Li et al., Citation2022b). Due to the lack of aerodynamic noise tests for high-speed trains with speeds exceeding 400km/h, we chose to use aerodynamic tests of high-speed pantographs as a comparison. The test was carried out in the FL-26 wind tunnel of China Aerodynamic Research and Development Center, as shown in Figure . For the validation of numerical methods, the same model configurations as the wind tunnel tests were established for simulation. Two states of the pantograph are simulated, one folded and one folded. Due to the complex geometric structure of the pantograph, it is difficult to select a suitable characteristic area. Generally, the cross-sectional area of the train (A = 10 m2) can be considered as a reference value for the characteristic area of the pantograph. Compared with the test results, the maximum error of the aerodynamic drag of the pantograph obtained by simulation is less than 6%, as shown in Figure . Overall, the IDDES hybrid calculation method can better predict the aerodynamic characteristics of high-speed pantographs.
4.3. Aerodynamic force
The aerodynamic drag results of pantograph with different configurations are shown in Table . Compared with M1 model, the aerodynamic drag of upper, lower arms and knuckle is smaller for M2 model. The aerodynamic drag of the frame system and the pantograph decreased by 13.64% and 1.58% respectively. The time-averaged pressure coefficient contours on the longitudinal centre section of the pantograph are shown in Figure . The near wall airflow is guided to the knuckle by the upper and lower arms composed of bare cylinders, resulting in the formation of a high pressure region. It can be seen that for the M2 model, the positive pressure amplitude of the knuckle region is greatly reduced. Although the spanwise waviness attached to the cylinder produces a positive pressure effect at a local position, it destroys the guiding mode of the upper and lower arms to the airflow, and has a positive effect on reducing the negative pressure on the leeward side, resulting in a decrease in resistance.
Figure 22. Time averaged pressure contours on longitudinal central section of pantograph. (a) M1: (b) M2.

Table 4. Comparison of aerodynamic drag of pantograph with different configurations.
Figure shows the time history curve and power spectral density of the aerodynamic lateral force coefficient of the frame system (the characteristic area is selected as the cross-sectional area of the train, and the value is 10m2). The fluctuation amplitude of the CL of the M1 model is larger, and the PSD has a peak characteristic at 386 Hz. For the M2 model, the fluctuation amplitude of the aerodynamic lateral force is attenuated by 38.5%, and the dominant frequency characteristics of the power spectrum disappear.
4.4. Aerodynamic noise
The aerodynamic noise of pantograph mainly comes from the flow separation, vortex shedding and fluctuating pressure on the surface of each component. The theory of vortex sound can be used to understand the formation mechanism of noise induced by turbulent vortex motion in the flow field around pantograph. The distribution of volume dipole source (intensity defined as) and volume quadrupole source (intensity defined as
) around the high-speed pantograph is shown in Figure and Figure , respectively. Flow separation on the surfaces of the panhead, upper arm and lower arm causes alternating vortex shedding to form a volume dipole sound source as shown in Figure . In the near-wall flow field near the rod, the flow separation produces strong fluid interaction, and the vortex motion is intense, resulting in a strong body dipole sound source. Compared with the M1 model, the spanwise waviness causes more small-scale separation vortices around the frame system of the M2 model, which reduces the generation of large-scale separation vortices and eventually leads to the decrease of the volume dipole source intensity in the near-field separation region. Compared with the volume dipole sound source, the intensity of the volume quadrupole sound source is lower, which can be seen in Figure . This indicates that the volume dipole sound source formed by the strong fluid interaction of turbulent vortex motion, vortex line deformation and rupture is more efficient in converting flow energy into sound energy. After the upper arm and the lower arm of the pantograph are attached to the spanwise waviness, the further generation of large-scale vortices is suppressed and the aerodynamic noise radiation is reduced due to the coherence between the vortex shedding.
The far-field radiation noise of high-speed pantograph can be obtained by solving FW-H equation. When the acoustic integral surface adopts the pantograph surface, the radiation aerodynamic noise of the dipole source on the pantograph surface is calculated. When the permeable acoustic integral surface surrounding the pantograph is adopted, the radiation noise including the dipole sound source on the pantograph surface and the spatial quadrupole sound source is calculated. The permeable acoustic integral is to establish a control surface including the main flow-induced sound source in the computational domain. A larger control integral surface can obtain more accurate prediction results. However, to balance the computational efficiency and accuracy, it is necessary to create a finite size but suitable integral control surface. Figure shows the vorticity distribution around the pantograph. The vorticity of the typical position in the flow field is extracted, and the vorticity development curve is shown in Figure . When the vorticity is small enough and basically unchanged, it can be considered that there is no acoustic disturbance source in the flow field. Finally, the size of the permeable integral surface is 8.5L × 1.5W × 1.2H.
Referring to the relevant international standard ISO3095-2013, the acoustic receiver used to evaluate the far-field noise characteristics of high-speed pantograph can be arranged 25m away from the centre line of the track. As shown in Figure , a total of 27 acoustic receivers are arranged at an interval of 2m from the ground 3.5m. Figure shows the sound pressure level results obtained using different acoustic integral surfaces. Compared with using the pantograph surface as the integral surface, when using the permeable acoustic integral surface, the radiation noise is larger (the maximum difference of the receiver is 4.65 dBA), and the receiver position is mainly located downstream of the pantograph wake. Compared with the M1 model, when the pantograph surface is used as the acoustic integral surface, the maximum radiation noise of the M2 model is reduced by 0.88 dBA, and the average sound pressure level of all receivers is reduced by 1.01 dBA. When the penetrable surface is used as the acoustic integral surface, it is reduced by 1.62 and 1.16 dBA, respectively.
Both far-field noise prediction methods show that the radiation noise of M2 model is lower. The integral method based on solid surface can further analyse the noise contribution. For different components as aerodynamic noise sources, the radiated noise results of some receivers (near the pantograph, other receivers can obtain similar conclusions) are shown in Table . More than 80% of the radiated noise comes from the panhead and base frame. However, the contribution of frame system to total noise is less than 20%. Compared with M1 model, the radiation noise of frame system of M2 model is reduced by 1.68, 2.10 and 2.42 dBA respectively. Although the panhead produces the main noise, the wavy frame system not only improves its own aeroacoustic performance, but also reduces the radiated noise of the base frame, resulting in the reduction of the whole pantograph noise.
Table 5. OASPL and contribution rate of different sound receivers.
For the M1 model, the spectrum results at the receiver y10 with different components as aerodynamic noise sources are shown in Figure (a). It can be seen that the radiated noise energy of the panhead and the base frame is distributed in different frequency bands of 150–800 Hz and below 200 Hz, respectively, and the panhead shows tonal noise characteristics with 340 Hz. In addition, the radiated noise of the frame system also has tonal noise characteristics with a peak frequency of about 370 Hz, but the SPL is significantly lower than the noise generated by other components. This indicates that the noise generated by the frame system may be worthy of attention when the noise of other components is controlled. Figure (b) shows the spectrum comparison at the far-field receiver for different frame system configurations. The spanwise waviness can suppress the generation of tonal noise. Tonal noise control of the strips and the upper and lower arms should be considered simultaneously when designing noise reduction for higher speed pantographs.
Figure 30. Comparison of sound pressure level spectrum. (a) Each component of the M1 model as sound source: (b) Frame system as sound source.

Overall, we investigate and discuss the application of waviness to the upper and lower arms of the pantograph for aerodynamic drag and noise reduction. From the results, the design of appropriate wave parameters to optimize the shape of the wavy cylinder can achieve better drag and noise reduction. In practical applications, it is necessary to consider comprehensively the manufacturing process of the wavy cylindrical structure and the firmness and durability of the structure. In addition, wind tunnel testing can be conducted to ensure its performance and safety when the demand is met.
5. Conclusions
The method of simulating flow field by IDDES and predicting far field noise by FW-H equation is verified by experimental data. The influence of spanwise waviness on flow characteristics is compared and discussed. The conclusions are as follows:
Compared with the bare cylinder, the spanwise waviness attached to the cylinder can diffuse the negative pressure core area in the wake and reduce the negative pressure amplitude, resulting in a decrease in aerodynamic drag. In addition, the fluctuation amplitude of the lateral force is greatly reduced by suppressing the shedding of the mainstream vortex, thereby achieving a decrease in the radiated noise level. The effect of wave amplitude on aerodynamic drag and noise of wavy cylinder is greater than that of wavelength. When ω/D and λ/D are 0.8 and 1.2 respectively, the aerodynamic performance of wavy cylinder is better. Aerodynamic drag and noise are reduced by 6.3% and 19.9 dB, respectively.
The spanwise waviness induces a new vortex mode by changing the airflow separation on the cylinder surface. The waviness makes the diameter of the wavy cylinder change, which causes the vortex to randomly generate along the spanwise direction. The shedding vortices of different scales interact strongly. In the near-wall flow field, the small-scale vortices consume the mainstream energy and suppress the generation of large-scale vortices, thereby eliminating the tonal noise of the flow around the cylinder. The effect of inclination will attenuate the ability of waviness to reduce drag and noise. However, for all cases, the introduction of waviness results in noise reduction.
The near-wall airflow around the frame system is guided to the knuckle by the upper and lower arms composed of bare cylinders to form a high-pressure zone. However, the spanwise waviness attached to the frame system can destroy the guiding effect, resulting in a decrease in the aerodynamic drag of the frame system and the whole pantograph by 13.64% and 1.58%, respectively. For the quadrupole noise formed in the near-wall flow field, the volume dipole sound source is higher than the volume quadrupole sound source. The sound source intensity of the volume dipole around the M2 model is lower than that of the M1 model, and the aerodynamic radiation noise of the frame system and the whole pantograph is reduced by 2.42 and 1.16 dBA, respectively.
The radiated noise of panhead and frame system has the characteristics of tone noise, but the sound pressure level of frame system is low. The radiation noise of pan-head and base frame contributes more than 80% to the total noise. The modified upper and lower arms improve the acoustic performance of the frame system, base frame and overall pantograph.
In this study, considering the quadrupole sound source, the spanwise waviness is introduced into the cylinder and pantograph to reduce the aerodynamic drag and noise. The actual installation form of the pantograph, such as the sinking of the installation platform and the existence of the car body, may affect the effect of structural optimization. In contrast, only the isolated pantograph model is considered in the paper. Further research could investigate the integrated effects of train geometry and pantograph. Experimental verification can be conducted if the requirements for aerodynamic noise testing are met. The future research direction is to explore some potential measures to reduce drag and noise, such as active jet control, blowing and suction, bionic structures and new materials.
Disclosure statement
No potential conflict of interest was reported by the author(s).
Additional information
Funding
References
- Chen, D. W., Sun, Z. X., Yao, S. B., Xu, S. F., Yin, B., Guo, D. L., Yang, G. W., & Ding, S. S. (2022a). Data-driven rapid prediction model for aerodynamic force of high-speed train with arbitrary streamlined head. Engineering Applications of Computational Fluid Mechanics, 16(1), 2191–2206. https://doi.org/10.1080/19942060.2022.2136758
- Chen, Z. W., Ni, Y. Q., Wang, Y. Wu, Y. Wang, S. M., Liu, T. H. (2022b). Mitigating crosswind effect on high-speed trains by active blowing method: a comparative study. Engineering Applications of Computational Fluid Mechanics, 16(1), 1064–1081. https://doi.org/10.1080/19942060.2022.2064921
- Choi, H., Jeon, W. P., & Kim, J. (2008). Control of flow over a bluff body. Annual Review of Fluid Mechanics, 40(1), 113–139. https://doi.org/10.1146/annurev.fluid.39.050905.110149
- Dai, Z. Y., Li, T., Deng, J., Zhou, N., & Zhang, W. H. (2022). Effect of the strip spacing on the aerodynamic performance of a high-speed double-strip pantograph. Vehicle System Dynamics, 60(10), 3358–3374. https://doi.org/10.1080/00423114.2021.1945117
- Dong, T. Y., Minelli, G., Wang, J. B., Liang, X. F., & Krajnović, S. (2022). Numerical investigation of a high-speed train underbody flows: Studying flow structures through large-eddy simulation and assessment of steady and unsteady reynolds-averaged navier–stokes and improved delayed detached eddy simulation performance. Physics of Fluids, 34(1), 0015126. https://doi.org/10.1063/5.0075617
- Iglesias, E. L., Thompson, D. J., & Smith, M. G. (2017). Component-based model to predict aerodynamic noise from high-speed train pantographs. Journal of Sound and Vibration, 394, 280–305. https://doi.org/10.1016/j.jsv.2017.01.028
- Ikeda, M., Suzuki, M., & Yoshida, K. (2006). Study on optimization of panhead shape possessing low noise and stable aerodynamic characteristics. Quarterly Report of RTRI, 47(2), 72–77. https://doi.org/10.2219/rtriqr.47.72
- Jacob, M. C., Boudet, J., Casalino, D., & Michard, M. (2005). A rod-airfoil experiment as a benchmark for broadband noise modeling. Theoretical and Computational Fluid Dynamics, 19(3), 171–196. https://doi.org/10.1007/s00162-004-0108-6
- King, W. F., & Pfizenmaier, E. (2009). An experimental study of sound generated by flows around cylinders of different cross-section. Journal of Sound and Vibration, 328(3), 318–337. https://doi.org/10.1016/j.jsv.2009.07.034
- Lee, Y., Rho, J., Kim, K. H., Lee, D. H., & Kwon, H. B. (2015). Experimental studies on the aerodynamic characteristics of a pantograph suitable for a high-speed train. Proceedings of the Institution of Mechanical Engineers, Part F: Journal of Rail and Rapid Transit, 229(2), 136–149. https://doi.org/10.1177/0954409713507561
- Li, Q. L., Li, Z. M., Ouyang, M. H., & Yang, Z. G. (2022a). Coherence and reduced order analyses of flow field and aerodynamic noise for full-scale high-speed trains pantograph. Applied Acoustics, 193, 108777. https://doi.org/10.1016/j.apacoust.2022.108777
- Li, T., Qin, D., Zhang, W. H., & Zhang, J. Y. (2020). Study on the aerodynamic noise characteristics of high-speed pantographs with different strip spacings. Journal of Wind Engineering and Industrial Aerodynamics, 202, 104191. https://doi.org/10.1016/j.jweia.2020.104191
- Li, T., Qin, D., Zhou, N., Zhang, J. Y., & Zhang, W. H. (2022b). Numerical study on the aerodynamic and acoustic scale effects for high-speed train body and pantograph. Applied Acoustics, 196, 108886. https://doi.org/10.1016/j.apacoust.2022.108886
- Liu, H. K., Zhang, S. S., Liang, X. F., & Zou, Y. (2022). The effect of covering structure in pantograph sinking platform on the aerodynamics of high- speed train. Engineering Applications of Computational Fluid Mechanics, 16(1), 2157–2175. https://doi.org/10.1080/19942060.2022.2133517
- Liu, H. R., Wei, J. J., & Qu, Z. G. (2012). Prediction of aerodynamic noise reduction by using open-cell metal foam. Journal of Sound and Vibration, 331(7), 1483–1497. https://doi.org/10.1016/j.jsv.2011.11.016
- Liu, X. W., Hu, Z. W., Thompson, D. J., & Jurdic, V. (2018). Reduction of aerodynamic noise from square bars by introducing spanwise waviness. Journal of Sound and Vibration, 435, 323–349. https://doi.org/10.1016/j.jsv.2018.08.007
- Niu, J. Q., Zhou, D., Liang, X. F., & Jia, L. R. (2017). Influence of diversion device on unsteady aerodynamic performance of pantograph. Journal of ZheJiang University Engineering Science, 51, 752–760. https://doi.org/10.3785/jissn.1008-973X.2017.04.016
- Qin, D., Li, T., Zhang, J. Y., & Zhang, W. H. (2020). Effects of train pantograph operating height on aerodynamic performance. Scientia Sinica Technologica, 50(3), 335–345. https://doi.org/10.1360/SST-2019-0183
- Qin, D., Li, T., Zhou, P., & Zhang, J. Y. (2023). Effect of non-fully enclosed windshield on aerodynamic and acoustic behaviors of high-speed train. Environmental Science and Pollution Research, 30(25), 67804–67819. https://doi.org/10.1007/s11356-023-27296-4
- Shi, L., Zhang, C. C., Wang, J., & Ren, L. Q. (2012). Numerical simulation of the effect of bionic serrated structures on the aerodynamic noise of a circular cylinder. Journal of Bionic Engineering, 9(1), 91–98. https://doi.org/10.1016/S1672-6529(11)60101-7
- Shur, M. L., Spalart, P. R., Strelets, M. K., & Travin, A. K. (2008). A hybrid RANS-LES approach with delayed-DES and wall-modelled LES capabilities. International Journal of Heat and Fluid Flow, 29(6), 1638–1649. https://doi.org/10.1016/j.ijheatfluidflow.2008.07.001
- Spalart, P. R., Belyaev, K. V., Shur, M. L., Strelets, M. K., & Travin, A. K. (2019). On the differences in noise predictions based on solid and permeable surface ffowcs williams–hawkings integral solutions. International Journal of Aeroacoustics, 18(6-7), 621–646. https://doi.org/10.1177/1475472X19878934
- Sun, Z. K., Wang, T. T., & Wu, F. (2020). Numerical investigation of influence of pantograph parameters and train length on aerodynamic drag of high-speed train. Journal of Central South University, 27(4), 1334–1350. https://doi.org/10.1007/s11771-020-4370-6
- Sun, Z. X., Guo, D. L., Yao, S. B., Yang, G. W., & Li, M. G. (2013). Identification and suppression of noise sources around high speed trains. Engineering Applications of Computational Fluid Mechanics, 7(1), 131–143. https://doi.org/10.1080/19942060.2013.11015459
- Szepessy, S. (1994). On the spanwise correlation of vortex shedding from a circular cylinder at high subcritical reynolds number. Physics of Fluids, 6(7), 2406–2416. https://doi.org/10.1063/1.868438
- Tan, X. M., Xie, P. P., Yang, Z. G., & Gao, J. Y. (2019). Adaptability of turbulence models for pantograph aerodynamic noise simulation. Shock and Vibration, 1–20. https://doi.org/10.1155/2019/6405809
- Tan, X. M., Yang, Z. G., Tan, X. M., Wu, X. L., & Zhang, J. (2018). Vortex structures and aeroacoustic performance of the flow field of the pantograph. Journal of Sound and Vibration, 432, 17–32. https://doi.org/10.1016/j.jsv.2018.06.025
- Thompson, D. J., Iglesias, E. L., Liu, X. W., Zhu, J. Y., & Hu, Z. W. (2015). Recent developments in the prediction and control of aerodynamic noise from high-speed trains. International Journal of Rail Transportation, 3(3), 119–150. https://doi.org/10.1080/23248378.2015.1052996
- Wu, J. M. (2017). Numerical computation and improvement of aerodynamic radiation noises of pantographs. Journal of Vibroengineering, 19(5), 3939–3952. https://doi.org/10.21595/jve.2017.18300
- Wu, Z., Xie, Z., Wang, P., & Ding, W. (2020). Aerodynamic drag performance analysis of different types of high-speed train pantograph fairing. Journal of Applied Science and Engineering, 23, 509–519. https://doi.org/10.6180/jase.202009_23(3).0015
- Xiao, C. H., Yang, M., Tan, C., & Lu, Z. (2020). Effects of platform sinking height on the unsteady aerodynamic performance of high-speed train pantograph. Journal of Wind Engineering and Industrial Aerodynamics, 204, 104284. https://doi.org/10.1016/j.jweia.2020.104284
- Yao, S. B., Guo, D. L., & Yang, G. W. (2015). A modified multi-objective sorting particle swarm optimization and its application to the design of the nose shape of a high-speed train. Engineering Applications of Computational Fluid Mechanics, 9(1), 513–527. https://doi.org/10.1080/19942060.2015.1061557
- Yao, Z., Zhang, N., Chen, X., Zhang, C., Xia, H., & Li, X. (2020). The effect of moving train on the aerodynamic performances of train-bridge system with a crosswind. Engineering Applications of Computational Fluid Mechanics, 14(1), 222–235. https://doi.org/10.1080/19942060.2019.1704886
- Yu, H. H., Li, J. C., & Zhang, H. Q. (2013). On aerodynamic noises radiated by the pantograph system of high-speed trains. Acta Mechanica Sinica, 29(3), 399–410. https://doi.org/10.1007/s10409-013-0028-z
- Yuan, X. P., Miao, X. D., Yuan, T. C., & Yang, J. (2021). Aerodynamic noise analysis of high-speed train pantograph and study on noise reduction of pantograph head. Journal of the China Railway Society, 43(12), 38–48. https://doi.org/10.3969/j.issn.1001-8360.2021.12.005
- Zhang, P. K., Liu, Y., Li, Z. Y., Liu, H. R., & Yang, Y. N. (2020). Numerical study on reducing aerodynamic drag and noise of circular cylinders with non-uniform porous coatings. Aerospace Science and Technology, 107, 106308. https://doi.org/10.1016/j.ast.2020.106308
- Zhang, Y. D., Zhang, J. Y., Li, T., & Zhang, L. (2017). Investigation of the aeroacoustic behavior and aerodynamic noise of a high-speed train pantograph. Science China Technological Sciences, 60(4), 561–575. https://doi.org/10.1007/s11431-016-0649-6
- Zhang, Y. D., Zhang, J. Y., Li, T., Zhang, L., & Zhang, W. H. (2016). Research on aerodynamic noise reduction for high-speed trains. Shock and Vibration, 1. https://doi.org/10.1155/2016/6031893