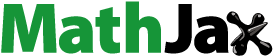
Abstract
The pantograph is one of the primary sources of aerodynamic drag and noise during the operation of a high-speed train. To investigate the impact of the pantograph fairing on the aerodynamic performance at various train heights, six computational models are established, and the Improved Delayed Detached Eddy Simulation method is employed. The results indicate that the installation of the fairing can effectively reduce the aerodynamic drag coefficient of the high-speed train and pantograph at different train heights. As the train height increases, the drag reduction effect of the fairing enhances when the sinking platform coexists. The overall drag reduction rate of up to 4.30% can be achieved at a train height of 4000 mm. However, the larger reduction rate of 58.32% in the pantograph's drag coefficient at a train height of 3700 mm indicates that the fairing could make a greater effect on the pantograph at a smaller train height. The reduction in train height and pantograph sinking height leads to an increase in drag coefficient on the tail car, and the implementation of the fairing further amplifies the drag. This work offers a reference for optimizing the aerodynamic performance of high-speed train.
1. Introduction
The aerodynamic effect of trains is becoming increasingly apparent as the operating speeds rise. The sharp increase in aerodynamic drag has become one of the main factors restricting further speed improvements and hindering energy saving. Reducing the aerodynamic drag of the train contributes to achieving high efficiency and low energy consumption for high-speed trains, thereby reducing operating costs and ensuring the potential for further increases in speed. Studies have shown that the drag generated by the air on the high-speed trains (HSTs) is proportional to the square of its velocity (Tian, Citation2009). With a rise in operating speed from 350 km/h to 400 km/h, the total train drag increases by 28.8%, with the proportion of aerodynamic drag increasing significantly to 92% (Wang et al., Citation2020). Additionally, the high-speed operation of trains can lead to fluctuations in aerodynamic forces, posing potential risks to the stable operation of trains under crosswind conditions. Further improvement of aerodynamic performance is a great challenge for high-speed trains. Generally, the main body structure of a train is crucial to its aerodynamic characteristics, and accessory components can also have notable impacts. At present, numerous scholars have conducted extensive and in-depth research on the train body and surrounding infrastructure to enhance the aerodynamic performance of HSTs. Existing optimizations encompass actions such as altering the shape of the head and tail sections of the train (Chen et al., Citation2018; Li et al., Citation2020; T. Li et al., Citation2019a; Tan & Yang, Citation2022; Wang et al., Citation2021), modifying the structure of the bogies and pantograph regions (H. Liu, S. Zhang, et al., Citation2022; W. Liu et al., Citation2022; Yao et al., Citation2022), designing the barriers around the train(Mohebbi & Rezvani, Citation2018a, Citation2018b; Mohebbi & Safaee, Citation2022), and optimizing the outer windshield (Niu et al., Citation2019). Especially, the pantograph installed on the top of the train is taken as one of the important sources of drag and noise of HSTs. Its drag constitutes about 12% of the total resistance (Yao et al., Citation2012), and thus its design for reducing drag and noise deserves further work for higher-speed trains.
Existing work on pantograph systems to reduce drag and noise is abundant and has been widely applied. The drag reduction achieved through the optimization of the pan head and rod components is notably remarkable. Lee et al. (Citation2015) found that optimizing the shape of the pan head and rod type could enhance the aerodynamic characteristics of the pantograph. Guo et al. (Citation2022) effectively reduced the radiated noise by modifying the upper and lower arms and the bow head. Ikeda & Mitsumoji (Citation2009) adopted the CFD technique to investigate the vortex and noise source structures in the vicinity of the pan head and articulated frame, and concluded that the combination of an optimized pan head with improved pan head support could efficiently lower the pantograph's aerodynamic noise. Nevertheless, the optimization space is also constrained when taking into account current collection.
The sinking platform is also an effective approach to reduce drag and has been used in HSTs such as CR and CRH. It can directly minify the windward area at the bottom of the pantograph to reduce itself resistances (Kim et al., Citation2020). Some scholars have conducted researches on roof smoothing, and analysed the impact of pantograph sinking on the aerodynamic characteristics of HSTs and pantographs (Kong & Yang, Citation2017). It is found that increasing sinking height leads to a reduction in the pantograph's drag and lift coefficients (Xiao et al., Citation2020). However, the sinking platform inherently brings about inevitable drag and cavity flow effects, and the depth of the sinking platform is constrained when the train height is insufficient. Under such circumstances, the adoption of streamlined fairing design has progressively emerged as a viable strategy for drag reduction and noise mitigation, especially when the train height is insufficient, while reducing noise emissions also helps to reduce passenger discomfort and reduce noise pollution in the surrounding environment.
According to research, covering structures or different forms of fairing have various impacts on the drag of HSTs (H. Liu et al., Citation2022; Wu et al., Citation2020). Compared with installing the pantograph directly on the roof, adding a streamlined fairing can significantly reduce the pantograph's drag and noise (H. Liu et al., Citation2022; Niu et al., Citation2017). A suitable fairing height can reduce the overturning moment and side deflection moment of the pantograph (Zhao et al., Citation2021). In terms of structural design and manufacturing feasibility, the design and production of the fairing are engineering achievable. Although the fairing itself might lead to an increase in structural weight, this issue can be alleviated by utilizing lightweight materials. Additionally, there will be associated costs in terms of maintenance and upkeep. Enhancing the aerodynamic characteristics of the pantograph can be accomplished by optimizing the fairing's design and the installation position of the pantograph (Sun et al., Citation2020; Zhang et al., Citation2017). At present, the research and application of this method are relatively limited, and they are all conducted based on the same train height. Further in-depth investigation is still required for the drag reduction optimization of fairings.
Taking an overall perspective, the current methods for drag and noise reduction in pantograph systems exhibit their respective advantages and limitations. To further enhance the effectiveness of drag reduction, it is necessary to fully integrate the respective advantages of the sinking platform and the fairing, especially considering different train heights. However, the research on the drag and noise reduction mechanisms of the coupling of the sinking platform and the fairing remains unclear at present. Therefore, to investigate the impact of pantograph fairing on the running resistance of trains at different train heights and pantograph settling heights, as well as to enhance the aerodynamic characteristics of HSTs, it is necessary to undertake corresponding research.
This work adopts numerical simulations to investigate the unsteady flow field in the pantograph region. The fairing is added in the pantograph region and the coverage height at the bottom of the pantograph is made consistent for different train heights. Subsequently, the effect of the fairing on the comprehensive aerodynamic characteristics of HSTs is investigated at different train heights and pantograph sinking heights, which can serve as a reference for research to reduce resistance.
2. Numerical simulation
2.1. Computational models and cases
The computational model, consisting of the head, middle, and tail cars, is constructed based on a scale of 1/8th of the CRH380A. The train retains detailed structures such as carriage joints and bogies while some subtle components such as handrails on the train's surface are omitted, to balance computational resources and the accuracy of the simulation. The pantograph is kept in a raised state and mounted on the rear of the middle roof when the train is in operation. The pantograph is comprised of the pan head, upper frame, lower frame, and base frame, and is simplified as closely as possible to the actual structure, as illustrated in Figure . To explore the effect of pantograph fairing on train resistance, six cases involving various train heights and pantograph settling heights are selected. The detailed settings are shown in Figure and Table , including the height of the train and the sinking basin. In the full-scale scenario, the distance from the bottom of the train's wheels to the top of the pantograph remains constant, with a height of 5.57 m, and the pantograph height is 1.87 m. The characteristic length H is defined as the distance between the bottom of the wheel and the top of the train, and its value is 0.5 m for a 1/8th scaled model. The 3-cars train's length is 19.5H.
Table 1. Calculation cases (full-scale).
2.2. Computational domain and boundary conditions
In compliance with the European standard (BS EN 14067), it is essential to configure the calculation domain in a manner that the flow field can reach a state of complete development during train operation. The length of upstream and downstream flow fields should be no less than eight and sixteen times the characteristic length H, respectively (Goldstein, Citation1974). The computational domain for all the cases is presented in Figure . The velocity inlet is situated at a distance of 18H from the train nose, and its speed is set at 111.11 m/s. The outlet with its gauge pressure of 0 Pa is positioned at a place of 37.5H from the rear of the train. The computational domain width and height are 16H and 10H, respectively. The symmetric boundary conditions are utilized for both the side and upper faces. The surface of HST is set as no-slip wall boundary condition and the ground is defined as moving wall, which adopts the inlet velocity to consider the relative motion of train to ground. The train is placed at the middle of the transverse direction, and its wheel bottom is 0.2 m from the bottom of the domain.
2.3. Grid generation
In the present work, the commercial software STAR-CCM + is employed for simulating turbulent flow around HSTs. This software has found extensive application in aerodynamic researches (Dong et al., Citation2020; X. Li et al., Citation2019). The meshing strategy is identical for all the models. An unstructured trimmed mesh with five levels of refinement is used to discretize the calculation region to guarantee the accuracy of the simulation. The ratio of grid size between the two encrypted areas is double to ensure a reasonable transition of the grid. Considering the importance of the boundary layer, 18 and 14 prism layers are set near the train and pantograph surfaces, respectively. The grid refinement is applied to the tail wake and pantograph region to achieve a more precise capture of the flow field around the train. The minimum surface grid size in the pantograph area is 3.75 × 10−4 m. The average y + is approximately 1. The grid of the calculation model is shown in Figure .
2.4. Numerical method
The Improved Delayed Detached-Eddy Simulation (IDDES) method is an improvement of DDES, incorporating DDES and Wall-Modeled Large Eddy Simulation methods, which can avoid the issue of Logarithmic-Layer Mismatch (LLM) and effectively save computational effort. By employing the IDDES method with the SST k-ω model, the present work simulates the flow field in the vicinity of the train. This turbulence model has been widely applied in engineering applications for train aerodynamics (X. Li et al., Citation2019; Luo et al., Citation2023; Xiao et al., Citation2020). The study employs a segregated flow solver based on the three-dimensional Navier-Stokes equations and utilizes the SIMPLE algorithm for the pressure-velocity coupling process. The convective term is discretized based on a hybrid scheme switching between a bounded central-differencing scheme and a second-order upwind scheme. A physical time step of 5 × 10−5 s is chosen for the simulation, and eight steps are iterated within each time step to provide temporal precision and numerical stability. In order to guarantee that the airflow reaches a satisfactory development situation, the overall simulation period is 0.9 s, and the aerodynamic drag is calculated by taking the average value between 0.45 and 0.9 s of flow field stability.
2.5. Numerical validation
The model of CRH2 is employed for numerical method validation due to its relatively extensive and accepted experimental data. The numerical results obtained using the IDDES method are compared with the test data provided by Zhang et al. (Citation2018). Identical boundary conditions are applied in both the numerical calculation and the wind tunnel test, and the airflow speed at the inlet is 60 m/s. Both numerical simulations and wind tunnel tests are conducted using a 1/8th scale train model. To facilitate analysis, the aerodynamic forces are dimensionless and defined as follows:
(1)
(1)
(2)
(2)
(3)
(3) where Cd, Cl, and Cp represent the aerodynamic drag, lift, and pressure coefficients, respectively. Fd and Fl are aerodynamic drag and lift. ρ is the reference density, u is the reference velocity, and S is the reference area. P and P0 are static and reference pressure.
To validate the grid-independence, three types of grids are established, including coarse, medium and fine grids. The grids contain 25, 40, and 59 million cells, respectively. Table displays the computed results. The numerical results show that the total drag coefficients agree well with the experimental data. Specifically, the differences for both fine and medium grids are even less than 1%, signifying that the present predictions are credible. It should be noted that the calculation errors for the head car are relatively large, which could partially be the differences in train geometry and the relatively complex airflow. Similar results can be found in previous studies (Guo et al., Citation2020). In addition, the nearly ignorable discrepancy between the fine and medium grids indicates that the latter is adequate to correctly predict the flow. Finally, Figure presents the Cp along the upper centreline of the train surface calculated by the medium grid. Good agreement can be observed with the wind tunnel data. In short, the good consistency with experimental result and the grid-independence study concludes that the current IDDES method and grid strategy are appropriate for analysing the aerodynamic performance of trains.
Figure 5. Comparison of Cp distribution along the upper centreline of the train surface between wind tunnel test and numerical result.

Table 2. Comparison of drag coefficient of CFD and wind tunnel tests.
3. Results and discussion
This section mainly presents the influence of pantograph fairing on aerodynamic performance at various train heights. Aerodynamic forces and flow field characteristics are compared and analysed for the train, with a focus on the pantograph area.
3.1. Aerodynamic forces
Table shows the Cd and Cl for various parts of the train, where the pantograph refers to the entire pantograph unit including the pan head, upper frame, lower frame, and base frame. Trains of various heights fitted with fairing structures experience lower aerodynamic drag coefficients than those without. The fairing demonstrates its maximum efficacy in diminishing net drag at a train height of 4000 mm, achieving a drag reduction rate of up to 4.30%. For trains with heights of 3850 and 3700 mm, the total drag coefficient can be lowered by 2.22% and 0.73%, respectively. With the inclusion of a streamlined fairing and a pantograph sinking depth of 150 mm, the train with a height of 3850 mm showcases the most optimal overall Cd.
Table 3. Force coefficients for different parts of the train.
The pantograph, positioned at the tail of the middle car, exerts a relatively lesser influence on the drag coefficient of the head car. With the decrease in train height, there is a corresponding reduction in the force surface of the entire train, resulting in a gradual decrease in the aerodynamic drag coefficient of the head car. The setting of the fairing has a considerable effect on the flow field near the pantograph. Air flow disturbs the pantograph region and continues to propagate backward, affecting the flow field distribution around the tail car. This explains the substantial change in the aerodynamic drag coefficient of the tail car. The Cl of the train is primarily affected by the different pressure distribution across a train's upper and lower surfaces. Excessive negative lift can result in an elevation of the train's dynamic axis weight, accentuating the dynamic impact. The aerodynamic lift of the whole train is negative in the six cases calculated. With the addition of the fairing, the absolute value of the negative lift is reduced for trains with heights of 4000 and 3850 mm, which can reduce the dynamic impact to a certain extent. After installing the fairing, the Cl of the pantograph increase, enhancing the contact force with the catenary. In the presence of the fairing, middle car's Cl grows as the train height declines and the fairing height increases, while the tail car's Cl lowers.
The information in Table shows that setting the fairing has an effect on Cd and Cl in the pantograph region. Table presents the aerodynamic coefficients for pantograph, sinking platform, and fairing, and Figure displays the variation in Cd and Cl for each pantograph component as a bar graph, providing a more detailed study of the pantograph area. Installing a streamlined fairing can markedly lower the drag coefficient of the covered components. By employing fairings, the pantograph's drag coefficient can be substantially reduced by 42.43%, 54.83%, and 58.32% at train heights of 4000, 3850, and 3700 mm, respectively. With the increase in fairing height, the aerodynamic drag coefficients of the pan head, upper and lower frame gradually amplify. This phenomenon arises from the rising height of the fairing, causing air to flow to the upper section of the pantograph after striking the front side of the fairing, thereby impacting the lower frame and upper portions to a greater extent. Comparing the three models of A2, B2 and C2 with the fairing installed, the immediate influence area of the airflow on the fairing enlarges as the fairing's height increases, leading to a significant grow in the fairing's drag. Additionally, the fairing device also exerts a pivotal function in reducing the drag of the platform surface. The Cl of the upper and lower frame exhibit contrary directions owing to their distinct spatial orientations.
Figure 6. Comparison of aerodynamic forces: (a) Cd of pantograph components; (b) Cl of pantograph components.

Table 4. Force coefficients of pantograph, sinking platform and fairing.
In Figure , the time-dependent partial curves are presented to analyse the effect of the fairing on the pan head and pantograph for different train heights. The setting of the fairing increases the Cd of the pan head at different train heights. This is mainly due to the airflow continues to flow upwards after hitting the fairing, creating a disturbance to the flow field near the pan head. The Cl of the pan head varies less in different cases. On the other hand, the installation of the fairing leads to a substantial decrease in the total Cd of the pantograph, accompanied by an increase in the Cl. This can be attributed to the base frame is fully submerged in the low-speed airflow generated by the fairing, while the sections above the base frame are affected by the airflow lifted by the fairing.
Figure 7. Aerodynamic coefficients of the pan head and pantograph: (a) Cd of pan head, (b) Cd of pantograph, (c) Cl of pan head, (d) Cl of pantograph.

To acquire a more comprehensive understanding of the response frequency discrepancy of the pantograph when subjected to aerodynamic effects, Fourier transform is implemented. Fourier transform can be employed to identify any dominant frequencies and their associated amplitudes, providing valuable insights into the aerodynamic effects on the pantograph. The power spectral densities of the pan head, upper frame and lower frame are displayed in Figure . According to the characteristic height and air velocity, the frequency can be converted to the corresponding Strouhal number (St). Results show that the power spectral densities of the pan head, upper and lower frame exhibit significant variations at St >10 when the fairing is installed. The installation of the fairing causes the energy of the pan head to converge at different train heights. For the train height of 4000 mm without a fairing, small peaks in energy are observed at St = 4.88, 7.91, and 14.29 for the pan head. At St = 14.29, the energy of the pan head exhibits disparities among various train heights in the absence of the fairing, whereas it becomes similar when the fairing is introduced. Furthermore, the configuration of the fairing leads to a reduction in energy of the lower frame.
Figure 8. Power spectral density plot: (a) pan head without fairing, (b) pan head with fairing, (c) upper frame without fairing, (d) upper frame with fairing, (e) lower frame without fairing, (f) lower frame with fairing.

The aerodynamic drag coefficients with and without fairings are shown in Figure for the three train heights. Upon comparison of the cases without a fairing, it is evident that there is a reduction in both the drag coefficient and it's changing amplitude as the train height decreases. The fairing further reduces the train drag coefficient, and the highest net drag reduction rate occurs at a train height of 4000 mm. It deserves attention that the net drag reduction rate of the train diminishes with the decrease in train height and the increase in fairing height. This can be attributed to the drag-lowering effect caused by the decrease in train height gradually weakens, while the drag-increase effect caused by the growth in fairing height gradually becomes more prominent.
Table and Figure present the changes in Cd and Cl of various train components for different cases. By employing a fairing, a substantial reduction in the pantograph's Cd and an elevation in its Cl are observed. The decrease in train height results in a reduction of the Cd for the head car. The installation of the fairing has a more pronounced impact on the middle and tail cars. The airflow continues to propagate backward after passing through the pantograph area, causing some disturbance to the flow field in the vicinity of the tail car. With the reduction in train height, the tail car's drag intensifies, and the implementation of the fairing exacerbates the drag even further. The reduction in train height and the elevation of fairing height causes a rise of the Cl for the middle car, whereas the opposite trend is observed for the tail car.
Figure illustrates the distribution of Cp along the upper centreline of the train surface. The various train heights cause a significant difference in the curve when it is located at the streamlined rear of the head and tail cars. It is evident that negative pressure occurs in the streamlined portion of the tail car. As the train height decreases, the absolute value of the negative pressure increases, which potentially constitutes factor leading to the escalation of drag on the tail car. In the gap regions of the carriage, the airflow experiences flow separation owing to the discontinuous surface, which can have a considerable impact on the aerodynamic characteristics of the train. The minimum value of Cp appears at the trailing edge of the platform in A1, and the negative peak disappears after setting the fairing. Setting the fairing and changing the depth of the pantograph sinking directly affects the Cp of the top of the train in the pantograph zone. In the presence of fairing, the pressure variation in the front half of region R1 is similar, rising first and falling later. The airflow hits the fairing and results in an increase in the surrounding pressure. Afterwards, the disturbance of airflow inside the cavity reduces due to the covering effect of the fairing, leading to a decrease in pressure. Subsequently, the airflow hits the inner end of the fairing and the pressure on the train surface continues to increase again. The expansion of the space inside the fairing also results in a drop in the train's surface pressure.
Figure 11. Comparisons of Cp distribution (Without pantograph and fairing): (a) along the upper centreline of the train surface, (b) local magnification of region R1.

From Figure , it can be observed that in the absence of the fairing, there exists negative pressure at the rear edge of the sinking platform on the middle train. Influenced by the lifting effect of the trailing edge of the sinking platform on the airflow and the gap at the car connection, a negative pressure region forms at the front end of the tail car, which gradually decreases in absolute value with increasing distance. After adding the fairing, the accumulation of airflow on the inner side of the fairing's rear end induces a positive pressure at the rear edge of the sinking platform. The interference from the fairing's rear end with the airflow leads to a positive pressure at the front end of the tail car, with the pressure diminishing as the distance increases. As the fairing's bulge height increases, the positive pressure at the front end of the fairing rise.
3.2. Flow structures
To compare the aerodynamic characteristics of different models, the Cp distribution in the pantograph area and carriage joint region of the tail car is illustrated in Figures and . The aerodynamic drag of HSTs is basically resulting from the difference in pressure. As can be seen in Figures and , there is a significant variation in the distribution of Cp. Figure illustrates that the rear surface and the edge of the sinking platform in A1 have large areas of positive and negative pressure, respectively. Installing a fairing effectively reduces the positive and negative high-pressure regions inside the sinking platform, thereby lowering the drag. In addition, the setting of the fairing also effectively decreases the positive and negative high-pressure regions of the base frame, which is the vital reason of the variation in the Cd in Table . As the fairing height increases, larger areas of positive and negative pressure appear at the front and top of the fairing, respectively. Based on the information presented in Figure , in the absence of the fairing cover, the upper edge of the tail car connection exhibits a negative pressure region when the train height is 4000 mm, while the remaining edges manifest as positive high-pressure zones. With the reduction in train height and the elevation of the fairing height, the positive pressure zone on the upper edge of the tail car connection grows significantly. This indicates that the setting of the fairing and its height modification affect the airflow at the tail car connection and alter the tail car's drag.
As can be seen from Figures and , with the decrease of train height and pantograph sinking height (i.e. A1-B1-C1), the pressure on the surface at the carriage connection of the tail car gradually increases. This is due to the lack of a windshield at the carriage connection, which makes the airflow impact on the front surface of the tail car to a certain extent, consequently leading to an increase in drag on the tail car. Upon the installation of the fairing, due to the disruption caused by the rear end of the fairing to the airflow, the airflow does not lift upward at the trailing edge of the sinking platform as in A1, and more airflow converges at the carriage connection between the middle and tail car. This intensifies the impact on the tail car's surface, thereby increasing the drag on the tail car. Therefore, it is necessary to adopt an appropriate windshield structure to reduce the aerodynamic drag on the tail car.
Figure displays the mean vorticity of the middle symmetrical plane for the six cases. In the absence of the fairing, the region surrounding the pantograph and platform exhibits significant vorticity, with the wake developing upward at the junction of the upper and lower frames. The installation of the fairing results in a decrease in vorticity near the base frame and lower regions. The direction of the wake flow at the articulation of the upper and lower frames changes, and the vorticity at this location becomes stronger. Furthermore, the presence of the fairing disrupts the flow field in the vicinity of the pantograph, while the wake generated by the fairing and pantograph affects the distribution of vortices at the connection of the train body. It makes the vortex at the connection between the middle and tail cars become larger. As the wake generated in front of the fairing converges with the upper section of the pantograph, the flow field becomes more intricate, resulting in an augmentation of vorticity around the lower frame. Moreover, the vortex on the back side of the fairing intensifies as the fairing height increases.
Figure shows the velocity contours and streamline distribution in the pantograph region on the middle symmetrical plane. The high-speed airflow is predominantly distributed around the pan head, within the clamping angle of the upper and lower frames and their articulation. The airflow experiences a decrease in velocity after passing through the pantograph. When the depth of sinking platform is large, notable flow separation can be found at the front end of the platform, resulting in the formation of a low-speed zone. Widening of the distribution range of the low-velocity flow field can be observed after the fairing is installed, and the effect of airflow disturbance on the base frame is weakened. The airflow velocity above the fairing increases. Additionally, the recirculation is predominantly located in the wake region behind the base frame and carriage joint region of the tail car. With the reduction of train height and the increase of fairing height, the front flow field inside the fairing becomes smoother.
Figure shows the iso-surface of Q around the pantograph rendered by the time-average velocity, and Q can be written as:
(4)
(4) where
and
are the symmetric and anti-symmetric parts of the velocity gradient tensor, respectively.
During train operation, the airflow generates a number of vortex structures behind the pantograph which continue to develop, fall off, and rupture towards the rear of the train. Large-scale vortices primarily distributed around the base frame and behind the pantograph. The pan head, base frame, articulation of the upper and lower frame are the main locations for the high-speed vortex to be found. The placement of the fairing reduces the vortex velocity close to the base frame, but it interferes with the nearby air distribution. The installation of the fairing causes the airflow to converge within the gap at the front end of the tail car, leading to an increase in pressure at the carriage joint and accordingly an augment of aerodynamic drag for the tail car. The number of high-speed large-scale vortices around the pantograph increases as the fairing height rises. A series of small-scale vortices created at the front of the fairing shield merges with the large-scale vortices created by the base frame and continues to flow towards the posterior region of the train.
4. Conclusions
In this paper, the IDDES method is applied for simulating the flow field structure in the vicinity of the HSTs. Studies are conducted on the impact of pantograph fairing on the aerodynamic properties at various train heights and pantograph settling heights. According to the results of the present work, the primary conclusions are as follows:
The installation of the fairing contributes to drag reduction for trains at various train heights. The fairings are more effective in reducing drag with increasing train height when combined with the sinking platform. By employing fairings, the pantograph's drag coefficient can be substantially reduced by 42.43%, 54.83%, and 58.32% at train heights of 4000, 3850, and 3700 mm, respectively. Meanwhile, a drag reduction rate of 4.30% can be achieved by the train with a height of 4000 mm.
The lift coefficient of the pantograph experiences an elevation by mounting the fairing, while the energy of the pan head reaches a consistent level under different train heights. The positive and negative pressure regions of the covered components are greatly reduced, such as the base frame and sinking platform. The vorticity near the base frame exhibits a reduction, whereas vorticity intensifies around the lower frame. Moreover, the quantity of high-speed large-scale vortices surrounding the pantograph increases with the elevation of the fairing height.
The flow field near the tail car is affected significantly, as the airflow propagates rearward after perturbing the pantograph region. The tail car experiences an amplified drag with the reduction in both train height and pantograph sinking height, which is further exacerbated by the implementation of the fairing. The growth of the fairing height brings about a notable expansion of the positive pressure zone along the upper edge of the tail car connection. Additionally, the middle car exhibits an increase in lift, whereas the opposite effect occurs for the rear car.
The shape parameter of the pantograph fairing also affects its aerodynamic performance. This study exclusively investigated the aerodynamic effects of a specific form of the fairing while disregarding its noise performance. It is suggested that both the aerodynamic drag and noise performance of the pantograph fairing should be considered comprehensively in the subsequent research, and the design parameters, performance under crosswind operating conditions and the combined effects of the fairing with other aerodynamic measures should be explored.
Acknowledgments
The authors acknowledge the computing resources provided by the High Performance Computing Public Platform of Central South University, China.
Disclosure statement
No potential conflict of interest was reported by the author(s).
Additional information
Funding
References
- Chen, Z., Liu, T., Jiang, Z., Guo, Z., & Zhang, J. (2018). Comparative analysis of the effect of different nose lengths on train aerodynamic performance under crosswind. Journal of Fluids and Structures, 78, 69–85. https://doi.org/10.1016/j.jfluidstructs.2017.12.016
- Dong, T., Minelli, G., Wang, J., Liang, X., & Krajnović, S. (2020). The effect of ground clearance on the aerodynamics of a generic high-speed train. Journal of Fluids and Structures, 95, 102990. https://doi.org/10.1016/j.jfluidstructs.2020.102990
- Goldstein, M. (1974). Unified approach to aerodynamic sound generation in the presence of solid boundaries. The Journal of the Acoustical Society of America, 56(2), 497–509. https://doi.org/10.1121/1.1903283
- Guo, J., Tan, X., Yang, Z., Xue, Y., Shen, Y., & Wang, H. (2022). Aeroacoustic optimization design of the middle and upper part of pantograph. Applied Sciences-Basel, 12(17), 8704. https://doi.org/10.3390/app12178704
- Guo, Z., Liu, T., Hemida, H., Chen, Z., & Liu, H. (2020). Numerical simulation of the aerodynamic characteristics of double unit train. Engineering Applications of Computational Fluid Mechanics, 14(1), 910–922. https://doi.org/10.1080/19942060.2020.1784798
- Ikeda, M., & Mitsumoji, T. (2009). Numerical estimation of aerodynamic interference between panhead and articulated frame. Quarterly Report of RTRI, 50(4), 227–232. https://doi.org/10.2219/rtriqr.50.227
- Kim, H., Hu, Z., & Thompson, D. (2020). Effect of cavity flow control on high-speed train pantograph and roof aerodynamic noise. Railway Engineering Science, 28(1), 54–74. https://doi.org/10.1007/s40534-020-00205-y
- Kong, X., & Yang, M. (2017). Influence of pantograph subsidence on high-speed train aerodynamic drag. Journal of Railway Science and Engineering, 14(09), 1805–1813. https://doi.org/10.19713/j.cnki.43-1423/u.2017.09.001
- Lee, Y., Rho, J., Kim, K. H., Lee, D. H., & Kwon, H. B. (2015). Experimental studies on the aerodynamic characteristics of a pantograph suitable for a high-speed train. Proceedings of the Institution of Mechanical Engineers Part F-Journal of Rail and Rapid Transit, 229(2), 136–149. https://doi.org/10.1177/0954409713507561
- Li, R., Xu, P., & Yao, S. (2020). Optimization of the high-speed train head using the radial basis function morphing method. Proceedings of the Institution of Mechanical Engineers, Part F: Journal of Rail and Rapid Transit, 234(1), 96–107. https://doi.org/10.1177/0954409719841518
- Li, T., Qin, D., Li, M., & Zhang, J. (2019a). Aerodynamic drag reduction of a high-speed train nose With bionic round pits. Computing in Science & Engineering, 21(3), 31–41. https://doi.org/10.1109/MCSE.2019.2902474
- Li, X., Chen, G., Wang, Z., Xiong, X., Liang, X., & Yin, J. (2019). Dynamic analysis of the flow fields around single- and double-unit trains. Journal of Wind Engineering and Industrial Aerodynamics, 188, 136–150. https://doi.org/10.1016/j.jweia.2019.02.015
- Liu, H., Zhang, S., Liang, X., & Zou, Y. (2022). The effect of covering structure in pantograph sinking platform on the aerodynamics of high- speed train. Engineering Applications of Computational Fluid Mechanics, 16(1), 2156–2174. https://doi.org/10.1080/19942060.2022.2133517
- Liu, H., Zhou, S., Chen, R., Li, Z., Zhang, S., & Zhao, Y. (2022). Numerical study on the aeroacoustic performance of different diversion strategies in the pantograph area of high-speed trains at 400 km/h. Applied Sciences-Basel, 12(21), https://doi.org/10.3390/app122110702
- Liu, W., Ji, Z., Guo, D., Yang, G., Zhou, G., & Ren, K. (2022). Effects of bottom deflectors on aerodynamic drag reduction of a high-speed train. Acta Mechanica Sinica, 38(5), 321251. https://doi.org/10.1007/s10409-021-09058-x
- Luo, J., Wang, L., Shang, S., Li, F., Guo, D., Gao, L., & Wang, D. (2023). Study of unsteady aerodynamic performance of a high-speed train entering a double-track tunnel under crosswind conditions. Journal of Fluids and Structures, 118, 103836. https://doi.org/10.1016/j.jfluidstructs.2023.103836
- Mohebbi, M., & Rezvani, M. A. (2018a). The impact of Air fences geometry on Air flow around an ICE3 high speed train on a double line railway track with exposure to crosswinds. Journal of Applied Fluid Mechanics, 11(3), 743–754. https://doi.org/10.18869/acadpub.jafm.73.246.27862
- Mohebbi, M., & Rezvani, M. A. (2018b). Multi objective optimization of aerodynamic design of high speed railway windbreaks using lattice boltzmann method and wind tunnel test results. International Journal of Rail Transportation, 6(3), 183–201. https://doi.org/10.1080/23248378.2018.1463873
- Mohebbi, M., & Safaee, A. M. (2022). The optimum model determination of porous barriers in high-speed tracks. Proceedings of the Institution of Mechanical Engineers Part F-Journal of Rail and Rapid Transit, 236(1), 15–25. https://doi.org/10.1177/0954409721995323
- Niu, J., Wang, Y., & Zhou, D. (2019). Effect of the outer windshield schemes on aerodynamic characteristics around the car-connecting parts and train aerodynamic performance. Mechanical Systems and Signal Processing, 130, 1–16. https://doi.org/10.1016/j.ymssp.2019.05.001
- Niu, J., Zhou, D., Liang, X., & Jia, L. (2017). Influence of diversion device on unsteady aerodynamic performance of pantograph. Journal of Zhejiang University(Engineering Science), 51(04), 752–760. https://doi.org/10.3785/j.issn.1008-973X.2017.04.016
- Sun, Z., Wang, T., & Wu, F. (2020). Numerical investigation of influence of pantograph parameters and train length on aerodynamic drag of high-speed train. Journal of Central South University, 27(4), 1334–1350. https://doi.org/10.1007/s11771-020-4370-6
- Tan, X., & Yang, Z. (2022). Investigation on aerodynamic noise reduction for snow-plough of high-speed train. Journal of Central South University, 29(5), 1735–1748. https://doi.org/10.1007/s11771-022-4922-z
- Tian, H. (2009). Formation mechanism of aerodynamic drag of high-speed train and some reduction measures. Journal of Central South University of Technology, 16(01), 166–171. https://doi.org/10.1007/s11771-009-0028-0
- Wang, L., Yu, D., & Ding, Y. (2020). Feasibility study on raising the operation speed of EMU from 350 km/h to 400 km/h. Electric Drive for Locomotives (02), 17-22. https://doi.org/10.13890/j.issn.1000-128x.2020.02.004
- Wang, S., Wang, R., Xia, Y., Sun, Z., You, L., & Zhang, J. (2021). Multi-objective aerodynamic optimization of high-speed train heads based on the PDE parametric modeling. Structural and Multidisciplinary Optimization, 64(3), 1285–1304. https://doi.org/10.1007/s00158-021-02916-0
- Wu, Z., Xie, Z., Wang, P., & Ding, W. (2020). Aerodynamic drag performance analysis of different types of high-speed train pantograph fairing. Journal of Applied Science and Engineering, 23(3), 509–519. https://doi.org/10.6180/jase.202009_23(3).0015
- Xiao, C., Yang, M., Tan, C., & Lu, Z. (2020). Effects of platform sinking height on the unsteady aerodynamic performance of high-speed train pantograph. Journal of Wind Engineering and Industrial Aerodynamics, 204, 104284, https://doi.org/10.1016/j.jweia.2020.104284
- Yao, S., Guo, D., Yang, G., & Li, M. (2012). Distribution of high-speed train aerodynamic drag. Journal of the China Railway Society, 34(07), 18–23. https://doi.org/10.3969/j.issn.1001-8360.2012.07.003
- Yao, Y., Sun, Z., Li, G., Yang, G., Prapamonthon, P., Guo, Y., & Wang, M. (2022). Aerodynamic optimization using passive control devices near the bogie cabin of high-speed trains. Acta Mechanica Sinica, 38(9), 321363. https://doi.org/10.1007/s10409-022-21363-x
- Zhang, L., Yang, M., & Liang, X. (2018). Experimental study on the effect of wind angles on pressure distribution of train streamlined zone and train aerodynamic forces. Journal of Wind Engineering and Industrial Aerodynamics, 174, 330–343. https://doi.org/10.1016/j.jweia.2018.01.024
- Zhang, L., Zhang, J., Li, T., & Zhang, W. (2017). Influence of pantograph fixing position on aerodynamic characteristics of high-speed trains. Journal of Modern Transportation, 25(1), 34–39. https://doi.org/10.1007/s40534-017-0125-y
- Zhao, M., Liu, M., Liu, X., Liu, Z., & Wang, Y. (2021). Influence of height of dome on aerodynamic characteristics of pantograph under cross wind. Mechanical Science and Technology for Aerospace Engineering, 40(11), 1710–1716. https://doi.org/10.13433/j.cnki.1003-8728.20200266